DOI:
10.1039/D2LC00796G
(Perspective)
Lab Chip, 2023,
23, 1349-1357
Will the next generation of chemical plants be in miniaturized flow reactors?
Received
29th August 2022
, Accepted 7th October 2022
First published on 12th October 2022
Abstract
For decades, a production paradigm based on centralized, stepwise, large scale processes has dominated the chemical industry horizon. While effective to meet an ever increasing demand for high value-added chemicals, the so-called macroscopic batch reactors are also associated with inherent weaknesses and threats; some of the most obvious ones were tragically illustrated over the past decades with major industrial disasters and impactful disruptions of advanced chemical supplies. The COVID pandemic has further emphasized that a change in paradigm was necessary to sustain chemical production with an increased safety, reliable supply chains and adaptable productivities. More than a decade of research and technology development has led to alternative and effective chemical processes relying on miniaturised flow reactors (a.k.a. micro and mesofluidic reactors). Such miniaturised reactors bear the potential to solve safety concerns and to improve the reliability of chemical supply chains. Will they initiate a new paradigm for a more localized, safe and reliable chemical production?
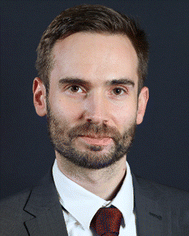 Jean-Christophe M. Monbaliu | Jean-Christophe M. Monbaliu is currently Associate Professor at the University of Liège (Belgium) and serves as Associate Editor of the Journal of Flow Chemistry. He is heading the Center for Integrated Technology and Organic Synthesis (CiTOS, https://www.citos.uliege.be), the first European Corning® Advanced-Flow™ reactor qualified lab. Research interests at CiTOS revolve around synthetic organic chemistry but are multidisciplinary in essence and aim at (a) designing cheaper and more efficient routes for the preparation of high value-added chemicals such as active pharmaceutical ingredients; (b) accelerating the transition from petrobased to biobased strategies and (c) developing efficient processes with a lower environmental impact. |
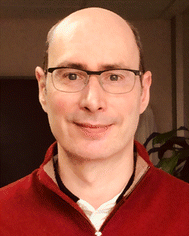 Julien Legros | Julien Legros is currently CNRS Research Director at the University of Rouen Normandy (France) and head of the CNRS French Network on Flow Chemistry (GDR Synth Flux). Group leader of the “MESOO” research team within the COBRA laboratory in Rouen (https://www.lab-cobra.fr), his research is oriented toward the use of alternative reactors, namely hyperbaric reactors and miniaturized continuous flow reactors for the detoxification of chemical warfare agents, the synthesis of active pharmaceutical ingredients and methodological development in organic and organometallic chemistry. He has been CNRS coordinator for the European France(Channel)England program INTERREG Turning Laboratories into Factories (LabFact) and Smart Textiles (SmartT). |
Introduction
Recent global events are leading the world to rethink some models which, until recently, seemed irreplaceable. The COVID pandemic has pulverised economic patterns of some manufactured goods, among which the severe limit in fine chemical supply, and therefore of active pharmaceutical ingredients (APIs), has been highlighted. Significant shortages of strategic medicines were thus cruelly felt, jeopardising the capacity of national health systems to treat their fellow citizens. Most of the so-called “industrialized nations” have shown the limitations of their industrial apparatus in this emergency situation. Moreover, the Russian–Ukrainian conflict has further compounded this situation with an urgent need for rapid and radical change in reorganizing manufacturing.
Over the last twenty years, the production of fine chemicals, such as APIs and their intermediates (most of which have fallen into the public domain), has been almost entirely relocated to remote countries for both reasons of cost and lower environmental/safety constraints, which is intrinsically linked to the production method for these compounds: macroscopic batch reactors. Indeed, the use of batch reactors means that, in order to produce more, their capacity must be increased, leading to high investments and significant workforces, as well as significant safety concerns. This logic has led to the dismantling of chemical production facilities in numerous countries.
Unlike other sciences that have revolutionised their concepts over the last few decades, organic synthesis (the centrepiece of drug manufacturing) has used roughly the same tools since the 1950s and is still based on knowledge that is often empirical. However, there is now an alternative technology to this synthesis in large reactors: miniaturised continuous flow reactors. Where conventional production requires very large installations, flow synthesis uses a production tool with the size of household appliances.1–9 This “miniature factory” is both modular and mobile; it could be used to prepare different molecules of interest at different sites according to local needs.
Chemical synthesis: thinking small to produce more… and better
As evoked above, the tools for producing fine chemicals have not been subjected to major changes and the transfer of a chemical reaction from lab-scale to production (scale-up) goes through the increase of reactor size from milliliters to several thousands of liters. The quantity of chemical produced is thus related to the capacity of the reactor (hence the name of “batch synthesis”). In contrast, miniaturized continuous-flow reactors consist of reacting chemicals (either liquid, gaseous or solid) in motion in tubes (or channels) of micrometric or millimetric diameter, without ever interrupting the reaction (at least theoretically, maintenance may impose periodic interruptions). The intrinsic features of this technology make it possible to solve many problems encountered in chemical synthesis. Fast mixing and efficient heat transfers are now well-established assets of flow reactors and often deployed for reactions sensitive to local stoichiometry,10 biphasic systems,11,12 strongly exothermic and high-temperature processes with sensitive materials.13 Flow chemistry also brings novel challenges to the Chemist and Chemical Engineer, such as for handling solids and high viscosity materials. Viscosity is commonly dealt through higher process temperatures or with appropriate additives. For solids, major advances were recently reported with oscillatory reactors for the handling of slurries/heterogeneous catalysts.14 Alternatively, the use of packed-bed reactors with immobilized heterogeneous regents or catalysts is very common. Marginal, yet effective solutions for handling solids may also rely on process temperatures above their melting points.15
Although counter-intuitive, miniaturised flow-through devices greatly accelerates and simplify the scale-up of a process since a flow-through reactor allows for a quantity of material proportional to its operating time, avoiding reactor re-sizing and inevitable redesign of reaction conditions.1,16 Note that the quantity of material involved at a given time remains the same regardless of scale, which is very valuable for reactions that are difficult to control on a large scale, or for the manipulation of toxic species (vide infra). In order to produce substantial amounts of compounds, some companies even commercialize flow devices with an increasing number of reactor plates and high flow rates (Scheme 1). Consequently, numbering-up or scaling-out strategies can be easily deployed for accessing larger scales in flow.7 Typical outputs for miniaturized reactors can drastically vary, hence also conditioning their end-use: microreactors with a typical internal diameters of ∼100 μm and an internal volume of a few 100 μL are usually associate with throughputs of a ∼100 μL min−1; mesofluidic reactors with an internal diameter of up to 800 μm are usually associated with total flow rates of about 10 mL min−1, and are well suited in lab environments. Larger with internal dimensions of about 1 mm can accommodate flow rates of several hundred mL min−1 (pilot scale) and up to several L min−1 (commercial scale). Accessing the scalability and selecting an appropriate strategy to reach larger scales in flow is often a critical point. The selection of a strategy, namely numbering-up or scaling-out (Scheme 1) ultimately depends on the end product, the target market and internal organization protocols. For instance, radiopharmaceuticals, the shelf-life of which is very limited, are often produced on the spot with microreactors of limited volumes being more than sufficient to produce the required dosages. Scaling up a process to access pharmaceuticals will already be very successful with a few kilograms per day of active ingredient. Other specialty or bulk chemicals often require much larger scales.
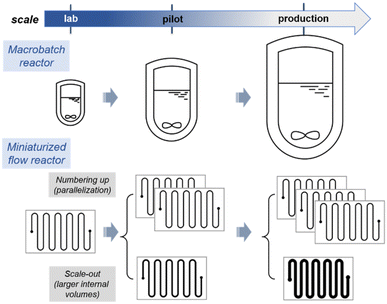 |
| Scheme 1 Chemical synthesis, from lab to production: batch vs. flow strategy. | |
One of the major breakthrough in flow-microreactors is the development of the so-called “flash chemistry” by Yoshida.17,18 The uniqueness of this approach relies on the control of very fast reactions, in virtue of the very short residence time tR permitted in the microreactor, allowing to perform “impossible chemistries” (i.e. in batch reactors).18 Indeed in a flow reactor, the reaction time is a direct function of the reactor size according to tR = (volume of the reactor)/(flow rate), and miniaturized flow reactors allow to attain very short reaction times: a few minutes to some milliseconds19–21 and even below.22 This breakthrough is however often connected to a hard-to-eradicate misconception, namely, that flow conditions only applies to reactions with inherent fast kinetics. This misconception relates to the small internal volume of flow reactors, which is often associated with short residence/reaction times. Flow microreactors offer several options for process intensification, among which the temperature and pressure are often leveraged to significantly accelerate slow reactions. It is quite common to find in the actual state of the art striking examples with reaction processes taking hours in batch while reaching completion within minutes or less under intensified flow conditions.13,23,24
Moreover, a key distinction between batch and flow conditions is the composition of the reaction medium. In a macroscopic batch reactor, the chemical composition of the reaction medium evolves with time as the conversion of reactants/substrates to products increases, hence labelling these processes as “time-resolved”. Under flow conditions, the conversion varies along the reaction path, thus increasing (and ideally reaching completion at the outlet of the flow reactor) and thus defining “space-resolved” conditions. An easy fix to this apparent dilemma, which might be one of the most confusing aspects of flow chemistry for neophytes, relies on the concept of residence time. The latter correlates space and time and offers a very handy metric to connect the volume of the reactor, the overall velocity of fluids going through it and the time required to leave the reactor. Regardless of the regime in a flow reactor (laminar, transitional or turbulent), any point in the reactor thus corresponds to a specific state of progress of the reaction (Scheme 2). This feature avoids undesired byproducts due to consecutive competitive reactions between reagents and products, with high benefits for chemical selectivity toward a desired single reaction product.25,26 The emergence of photochemistry in flow over the past decade has also tremendously benefited from this feature, with a much finer control on irradiation time even for large scale applications,27 and there a much reasons to believe that electrochemistry in flow is improved likewise.28–30
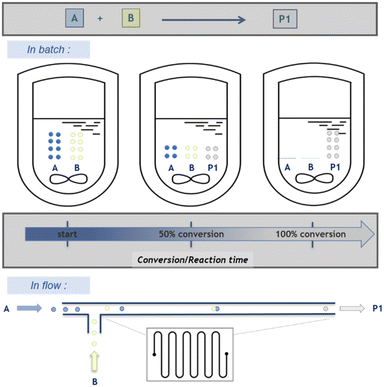 |
| Scheme 2 Evolution of the chemical composition of a reactor: batch (up) vs. flow (down). | |
This approach is especially fruitful for the ultra-fast halogen–lithium exchange where unstable chemical entities can be formed, previously untamed under classical conditions.21,31,32 The generation and trapping of such entities in an integrated system allow thus to extend the chemical space to new transformations and novel molecular architectures (Scheme 3).
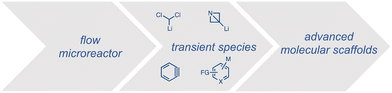 |
| Scheme 3 Flash chemistry in flow microreactors. | |
Noteworthy, such very fast reactions in very small reactors naturally induce high productivity (quantity of product/time) and high space–time yields (productivity/reactor volume), which are key points for cost-effective delivery of chemicals.
The development of processes under flow conditions in miniaturized reactors is often presented as a solution for reducing their environmental footprint and to increase their sustainability. While the arguments associated with the reduced needs for energy, improved homogeneity and purity of reactor effluents and increased inherent safety definitively speak for themselves, the simple transposition of batch conditions under flow does not guarantee de facto sustainability.33–36 The development of flow processes for replacing older batch processes must also be seen as an opportunity to redefine the chemistry in light of the assets of flow technology.37
Reactors downsized, safety increased
Performing chemical reactions at large scale in macroscopic batch reactors comes with safety concerns, which arise at different stages of the process. Safety issues are associated with stockpiling and shipment of large volumes of starting materials and intermediates with a high risk of accidental chemical spilling. Many industrial incidents are associated with thermal runaway in large vessels, with potentially dramatic impacts on the surrounding operators and environment. One obvious solution to significantly reduce chemical hazard upon processing chemicals relies on downsizing the internal size of the reactor – such strategy is not compatible with macroscopic batch reactors within the context of a centralized, global production scheme, since a reduction in size would be deleterious to the production scale. However, this does not apply to flow reactors that are continuously operated. The reduced internal volume guarantees a safer inherent safety:38 in case of rupture or a runaway at a given time, only a minimal amount of chemicals would be released to the environment, hence also improving the direct operational safety. In addition to the direct positive impact on safety of downsizing reactors, the high heat transfer efficiency of flow reactors also enables either the handling of highly exothermic processes or to suppress the formation of problematic byproducts. Along with an improved heat transfer deeply rooted into the much larger surface/volume ratio of flow reactors, structural robustness of the reactor wall is another important factor that unlocks safe use of high pressure for routine operations.
Besides the internal and structural features of flow reactors, the concepts of reaction telescoping (or concatenation)39,40 and of chemical generator41 have been widely developed under flow conditions to enable the safe handling of toxic or highly unstable materials, even at large scale (Scheme 4). A chemical generator in flow feeds upon feed solutions of widely available, stable and non-toxic materials, which upon mixing in a first flow module will react and produce discrete amounts of a highly reactive or unstable chemical. The concatenation of the first flow module to a second, downstream flow module, enables to consume right on the spot the reactive/toxic species with another substrate, hence drastically reducing the inherent risk of processing such chemicals. Such concepts were widely documented by Kappe and coworkers and has become an invaluable tool to implement conditions and chemicals that would otherwise not be allowed in conventional processing units.41–46 This concept is used likewise for the generation of explosive intermediates, such as peroxides, diazo species and azides, hence minimizing the chemical risk with only discrete amounts of explosive materials being generated and consumed right away.47–55
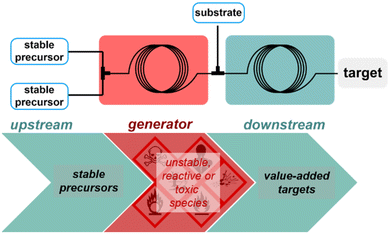 |
| Scheme 4 Reaction concatenation/telescoping in flow: illustration of a chemical generator. | |
On another note, the absence of head-space in flow reactors also allows the implementation of dangerous conditions, such as the use of oxygen with flammable solvents56,57 or to stabilize reactive gaseous species in solution.51
Devices for the disposal of toxic chemicals
As stated above, the wide diversity of chemical processes can be labelled as constructive processes and aim at increasing the molecular diversity and the added value of compounds, starting from widely available and affordable building blocks. For instance, the manufacture of API clearly fits in such definition, where complex reaction sequences or costly catalysts and reagents are acceptable, as long as the added value of the final target leaves room for comfortable margins.58–60Destructive processes are different in essence. Regardless of the end application, the latter aims at the physical or chemical destruction of molecular entities to annihilate safety concerns through a chemical modification.61 In the context of the chemical disposal of chemical warfare agents (CWAs), added-value (in the sense of their production costs and not for their end application) molecular entities with an acute toxicity are neutralized through chemical modification of their backbone toward lower value, lower toxicity entities that can be next safely handled for disposal.61 It comes without saying that such processes inherently come with a reverse economical scheme. An ideal destructive process would therefore rely not only on affordable reagents and catalysts, but also on robust and straightforward process conditions that are amenable to chemical threats of high societal impact.5,61
The design of affordable and robust conditions is not an easy task.60 The affordability of a process comes mostly from the chemicals (ideally off-the-convenience-store) and the operating conditions (ideally room temperature and low pressure), as well as from the process technology that is relied on. The robustness of a process results from the combination of a thorough understanding of the mechanisms at stakes with process technologies that enable steady process conditions. Besides, within the specific context of the neutralization of CWAs, additional important criteria include a potential mobility and high operational safety to make it deployable anywhere with minimal resources.61,62 Miniaturized flow reactors meet all these criteria and they have been shown to allow safe generation/handling of hazardous compounds such as oxidants.41,63–65 Therefore, flow devices are safe systems for the controlled oxidative neutralization of CWA, since the selectivity of the process is a key aspect to afford harmless compounds. This has been successfully performed with commercial oxidizing reagents66,67 or by generating more reactive/unstable oxidants upstream in the flow system.68,69 The fine control of the conditions in the miniaturized reactor avoids the formation of undesired and harmful overoxidized products (Scheme 5).
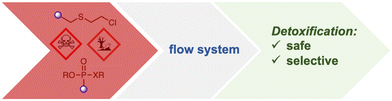 |
| Scheme 5 Safe flow neutralization of CWA and pesticides. | |
Strong bases can also be generated/safely used in dedicated flow systems for the same purpose.70,71 Noteworthy, the treatment of methyl paraoxon in a concatenated sequence (basic hydrolysis/reduction/acylation) allows the cleavage of the side chain of this toxic pesticide and its transformation into the API paracetamol.71
Pharmaceutical sovereignty and factory 5.0
Following up the conclusions of the 2007 Green Chemistry Institute roundtables that identified the next priorities for investment and development in the pharmaceutical production, a thrust toward the implementation of miniaturized flow chemistry reactors was witnessed at the R&D level. A few years later, when the regulatory authorities such as the US Food and Drug Administration (US FDA) started to advocate continuous manufacturing at all stages of an API development, hence leading to a progressive adoption of flow reactors at the production scale. With more than 15 years of technology development, mature flow processes are now thriving. This momentum was further increased in the current context with a succession of global crises causes regular supply disruption of fine chemicals along with prices volatility, with dramatic consequences in all economic sectors. The most visible impacted one is undoubtedly the health system which has undergone shortages in essential drugs, such as anesthetics for intensive care units. To circumvent this issue the relocation of APIs on domestic soils using the “same old recipes” seems unrealistic, and even non-desirable, for several reasons. Obviously, it will not be economically viable to reinvest in large macroreactors to produce old essential – and no longer cost-effective – API that are in the public domain. Even if public health care is a national priority, it might not be bearable on a medium/long-term. More, such factories can only be dedicated to well-established processes for API synthesis with zero impact on research and drug discovery, whereas the emergence of new diseases will require new drugs. Major shortages have triggered a thrust toward re-shoring the production of drug substances to Western countries. However, extremely restrictive environmental and safety policies (e.g., REACH in the European Union) are incompatible with outdated large-scale, stepwise macroscopic batch settings. Besides, in many countries, citizens are now much reluctant to live next door to big chemical plants, which are associated with environmental and health issues. Advanced flow technologies have emerged over the last decade as a robust and viable solution to address both environmental and safety concerns. In this context, a new generation of chemical plants based on miniaturized flow reactors is certainly most promising. Indeed, flow devices allow faster transfer to laboratory to production than batch technology, which is highly desirable for drug discovery. Moreover, not only the reactors are miniaturized in flow systems, but also the usual analytical tools have been significantly downsized such as benchtop-sized NMR as most impressive achievement.72 Thus, the reacting flow passing in a continuous fashion through the analytical tool (inline analysis), real-time information is provided on the reaction course, which offers the possibility to adapt the continuous reaction parameters, mostly through modification of the flow rates (change in stoichiometry, residence time), temperature and pressure.
In a 2016 visionary article (3 years before COVID crisis and subsequent drug shortage), Jamison, Jensen and Myerson already proposed a reconfigurable “fluidic household appliance” for the on-demand synthesis of essential drugs according to U.S. Pharmacopeia standards, namely diphenhydramine, lidocaine, fluoxetine (as hydrochloride salts) and diazepam (Scheme 6).73 The reconfigurable MIT setup was then further improved with an extended scope, more versatile fluidic modules and with advanced automation, robotics and artificial intelligence.74,75
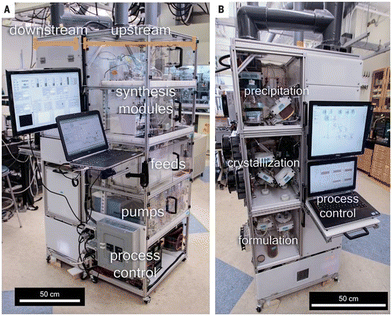 |
| Scheme 6 Reconfigurable flow platform for the on-demand synthesis of some APIs from Jamison, Jensen and Myerson: A. Details of the upstream section of the flow platform featuring feeds, pumps, synthesis modules, and process controls. B. Details of the downstream section of the flow platform featuring precipitation, crystallization, and formulation modules.73 Copyright 2016 The American Association for the Advancement of Science. | |
More recently, Khan and Wu demonstrated that, while counter-intuitive at first glance, multi-step solid-phase synthesis could be implemented in an automated flow system to perform a six-step synthesis of prexasertib in 65% isolated yield after 32 h of continuous execution (Scheme 7).76 A library of 23 analogues of this kinase inhibitor was easily synthesized by feeding the platform inlets with different reagents.
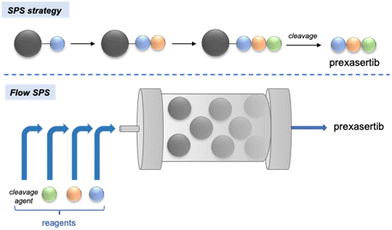 |
| Scheme 7 Automated synthesis of prexasertib and derivatives enabled by continuous-flow solid-phase synthesis (SPS).76 | |
Miniaturized flow reactors offer thus the possibility to perform numerous chemical experiments with few interventions, and provide numerous data to be collected and stored as digital files, also with alternative trends to the more conventional linear approach including radial and cyclic approaches.77 The merging of flow reactors with advanced automation and process analytic technology (PAT) significantly strengthens the robustness and versatility of flow processes, either at the R&D or production scales, and provide invaluable tools for fast optimization.78,79 Combined to intelligent algorithms, it would ultimately lead to autonomous flow platforms able to self-plan and -execute its own synthetic pathway to designated target molecules.79–81 Whereas this road might take some times, efficient black-box algorithms have proven their effectiveness. These algorithms do not require information on the reaction studied and focus on identifying an optimum (yield) rather than generating data to develop reaction models.82,83 For example, Felpin reported a 4-step continuous flow synthesis of a potent anxiolytic compound (FGIN-1-27) from inexpensive and commercially available starting materials (Scheme 8). The use of inline analyses and modified Nelder–Mead optimization algorithm, to assist the decision-making process significantly minimized the number of experiments required in optimization campaigns.84 Further digitalization of chemical processes is expected to become the emerging trend for the next few years.85
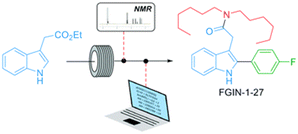 |
| Scheme 8 Continuous flow synthesis of FGIN-1-27 enabled by in-line 19F NMR analyses and optimization algorithms. Copyright 2021 Royal Society of Chemistry.84 | |
Conclusions
The last decade has seen the emergence of miniaturized flow reactors as a possible alternative to classical batch processes for chemical synthesis. Whereas this technology initially appeared as an exotism, or simply a transient technological fashion like the chemical world already experienced in the past (with micro-waves for example), several recent events transformed it into a new paradigm. As often during major crises, a full redesign of several unchanging patterns was required to overcome important issues, especially drug shortages. The re-shoring of fine chemicals can only be restored with a long-term viable approach breaking with the former recipes. Moreover, this re-shoring needs to adapt to the new life model of citizens who do not want to live with a massive chemical plant on the next door. Therefore, continuous flow miniaturized reactors penetrated into industrial research centers with significant momentum over the past five years, as witnessed with frequent job ads emphasizing the need for talents in flow chemistry, through media and press releases, patents or with primary scientific literature in less frequent occurrences. Industrial research centers include flow chemistry both at the R&D and production levels, in an ultimate effort to accelerate the lab-to-market transitions. Some examples of recent public disclosures include the “step-in” announcements of Angelini Pharma (CDMO, Italy) and Medichem (Spain) with substantial investments in lab, pilot and production flow reactors for development and manufacturing of custom APIs.96
Finally, flow devices have an intrinsic connection with automatization since the evolution of a reaction in a flow reactor is controlled by continuous parameters (flow rate), which are easily adjustable by algorithms/computers with the help of and process analytic technology. Whereas artificial intelligence will allow to self-plan a chemical synthesis from the reaction scheme to its execution, it is even possible to design its miniaturised flow system by CAD/additive manufacturing, and therefore to fully conceive a flow synthesis from the device and chemical route to advanced chemical scaffolds.70,86–88
Regarding the flow syntheses of API molecules that have been sorely missing during the COVID crisis, it can be noted the recent reports on the synthesis of anesthetics propofol89–91 and ketamine.92 The synthesis of remdesivir, a broad-spectrum antiviral agent approved against SARS-CoV has also been described,93–95 showing thus the increasing role of flow reactors for the implementation of the miniaturised chemical plants of the future.
Conflicts of interest
There are no conflicts to declare.
Acknowledgements
JL acknowledges the European France(Manche)England cross-border cooperation program INTERREG V A “SmartT”, co-financed by ERDF, for financial support, as well as the University of Rouen Normandy, INSA Rouen Normandy, the Centre National de la Recherche Scientifique (CNRS), Labex SynOrg (ANR-11-LABX-0029), Carnot Institute I2C, the graduate school for research XL-Chem (ANR-18-EURE-0020 XL CHEM) and the Région Normandie. JCMM acknowledges the University of Liège and the F.R.S.-FNRS (Incentive grant for scientific research MIS F453020F).
Notes and references
- R. L. Hartman, J. P. McMullen and K. F. Jensen, Angew. Chem., Int. Ed., 2011, 50, 7502–7519 CrossRef PubMed.
- T. Rodrigues, P. Schneider and G. Schneider, Angew. Chem., Int. Ed., 2014, 53, 5750–5758 CrossRef PubMed.
- D. Webb and T. F. Jamison, Chem. Sci., 2010, 1, 675–680 RSC.
- M. B. Plutschack, B. Pieber, K. Gilmore and P. H. Seeberger, Chem. Rev., 2017, 117, 11796–11893 CrossRef PubMed.
- R. Gérardy, N. Emmanuel, T. Toupy, V.-E. Kassin, N. N. Tshibalonza, M. Schmitz and J.-C. M. Monbaliu, Eur. J. Org. Chem., 2018, 2018, 2301–2351 CrossRef.
- K. S. Elvira, X. C. i Solvas, R. C. R. Wootton and A. J. deMello, Nat. Chem., 2013, 5, 905–915 CrossRef CAS PubMed.
- Z. Dong, Z. Wen, F. Zhao, S. Kuhn and T. Noël, Chem. Eng. Sci.: X, 2021, 10, 100097 Search PubMed.
- M. Guidi, P. H. Seeberger and K. Gilmore, Chem. Soc. Rev., 2020, 49, 8910–8932 RSC.
- C. A. Hone and C. O. Kappe, Chem.: Methods, 2021, 1, 454–467 Search PubMed.
- V.-E. H. Kassin, R. Morodo, T. Toupy, I. Jacquemin, K. V. Hecke, R. Robiette and J.-C. M. Monbaliu, Green Chem., 2021, 23, 2336–2351 RSC.
- V.-E. H. Kassin, T. Toupy, G. Petit, P. Bianchi, E. Salvadeo and J.-C. M. Monbaliu, J. Flow Chem., 2020, 10, 167–179 CrossRef.
- N. Emmanuel, C. Mendoza, M. Winter, C. R. Horn, A. Vizza, L. Dreesen, B. Heinrichs and J.-C. M. Monbaliu, Org. Process Res. Dev., 2017, 21, 1435–1438 CrossRef CAS.
- N. Lamborelle, J. F. Simon, A. Luxen and J.-C. M. Monbaliu, Org. Biomol. Chem., 2015, 13, 11602–11606 RSC.
- P. Bianchi, J. D. Williams and C. O. Kappe, J. Flow Chem., 2020, 10, 475–490 CrossRef.
- D. R. Snead and T. F. Jamison, Chem. Sci., 2013, 4, 2822–2827 RSC.
- C. Holtze and R. Boehling, Curr. Opin. Chem. Eng., 2022, 36, 100798 CrossRef.
-
J. Yoshida, Flash chemistry: fast organic synthesis in microsystems, Wiley, Hoboken, N.J, 2008 Search PubMed.
- J. Yoshida, H. Kim and A. Nagaki, J. Flow Chem., 2017, 7, 60–64 CrossRef.
- H. Usutani, Y. Tomida, A. Nagaki, H. Okamoto, T. Nokami and J. Yoshida, J. Am. Chem. Soc., 2007, 129, 3046–3047 CrossRef PubMed.
- H. Kim, A. Nagaki and J. Yoshida, Nat. Commun., 2011, 2, 264 CrossRef PubMed.
- T. Wirth, Angew. Chem., Int. Ed., 2017, 56, 682–684 CrossRef PubMed.
- H. Kim, K.-I. Min, K. Inoue, D. J. Im, D.-P. Kim and J. Yoshida, Science, 2016, 352, 691–694 CrossRef PubMed.
- D. V. Silva-Brenes, N. Emmanuel, V. L. Mejías, J. Duconge, C. Vlaar, T. Stelzer and J.-C. M. Monbaliu, Green Chem., 2022, 24, 2094–2103 RSC.
- N. N. Tshibalonza and J.-C. M. Monbaliu, Green Chem., 2017, 19, 3006–3013 RSC.
- P. Rys, Acc. Chem. Res., 1976, 9, 345–351 CrossRef.
- J. Yoshida, H. Kim and A. Nagaki, ChemSusChem, 2011, 4, 331–340 CrossRef.
- L. Buglioni, F. Raymenants, A. Slattery, S. D. A. Zondag and T. Noël, Chem. Rev., 2022, 122, 2752–2906 CrossRef PubMed.
- T. Noël, Y. Cao and G. Laudadio, Acc. Chem. Res., 2019, 52, 2858–2869 CrossRef.
- M. Atobe, H. Tateno and Y. Matsumura, Chem. Rev., 2018, 118, 4541–4572 CrossRef.
- D. Pletcher, R. A. Green and R. C. D. Brown, Chem. Rev., 2018, 118, 4573–4591 CrossRef PubMed.
- B. Heinz, D. Djukanovic, P. Filipponi, B. Martin, K. Karaghiosoff and P. Knochel, Chem. Sci., 2021, 12, 6143–6147 RSC.
- P. Musci, T. von Keutz, F. Belaj, L. Degennaro, D. Cantillo, C. O. Kappe and R. Luisi, Angew. Chem., Int. Ed., 2021, 60, 6395–6399 CrossRef PubMed.
- In Sustainable Flow Chemistry, ed. L. Vaccaro, John Wiley & Sons, Ltd, 2017, ISBN: 9783527338528 Search PubMed.
- V. Hessel, M. Escribà-Gelonch, J. Bricout, N. N. Tran, A. Anastasopoulou, F. Ferlin, F. Valentini, D. Lanari and L. Vaccaro, ACS Sustainable Chem. Eng., 2021, 9, 9508–9540 CrossRef.
- L. Vaccaro, D. Lanari, A. Marrocchi and G. Strappaveccia, Green Chem., 2014, 16, 3680–3704 RSC.
-
F. Ferlin, N. N. Tran, A. Anastasopoulou, M. E. Gelonch, D. Lanari, F. Valentini, V. Hessel and L. Vaccaro, in Volume 2 Flow Chemistry - Applications, ed. F. Darvas, G. Dormán, V. Hessel and S. V. Ley, De Gruyter, 2021, pp. 159–192 Search PubMed.
- L. Rogers and K. F. Jensen, Green Chem., 2019, 21, 3481–3498 RSC.
-
Y. Chen, M. Cattoen and J.-C. M. Monbaliu, in Volume 1 Flow Chemistry - Fundamentals, ed. F. Darvas, G. Dormán, V. Hessel and S. V. Ley, De Gruyter, 2021, pp. 269–312 Search PubMed.
- J. Britton and C. L. Raston, Chem. Soc. Rev., 2017, 46, 1250–1271 RSC.
- D. Webb and T. F. Jamison, Chem. Sci., 2010, 1, 675–680 RSC.
- D. Dallinger, B. Gutmann and C. O. Kappe, Acc. Chem. Res., 2020, 53, 1330–1341 CrossRef.
- B. Gutmann, D. Cantillo and C. O. Kappe, Angew. Chem., Int. Ed., 2015, 54, 6688–6728 CrossRef PubMed.
- B. Gutmann and C. O. Kappe, J. Flow Chem., 2017, 7, 65–71 CrossRef.
- G. Glotz, R. Lebl, D. Dallinger and C. O. Kappe, Angew. Chem., Int. Ed., 2017, 56, 13786–13789 CrossRef PubMed.
- F. J. Strauss, D. Cantillo, J. Guerra and C. O. Kappe, React. Chem. Eng., 2016, 1, 472–476 RSC.
- A. S. Kleinke and T. F. Jamison, Org. Lett., 2013, 15, 710–713 CrossRef PubMed.
- B. Gutmann, D. Obermayer, J.-P. Roduit, D. M. Roberge and C. O. Kappe, J. Flow Chem., 2012, 2, 8–19 CrossRef.
- R. M. O'Mahony, D. Lynch, K. S. O'Callaghan, S. G. Collins and A. R. Maguire, Org. Process Res. Dev., 2021, 25, 2772–2785 CrossRef PubMed.
- P. B. Palde and T. F. Jamison, Angew. Chem., Int. Ed., 2011, 50, 3525–3528 CrossRef CAS PubMed.
- K. Donnelly, H. Zhang and M. Baumann, Molecules, 2019, 24, 3658 CrossRef.
- Y. Chen, S. Renson and J.-C. M. Monbaliu, Angew. Chem., Int. Ed., 2022, 61, e202210146 CAS.
- H. Lehmann, Green Chem., 2017, 19, 1449–1453 RSC.
- R. Gérardy, M. Winter, A. Vizza and J.-C. M. Monbaliu, React. Chem. Eng., 2017, 2, 149–158 RSC.
- K. J. Hock and R. M. Koenigs, Chem. – Eur. J., 2018, 24, 10571–10583 CrossRef.
- M. Damm, B. Gutmann and C. O. Kappe, ChemSusChem, 2013, 6, 978–982 CrossRef.
- S. G. Newman, L. Gu, C. Lesniak, G. Victor, F. Meschke, L. Abahmane and K. F. Jensen, Green Chem., 2013, 16, 176–180 RSC.
- C. A. Hone, D. M. Roberge and C. O. Kappe, ChemSusChem, 2017, 10, 32–41 CrossRef CAS PubMed.
- K. Lovato, P. S. Fier and K. M. Maloney, Nat. Rev. Chem., 2021, 5, 546–563 CrossRef CAS.
-
D. Taylor, in Pharmaceuticals in the Environment, 2015, pp. 1–33 Search PubMed.
-
D. Brennan, Process Industry Economics, Elsevier, Netherlands, 2nd edn, 2020 Search PubMed.
- B. Picard, I. Chataigner, J. Maddaluno and J. Legros, Org. Biomol. Chem., 2019, 17, 6528–6537 RSC.
- V. B. Silva, Y. H. Santos, R. Hellinger, S. Mansour, A. Delaune, J. Legros, S. Zinoviev, E. S. Nogueira and E. S. Orth, Green Chem., 2022, 24, 585–613 RSC.
- M. Movsisyan, E. I. P. Delbeke, J. K. E. T. Berton, C. Battilocchio, S. V. Ley and C. V. Stevens, Chem. Soc. Rev., 2016, 45, 4892–4928 RSC.
- L. Wan, M. Jiang, D. Cheng, M. Liu and F. Chen, React. Chem. Eng., 2022, 7, 490–550 RSC.
- M. O'Brien, I. R. Baxendale and S. V. Ley, Org. Lett., 2010, 12, 1596–1598 CrossRef PubMed.
- B. Picard, B. Gouilleux, T. Lebleu, J. Maddaluno, I. Chataigner, M. Penhoat, F.-X. Felpin, P. Giraudeau and J. Legros, Angew. Chem., Int. Ed., 2017, 56, 7568–7572 CrossRef CAS PubMed.
- A. Delaune, S. Mansour, B. Picard, P. Carrasqueira, I. Chataigner, L. Jean, P.-Y. Renard, J.-C. M. Monbaliu and J. Legros, Green Chem., 2021, 23, 2925–2930 RSC.
- N. Emmanuel, P. Bianchi, J. Legros and J.-C. M. Monbaliu, Green Chem., 2020, 22, 4105–4115 RSC.
- V.-E. H. Kassin, D. V. Silva-Brenes, T. Bernard, J. Legros and J.-C. M. Monbaliu, Green Chem., 2022, 24, 3167–3179 RSC.
- S. Mansour, A. Delaune, M. Manneveau, B. Picard, A. Claudel, C. Vallières, L. Sigot, P.-Y. Renard and J. Legros, Green Chem., 2021, 23, 7522–7527 RSC.
- B. M. Sharma, S.-J. Yim, A. Nikam, G.-N. Ahn and D.-P. Kim, React. Chem. Eng., 2021, 6, 1454–1461 RSC.
- M. Rodriguez-Zubiri and F.-X. Felpin, Org. Process Res. Dev., 2022, 26, 1766–1793 CrossRef CAS.
- A. Adamo, R. L. Beingessner, M. Behnam, J. Chen, T. F. Jamison, K. F. Jensen, J.-C. M. Monbaliu, A. S. Myerson, E. M. Revalor, D. R. Snead, T. Stelzer, N. Weeranoppanant, S. Y. Wong and P. Zhang, Science, 2016, 352, 61–67 CrossRef CAS.
- C. W. Coley, D. A. Thomas, J. A. M. Lummiss, J. N. Jaworski, C. P. Breen, V. Schultz, T. Hart, J. S. Fishman, L. Rogers, H. Gao, R. W. Hicklin, P. P. Plehiers, J. Byington, J. S. Piotti, W. H. Green, A. J. Hart, T. F. Jamison and K. F. Jensen, Science, 2019, 365, eaax1566 CrossRef CAS.
- P. Zhang, N. Weeranoppanant, D. A. Thomas, K. Tahara, T. Stelzer, M. G. Russell, M. O'Mahony, A. S. Myerson, H. Lin, L. P. Kelly, K. F. Jensen, T. F. Jamison, C. Dai, Y. Cui, N. Briggs, R. L. Beingessner and A. Adamo, Chem. – Eur. J., 2018, 24, 2776–2784 CrossRef.
- C. Liu, J. Xie, W. Wu, M. Wang, W. Chen, S. B. Idres, J. Rong, L.-W. Deng, S. A. Khan and J. Wu, Nat. Chem., 2021, 13, 451–457 CrossRef.
- S. Chatterjee, M. Guidi, P. H. Seeberger and K. Gilmore, Nature, 2020, 579, 379–384 CrossRef PubMed.
- P. Sagmeister, R. Lebl, I. Castillo, J. Rehrl, J. Kruisz, M. Sipek, M. Horn, S. Sacher, D. Cantillo, J. D. Williams and C. O. Kappe, Angew. Chem., Int. Ed., 2021, 60, 8139–8148 CrossRef PubMed.
- S. Knoll, C. E. Jusner, P. Sagmeister, J. D. Williams, C. A. Hone, M. Horn and C. O. Kappe, React. Chem. Eng. 10.1039/D2RE00208F.
- T. Hardwick and N. Ahmed, Chem. Sci., 2020, 11, 11973–11988 RSC.
- C. R. Sagandira, S. Nqeketo, K. Mhlana, T. Sonti, S. Gaqa and P. Watts, React. Chem. Eng., 2022, 7, 214–244 RSC.
- E. C. Aka, E. Wimmer, E. Barré, N. Vasudevan, D. Cortés-Borda, T. Ekou, L. Ekou, M. Rodriguez-Zubiri and F.-X. Felpin, J. Org. Chem., 2019, 84, 14101–14112 CrossRef CAS PubMed.
- D. Cortés-Borda, K. V. Kutonova, C. Jamet, M. E. Trusova, F. Zammattio, C. Truchet, M. Rodriguez-Zubiri and F.-X. Felpin, Org. Process Res. Dev., 2016, 20, 1979–1987 CrossRef.
- N. Vasudevan, E. C. Aka, E. Barré, E. Wimmer, D. Cortés-Borda, P. Giraudeau, J. Farjon, M. Rodriguez-Zubiri and F.-X. Felpin, React. Chem. Eng., 2021, 6, 1983–1992 RSC.
- S. Rohrbach, M. Šiaučiulis, G. Chisholm, P.-A. Pirvan, M. Saleeb, S. H. M. Mehr, E. Trushina, A. I. Leonov, G. Keenan, A. Khan, A. Hammer and L. Cronin, Science, 2022, 377, 172–180 CrossRef CAS PubMed.
- F. Menzel, T. Klein, T. Ziegler and J. M. Neumaier, React. Chem. Eng., 2020, 5, 1300–1310 RSC.
- M. C. Maier, A. Valotta, K. Hiebler, S. Soritz, K. Gavric, B. Grabner and H. Gruber-Woelfler, Org. Process Res. Dev., 2020, 24, 2197–2207 CrossRef CAS.
- E. Peris, O. Okafor, E. Kulcinskaja, R. Goodridge, S. V. Luis, E. Garcia-Verdugo, E. O'Reilly and V. Sans, Green Chem., 2017, 19, 5345–5349 RSC.
- R. Mougeot, P. Jubault, J. Legros and T. Poisson, Molecules, 2021, 26, 7183 CrossRef CAS PubMed.
- G. M. Martins, M. F. A. Magalhães, T. J. Brocksom, V. S. Bagnato and K. T. de Oliveira, J. Flow Chem., 2022, 12, 371–379 CrossRef CAS PubMed.
- L. Vinet, L. Di Marco, V. Kairouz and A. B. Charette, Org. Process Res. Dev., 2022, 26, 2330–2336 CrossRef CAS.
- V.-E. H. Kassin, R. Gérardy, T. Toupy, D. Collin, E. Salvadeo, F. Toussaint, K. V. Hecke and J.-C. M. Monbaliu, Green Chem., 2019, 21, 2952–2966 RSC.
- T. Vieira, A. C. Stevens, A. Chtchemelinine, D. Gao, P. Badalov and L. Heumann, Org. Process Res. Dev., 2020, 24, 2113–2121 CrossRef CAS.
- T. von Keutz, J. D. Williams and C. O. Kappe, Org. Process Res. Dev., 2020, 24, 2362–2368 CrossRef CAS.
- T. von Keutz, J. D. Williams and C. O. Kappe, Org. Process Res. Dev., 2021, 25, 1015–1021 CrossRef CAS.
-
https://www.teknoscienze.com/tks_article/angelini-launches-flow-chemistry-for-development-and-manufacturing-of-apis/, accessed on 16 October 2022.
|
This journal is © The Royal Society of Chemistry 2023 |