DOI:
10.1039/D2LC00758D
(Paper)
Lab Chip, 2023,
23, 106-114
Behavioural responses of zebrafish with sound stimuli in microfluidics†
Received
14th August 2022
, Accepted 17th November 2022
First published on 18th November 2022
Abstract
Neuronal activities of the human brain responsible for cognitive features have been theorized through several animal models that exhibited various complementary spatial learning modes by generating a flexible repertoire of behavioral strategies. However, for such studies associated with a neurodegenerative disease, which can be further manipulated to provide therapeutic strategies, the animal models employed in their developmental stages have been preferred over the adult ones. This pilot work was incepted to underscore the spatial memory capabilities that strengthened the intricate mechanism of memory acquisition potential in one of the low-order evolutionarily conserved species, such as zebrafish larvae. Initially, a reliable and more easily reproducible microfluidic platform integrating simple and intricate paths was designed to learn and test the spatial information in zebrafish larvae of 4–6 d.p.f. under non-invasive acoustic stimuli. Further, to acquire spatial information as the representation of spatial memory formation in zebrafish larvae, the acoustic startle responses were evaluated by quantifying various dynamic behaviors under distinct operating parameters. After significant conditioning sessions, the spatial memory was tested by employing variable ‘freezing’. By the end of the 30 min-long test session, 6 d.p.f. larvae were found to exhibit the highest value of freezing of approximately 43% and 20% in the short and long paths, respectively. Even though a substantial rate of memory loss was observed, it can be envisaged to serve several behavioral strategies that process the dynamic cognitive memory among distinct spatiotemporal environments. Further, the proposed behavioral paradigm had the advantage of being more adaptable and reliably replicable by other researchers. As a consequence, different hypotheses can be readily tested to generate more reproducible findings towards distinct neurobehavioral characteristics. Therefore, the proposed paradigm for the consolidation of spatial memory based on the non-invasive spatial avoidance strategies could provide an enduring framework of reference for behavioral studies using zebrafish larvae.
Introduction
Learning and memory are the two essential features of all living organisms for survival. In particular, the former referred to the process of memory acquisition, whereas the latter referred to the consolidation and storage of such acquired memory.1 These features of any organism are assessed by habituation, which represents the non-associative form of learning.2 If such habituation arises due to the repeated exposure of the organism in a particular spatial location, then they are referred to as spatial habituation.3 Further, studies on spatial habituation (cognitive and mnemonic features associated with spatial information) can potentially assist in understanding the neuronal activities and the associated disruptions of the organisms.3–5 Since these neuronal circuits involve complex mechanisms,6 significant efforts have been taken to study them in laboratory settings by employing laboratory model organisms.7 Zebrafish is one of such extensively used organisms in various research fields,8 such as learning,9 memory,10 motivation,11 and addiction.12 Simpler and evolutionarily ancient vertebrate brain,13 neurochemistry,14 and neuroendocrine responses similar to mammalian15 are the distinct features established in zebrafish. Further, these features have favoured them as a potential candidate for investigating the intricate functions of the human limbic system.16,17 Though the brain area of zebrafish has not resembled the mammalian hippocampus, recent studies have shown them to be able to acquire and remember spatial information, which is the primary function ascribed to the mammalian hippocampus.18 Due to accelerating multifaceted learning and memory paradigms developed for adult zebrafish,19 researchers have extended their studies on larval zebrafish using tiny well-plates,20,21 petri dishes,22 and microfluidic devices.23–29
Studies on learning and memory in a laboratory setting require an experimental platform to incorporate the control stimuli.30 Such stimuli are difficult to manoeuvre in a three-dimensional environment of conventional platforms such as plates and dishes.23 As an alternative, several microfluidic devices31,32 have been proposed to incorporate the zone of stimuli for manoeuvring the learning processes. Particularly for spatial learning, the stimuli zones are located at different positions in the microfluidic devices to infuse the associated spatial information in the working organism.3 Meanwhile, different types of positive21,33–39 and negative stimuli (motivators)40–45 have been employed to investigate the associated behaviours of zebrafish larvae. A few positive stimuli, such as sight33,34 and computer-based images,35 have been employed to investigate the social behaviour of zebrafish larvae. Other positive motivators, such as auditory cues36,37 and appetitive reinforcement,38 are reported to stimulate zebrafish in a simple associate learning paradigm. On the other hand, negative motivators such as optimal fear-inducing stimuli,40 fish predators and their images,41 optogenetics,42 electric stimuli,43,44 and chemical alarm substances45 have been employed to investigate the fear and anxiety behaviours.
As one of the positive motivators, sound stimuli have been employed to train zebrafish larvae.21,39 Under such stimuli, zebrafish has been observed to manifest a defence response called startle response.46 Such response is arbitrated by Mauthner cells (M-cells), which are large reticulospinal neurons that acquire information from ipsilateral sensory afferents and synapses to contralateral spinal motor neurons.47,48 Motor neurons will be activated collaterally after being startled, and then the zebrafish bends into a “C” shape and escapes from the source of stimulation.49 Consequently, habituation can evolve when the response of a stimulation-generated startle decreases.50 Since spatial habituation is strongly associated with the interactions of the organism in its environment,51 different spatial environments were integrated into the proposed microfluidic device to quantify the behavioural responses of 4–6 d.p.f. zebrafish larvae under sound stimuli. Eventually, a systematic investigation was further carried out to quantify the formation and extinction of the acquired spatial information by employing the variable latency and freezing, respectively. The presented paradigm, together with the observed cognitive features, could potentially assist in understanding the complex mechanisms involved in learning and memory. Subsequently, this could pave the way for addressing numerous human disorders associated with memory impairment and memory loss.
Methods
Design and fabrication of the microfluidics
To examine various behavioural responses of the zebrafish within the microfluidic environment, a novel microfluidic device was proposed to learn and test zebrafish for spatial memory using sound stimuli. The microfluidic device was fabricated through a sequence of computerized numerical control micromachining methodologies and polydimethylsiloxane (PDMS) casting processes. The microchannel design outline was framed using commercial design software (Solid Works, Dassault Systems SolidWorks Corp., Waltham, MA, USA), and the design outline was engraved on an acrylic substrate (5 mm in thickness) using the CNC (Computer numerical control) micromachining technique. In the fabrication process of the microfluidic device, PDMS (Sylgard 184, Dow corning Corp., Midland, MI, USA) and curing agent in a ratio (10
:
1) were evenly mixed, degassed in a vacuum chamber, and added into the acrylic mold.52 Furthermore, the fabricated microfluidic structure was incubated on a hot plate at a temperature of 75 °C for about 10 h for the curing process. Finally, the PDMS channel was separated from the mold, and the inlet & outlet sections were punched through a biopsy puncher. In order to avoid fluid leakage, the microchannel was eventually bonded with another PDMS layer through an oxygen plasma process. The design details of the employed microfluidic devices are illustrated in Fig. 1a and b. Further, various stages involved in the fabrication process of the employed microfluidic devices are illustrated in ESI† Fig. S1. To evaluate the spatial memory of zebrafish larvae, various motion characteristics such as the curve speed (VCL, mm s−1), straight-line speed (VSL, mm s−1), and linearity of the progress (LIN = VSL/VCL) were investigated. Initial investigations were enacted to inspect the proposed devices' efficacy and optimize the application parameters. An ultrasonic transducer (400E08S) was employed to generate the acoustic stimuli for zebrafish larvae. This transducer was connected to a high-resolution digital class D audio amplifier, and thus the sound waves of different frequencies (200, 600, and 1200 Hz) and waveforms (sawtooth, triangle, and square) were generated using a graphical programming language (LabVIEW, National Instruments, Austin, triangular waveform (ESI† Fig. S2)). Therefore, the triangular waveform was employed for the transducers to generate a significant sound pressure level inside the microfluidic chip. Zebrafish larvae of different developmental stages (4–6 d.p.f.) were injected in the inlet section, and the corresponding spatial memory was quantified under the induction of acoustic stimuli. Zebrafish larvae responses were recorded using a camera mounted on a tripod. Open source Tracker software (OpenSource Physics, OPS) was employed to analyze various dynamic behaviours of zebrafish larvae, which are discussed in detail in the Results and discussion section. The schematic representation of the proposed experimental setup is illustrated in Fig. 1c. Further, various stages involved in the acoustically assisted training process of zebrafish larvae are demonstrated in Fig. 1d.
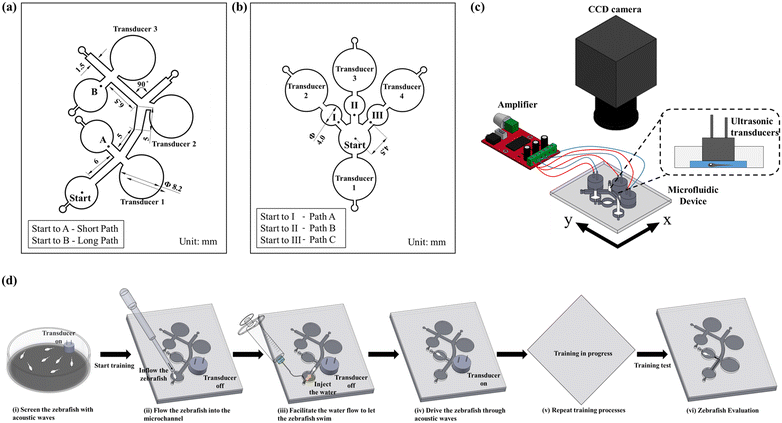 |
| Fig. 1 Schematic illustrations of the proposed microfluidic chip designs and the employed experimental setup. (a) Illustration of the geometric design details integrating short and long paths in the microfluidic device. With the help of sound stimuli generated by the transducers, zebrafish larvae were transported in these paths to learn spatial information. (b) Geometric design details of the microfluidic chip employed to test the reliability of the induced startle response (C-bend) elicited by the proposed experimental paradigm. (c) Illustration of the audio system setup to control the sound waves. (d) Illustration of various stages in successive learning and testing practices of zebrafish larvae. | |
Zebrafish culture
Wild-type (AB) 4–6 d.p.f. zebrafish larvae (D. rerio) were obtained from an adult zebrafish colony housed at the National Cheng Kung University. All embryos were bathed in pure water and cultured in a noise-free environment at 27 °C ± 1 °C. The solution was replaced every 24 hours and the unhealthy embryos were eliminated. At 4–6 d.p.f., zebrafish larvae were transported to the inlet section of the experimental apparatus using a dropper in order to minimize the damage to the lateral line neuromasts. Animal rearing and experimental procedures were approved by the National Cheng Kung University Institutional Animal Care and Use Committee (IACUC) with approval number: 108269.
Statistical methods
Statistical analyses were performed on the swimming behaviors, success rate, latency, and freezing data using the commercially available software SPSS (SPSS, Chicago, IL, USA). Further, the statistical significance of the data was calculated using the one-way ANOVA Scheffe method, and a *P value of ≤0.05 is considered statistically significant. The sample size was estimated using power analysis with a significance level of 0.05 and a power of 80%. The data represented as mean ± SD are obtained from three independent experiments, and the normal distribution was confirmed using Shapiro–Wilk tests.
Behavioural responses quantification
In order to investigate the escape avoidance behaviour of zebrafish larvae under acoustic stimuli, it is essential to study the degree of startle responses that the sound transducer can induce in such an animal model. Consequently, the transducers were employed to induce a significant sound pressure gradient inside the microfluidic platform. Such an induced sound pressure level (SPL) was observed to elicit a significant stimuli avoidance behaviour. Subsequently, the sound pressure level LP generated by the transducer was quantified as | 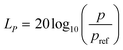 | (1) |
where p is the root mean square of the sound pressure, and pref is the reference value of the sound pressure.
Furthermore, to quantify the degree of startle responses, various motion characteristics53 such as curvilinear velocity (VCL, mm s−1), straight-line velocity (VSL, mm s−1), and the linearity of the progress (LIN = VSL/VCL) were analysed. The VCL was employed to measure the average velocity over the actual point-to-point track followed by larvae. It can be expressed as
|  | (2) |
where
DT is the total distance of zebrafish larvae displaced. On the other hand, the VSL was employed to measure the time-average velocity of a larva head along the straight line between its first and last detected positions. It can be expressed as
|  | (3) |
where
Di–f is the distance measured from the initial to the final location of zebrafish larvae.
Memory quantification
Formation of spatial memory.
In order to capture the degree of learning in zebrafish larvae, a parameter called latency was employed. Eventually, this parameter measured the duration of the response time between the stimuli.54 Repeated conditioning sessions were carried out to facilitate the representation of spatial information in zebrafish larvae. Further, after each conditioning session, the value of latency was quantified.
Quantification of memory extinction.
In this test session, the sound stimulation was switched off, and the spatial memory of zebrafish larvae was quantified. Further, the memory extinction parameter called freezing was employed to investigate the memory loss of conditioned aversion in zebrafish larvae. Eventually, this parameter quantified the degree of memory that existed over a duration of time.55 Moreover, zebrafish larvae subjected to eight subsequent conditioning sessions were employed for freezing quantification. This parameter can be expressed as | 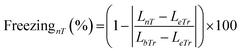 | (4) |
where LnT is the latency at nth time (5, 10, 15, 20, 25 and 30 min), LeTr is the latency quantified at the end of the conditioning sessions (at 10th trials), and LbTr is the latency quantified at the pre-conditioning session (the time taken by zebrafish larvae to reach the target section without any stimulation).
Results and discussion
To acquire spatial memory as the representation of spatial information through the proposed microfluidic platform, zebrafish larvae of 4–6 d.p.f. were tested under sound stimuli with distinct operating parameters such as different waveforms (sawtooth, triangle, and square) and frequencies (200, 600, and 1200 Hz). Meanwhile, to successfully drive and test this organism under acoustic stimuli, it is essential to generate a significant pressure gradient in the flow field. Such a distribution of sound pressure levels (SPL) was achieved by placing the sound transducers, which manipulated the direction of zebrafish larvae towards the targeted zone (ESI† Video S1). For instance, Fig. 2a shows the simulated distribution of SPL where the smooth transition from the region around the transducer (85 dB) towards the targeted zone (57 dB) can be observed, which shepherded the larvae to reach the zone with diminished auditory cues (target region). At this point, it should be noted that the maximum SPL generated by the employed transducer was well below 130–150 dB. Since the latter has been reported to induce physiological damages such as temporary hearing loss and hair cell trauma56 that could potentially strain the cognitive function of the larvae, the former range was adopted to non-invasively test the larvae for acquiring the spatial information. Further, such a conditioning process has been demanded to significantly induce the startle behaviours under external stimuli.
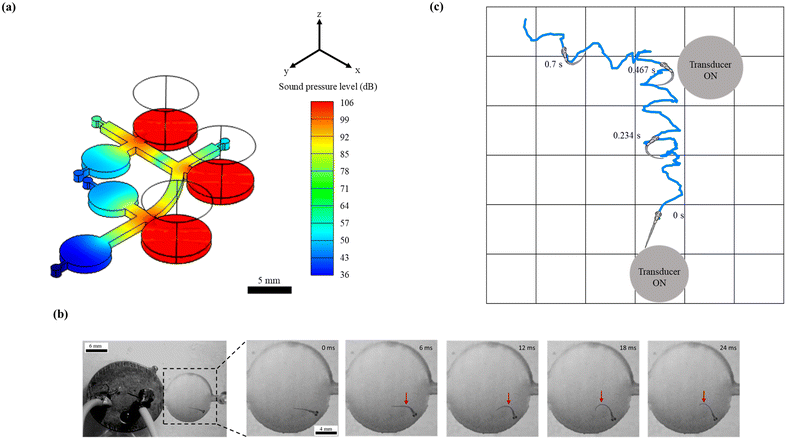 |
| Fig. 2 Elicitation of the C-start reflex in zebrafish larvae under sound stimuli. (a) Distribution of the sound pressure level in the microfluidic device simulated numerically at 1200 Hz. (b) Representative time-lapse images to illustrate the C-start escape behavior of 4 d.p.f. zebrafish larva in response to the acoustic stimulus. The red arrow indicates the region of maximum body bend (C-shape) at a given time. The C-shaped bend was observed to originate at the central region of the body, as shown in this figure at 6 ms. This bend was further observed to attain its maximum at 12 ms. Subsequently, this maximum bend was then observed to move along the body and eventually reached the tail, as shown in this figure at 24 ms. With this observation, it was confirmed that zebrafish larvae used their entire body to escape (as a consequence of C-start). (c) A representative larval motion is shown to illustrate the spatial exploration of zebrafish larvae exposed to acoustic signals. A grid background with a cell side length of 1.5 mm (same as the diameter of the microchannel) is employed to visualize the larval motion in two-dimensional space. The position of transducers was adequately chosen to elicit the larval motion in the horizontal and vertical flow paths inside the microchannel. During this experiment, both the transducers were kept in the ON state. Unlike the typical wall-seeking behavior (larval motion along the wall surface of the channel), the larvae were observed to explore the entire cross-section of the microchannel during such acoustically assisted transportation processes. | |
Among various escape responses of zebrafish larvae, the rapid C-start reflex medicated by a relatively simple neural network has been attracted by researchers as one of the essential vertebrate model behaviours for neurological investigations.57–60 It was observed from Fig. 2b that the larvae close to the transducer exhibited a positive C-start within 12 ms of acoustic stimulation. Further, such an escape reflex was noticed to stray their path followed by surged forward swimming which assisted the organism to precisely drive towards the targeted zone. To demonstrate this behaviour, zebrafish larvae of 4. d.p.f. were placed near the transducer (maximum SPL of 103 dB) that was operated at 1200 Hz. Consequently, the C-start was initiated at 8 ms, and the larvae attained the maximum C-bend at 12 ms, where a rapid forward swimming motion was expected (ESI† Video S2). Since this experiment was explicitly designed to show the C-start elicitation, it was conducted in a fully bounded region, and hence the forward movement cannot be observed. The induced sound pressure level (SPL) was observed to elicit a significant stimuli avoidance behaviour. Such an intensity of SPL was observed to be in conjunction with the prior studies that reported the essential SPL to initiate the C-start reflex in zebrafish larvae.36 Apart from this, the representative larval motion, as shown in Fig. 2c and ESI† Video S1, demonstrated the ability of the proposed experimental paradigm to successfully drive this organism to the targeted zone. Further, larvae were observed to manipulate the flow path by eliciting the C-bend under the application of sound stimuli. Such a phenomenon was observed to assist the larvae in imbibing spatial information after several trials
Several swimming parameters of larvae, such as VCL, VSL, and linearity, were quantified and considered as a repercussion of acoustic signals outraged in zebrafish larvae (Fig. 3). Moreover, the quantification of these parameters can be treated as an unprecedented approach to depict the dynamic behaviour of this organism under acoustic stimuli. Among the considered larvae stages, the swimming behaviours in 4 d.p.f. were observed to be starker than in the others (5 and 6 d.p.f.). The detailed illustration and comparison of these dynamic parameters under different waveforms and frequencies for the larval stages of 4–6 d.p.f. are shown in ESI† Fig. S3. Although the latter being older, has well-developed hearing organs, the no-feed situation makes them weaker. Such a deteriorating state has been reported to provoke significantly diminished swimming behaviors,61 dampening their responses to acoustic signals. Therefore, to obtain the optimized operating parameters, the swimming behaviours of 4 d.p.f. larvae were further experimented with distinct waveforms and frequencies, as shown in Fig. 3. It was observed that the larvae of 4 d.p.f. exposed to a sawtooth wave with a frequency of 1200 Hz had significant swimming characteristics with an estimate for the VSL and VCL of 8.94 ± 5.14 and 19.98 ± 14.51 mm s−1, respectively. On the other hand, for the linearity concerns, the lower the value, the better the induced startle behaviour. Further, this variable was observed to be relatively insignificant to the signal waveforms compared to signal frequencies and larval ages. In addition, it was noted that the value of linearity dropped significantly at a higher frequency (1200 Hz). This observed superior response at a higher frequency was similar to several published works that reported the effective signal frequency to elicit escape movements.36 In addition to the signal waveforms, the frequency of 1200 Hz was employed to train zebrafish larvae in imbibing spatial information through the proposed microfluidic platform.
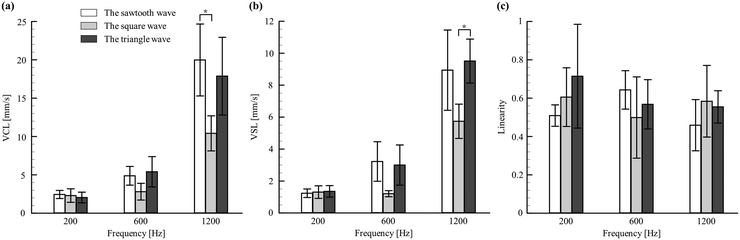 |
| Fig. 3 Swimming behaviors of 4 d.p.f. zebrafish larvae in response to the change of signals over selected frequencies. (a) The curvilinear velocity (VCL). (b) The straight-line velocity (VSL). (c) The linearity (LIN). Values are reported as mean ± SD. N = 20 and P values were determined by the one-way ANOVA Scheffe method, *P ≤ 0.05. | |
In order to explore the spatial memory features induced under startle responses, it has been necessary to inhibit the reliable startle stimulus that elicited robust escape and avoidance behaviours, which eventually propelled the larvae to the target section. Regardless of the swimming pathways and larval stages, it was observed that the employed external stimuli induced innate startle responses, which consequently drove the larvae to the target sections. However, the time required to reach the target section was observed to vary significantly with the larval stages. For demonstration purposes, the larvae of 4–6 d.p.f. were exposed to the acoustic signals integrated into the chip engraved with three different pathways associated with corresponding target sections, as shown in Fig. 4. Despite the induction pathways, it was observed that the larvae of all the considered stages were successfully transported to the target zone within 1.5 s. In addition, at any given time, 4 d.p.f. larvae were observed to exhibit a superior success rate compared to other stages of larvae. These results showed the consistency and accuracy of the developed experimental paradigm to induce startle reflex in zebrafish larvae, eventually attaining the target sections non-invasively. Further, such demonstrations substantiated the reliability of the proposed experimental paradigm to strengthen the outcomes of further experiments. In addition, such systematic investigation of the methodological protocol could be essential to rule out the inconsistency among the experimental designs, particularly to attain better reproducibility of behavioural neuroscience studies using zebrafish.
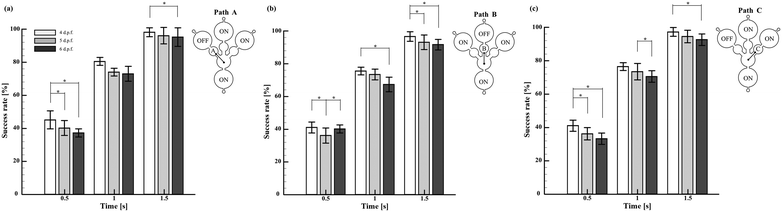 |
| Fig. 4 Efficacy of the proposed experimental paradigm in transporting the larvae to the target zone. To perform this test, microfluidic chips with three different flow paths, namely, Path A (a), Path B (b), and Path C (c), were designed and fabricated. These paths had similar designs and geometries. Only the flow direction associated with them was different. The variable ‘Success rate’ was employed to indicate the proximity of larvae in reaching the target zone at a given flow path. Irrespective of the flow paths, it was observed that the larvae of all the considered stages were successfully transported to the target zone within 1.5 s. In addition, at any given time, 4 d.p.f. larvae were observed to exhibit a superior success rate compared to other stages of larvae. The values are reported as mean ± SD. N = 20 larvae per experiment in three independent trials. P values were determined by the one-way ANOVA Scheffe method, *P ≤ 0.05. | |
To determine whether zebrafish larvae are capable of spatial learning, the established behavioural indices such as the startle response and habituation5 were quantified by employing a simple yet reliable experimental paradigm. Such a paradigm was configured with the sound transducers that acted as conditioned place avoidance in which the spatial memory features of different larval stages were explored. A few recent studies have demonstrated that the environment where the larvae were trained has a strong dependence on the degree of acquired spatial memory.62 Consequently, the proposed microfluidic platform was designed to enable the simple as well as the complex path for the systematic quantification and assessment of spatial memory through acoustically induced startle reflex habituation (Fig. 5 and ESI† Video S3). The experiments were conducted in two consecutive sessions. During the first session (conditioning), the larvae were tested to avoid the acoustic stimuli that allowed them to change their swimming paths. Such an escape behaviour was successfully demonstrated through the proposed platform to drive the larvae to the target zone, which inherently assisted the formation of spatial memory. In the second session (test), the acoustic stimulation was switched off, and the memory extinction rate of the larvae was tested. Eventually, two measures were employed to quantify the memory effects of conditioning such as latency (stimulation ON) and freezing (stimulation OFF). From the experiments, it was found that the larvae of stages 4–6 d.p.f. could significantly improve the memory of spatial information after multiple sessions of training. For instance, before training (conditioning), the latencies of 4–6 d.p.f. larvae in the short path and long path were 7.5 ± 1.8, 7.2 ± 1.6 and 6.9 ± 1.3 s, and 55.5 ± 3.8, 53.3 ± 5.5 and 54 ± 4.2 s, respectively. However, after multiple sessions of training (seven trials), the latencies of 4–6 d.p.f. larvae in both the paths significantly decreased (Fig. 5(a and b)). Further, there was no significant difference observed between the latencies of the larval stages in the short path training (simple environment) (Fig. 5(a)). However, in the complex environment (long path), the larvae of 6 d.p.f. were found to acquire spatial information more effectively than the other considered stages. Such behaviour was due to the relatively developed organs and cells seen at this developmental stage that could enable the larvae to remarkably acquire spatial information even in the complex environment.63 Moreover, this stage of larvae has been shown to exhibit long-term habituation behaviours significantly, during which memory formation can occur effectively.5 Conclusively, the presented simple paradigm was demonstrated to be more adaptable for testing different experimental hypotheses (simple and complex environments) that could bring a step forward towards reaching better replicability and reproducibility of behavioural neuroscience research in zebrafish. Apart from the process of aversive memory formation, the proposed platform was also employed to quantify the extinction of such memory during which the acoustic signals were switched off. Regardless of the paths, it can be revealed that the larvae remembered the location of spatial avoidance significantly in the first 5 min of the test session. Irrespective of paths and various time intervals in the test session, the larvae of 6 d.p.f. were observed to retain their aversive memory significantly compared to other stages. For instance, in the short path, the value of freezing quantified at 5 min for 4 and 6 d.p.f. larvae was 85.9 ± 4.3% and 89.4 ± 4.6%, respectively. Similarly, the value of freezing quantified at 20 min for 4 and 6 d.p.f. larvae was 50.6 ± 5.4% and 63.8 ± 4.4%, respectively. Even though such a superior performance of 6 d.p.f. larvae did not differ throughout the test session, the value of freezing quantified at the end of the test session was observed to diminish remarkably. In particular, the conditioned aversion was observed to deteriorate rapidly in the long path and attained the minimum value at the end of the test session. For instance, the values of freezing quantified at the 30 min-long test session for 6 d.p.f. zebrafish larvae in the short and long paths were 42.5 ± 4.8% and 19.3 ± 3.7%, respectively. This trend represented the diminished behaviour of the spatial avoidance by the end of the 30 min-long test session. Such observed rapid memory recovery (dishabituation) further supported the authors to believe that the decrease of latency (as discussed earlier) might be the consequence of spatial habituation. In addition, these behaviours of memory extinction were observed to be in contrast with the stimuli avoidance studies reported in rodents. Typically, rodents have shown a strong and lasting aversion to the stimuli location after sufficient training.64 In contrast, based on the obtained results from the present study, zebrafish larvae were found to exhibit quick memory loss that strongly abandoned the spatial information learned during the conditioning process. Such a behaviour may be interpreted as evidence of inadequate learning. Further, it is to be noted that the mechanism behind the gradual rapid loss of stimuli aversion in the test session could be passive or active.65 Therefore, to address and distinguish between these states of memory loss, further experiments are necessary by reinstalling and reintroducing the acoustic stimuli. Nevertheless, this rapid memory extinction may serve a different behavioural strategy.66,67 Such behavioural strategies could be envisaged to unravel the disruption induced in the human brain structure responsible for spatial cognitive functions associated with the wandering behaviour that generally occurs in patients with neurodegenerative and neuropsychiatric disorders.
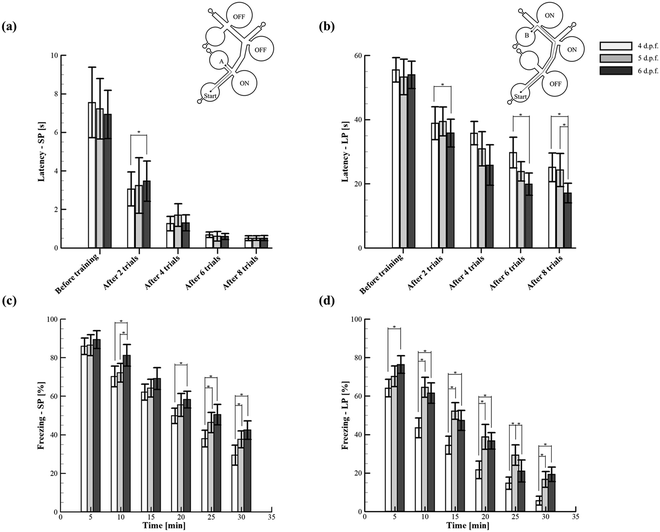 |
| Fig. 5 Quantification of spatial memory formation and extinction in zebrafish larvae under different spatial environments. (a) and (b) Latency was quantified to depict the spatial memory formation in short (SP) and long (LP) paths. It was observed that the latency decreased with the number of repeated trials. Specifically, this unfolded the occurrence of habituation in which zebrafish larvae had undergone a transformation from non-associative learning to associative learning. (c) and (d) Freezing was quantified to depict the extinction of spatial memory in short (SP) and long (LP) paths. Irrespective of the paths, freezing quantified for 6 d.p.f. larvae was observed to be superior compared to that of other considered larval stages. Further, freezing quantified in the short path was observed to decrease gradually with time. In contrast, a rapid decline of freezing was observed in the long path. Consequently, it can be inferred that the spontaneous recovery of the acquired memory was more profound in complex environments than the simple ones. Values are reported as mean ± SD. N = 20 larvae per experiment in three independent trials. P values were determined by the one-way ANOVA Scheffe method, *P ≤ 0.05. | |
Conclusion
In summary, the microfluidic platform reported here provided novel insights into the influence of distinct larval stages of zebrafish and stimuli parameters on the spatial memory features as the function of startle reflex habituation. Through the simple yet reliable behavioural paradigm, both the simple and complex habituation environments were systematically quantified and explored. The proposed paradigm could allow other researchers to perform easier and reliable replication for generating more reproducible findings. The non-invasive spatial guidance strategies of zebrafish larvae were uncovered, which could serve as a step forward towards revealing the flexible neural mechanisms underlying the neurological behaviour. The results shown here were integrated into a broader context of related findings in the literature that highlight the common grounds and attributes that still necessitate elucidation. Finally, the proposed methodological approach and the conclusions can provide an enduring framework of references for behavioural studies using zebrafish larvae.
Author contributions
Conceptualization: C. C.; methodology: S. W. and C. C.; investigation: S. W. and D. L.; supervision: C. C.; writing – original draft: D. L. and S. W.; writing – review & editing: D. L. and C. C. All authors have read and agreed to the published version of the manuscript.
Conflicts of interest
There are no conflicts to declare.
Acknowledgements
This study was supported through the Ministry of Science and Technology of Taiwan under Contract No. MOST 108-2221-E-006-221-MY4 (to Chia-Yuan Chen). This work would not be possible without the facility provided by the Center for Micro/Nano Science and Technology, National Cheng Kung University. This research was supported in part by the Higher Education Sprout Project, Ministry of Education to the Headquarters of University Advancement at National Cheng Kung University (NCKU).
References
- A. C. Roberts, B. R. Bill and D. L. Glanzman, Front. Neural Circuits, 2013, 7, 1–11 CrossRef PubMed.
- J. Larsch and C. Pantoja, Curr. Biol., 2019, 29, R292–R294 CrossRef CAS PubMed.
- K. Yashina, Á. Tejero-Cantero, A. Herz and H. Baier, iScience, 2019, 19, 119–134 CrossRef PubMed.
- A. C. Roberts, K. C. Pearce, R. C. Choe, J. B. Alzagatiti, A. K. Yeung, B. R. Bill and D. L. Glanzman, Neurobiol. Learn. Mem., 2016, 134, 360–368 CrossRef CAS PubMed.
- A. C. Roberts, J. Reichl, M. Y. Song, A. D. Dearinger, N. Moridzadeh, D. Elaine, K. Pearce, J. Esdin and D. L. Glanzman, PLoS One, 2011, 6, e29132 CrossRef CAS PubMed.
- J. A. Weiner, J. D. Jontes and R. W. Burgess, Front. Mol. Neurosci., 2013, 6, 1–2 Search PubMed.
- N. Frey, U. M. Sönmez, J. Minden and P. LeDuc, Nat. Commun., 2022, 13, 1–11 Search PubMed.
- T. Y. Choi, T. I. Choi, Y. R. Lee, S. K. Choe and C. H. Kim, Exp. Mol. Med., 2021, 53, 310–317 CrossRef CAS PubMed.
-
R. Gerlai, in Methods in Cell Biology, Elsevier Ltd, 2016, vol. 134, pp. 551–586 Search PubMed.
- M. Bruzzone, E. Gatto, T. L. Xiccato, L. D. Valle, C. M. Fontana, G. Meneghetti and A. Bisazza, PeerJ, 2020, 8, e8890 CrossRef PubMed.
- E. J. Horstick, T. Mueller and H. A. Burgess, J. Neurogenet., 2016, 30, 122–132 CrossRef CAS PubMed.
- F. M. Nathan, C. Kibat, J. S. Tanisha Goel, A. Claridge-Chang and A. S. Mathuru, Addict. Biol., 2022, 27, e13126 CAS.
- M. Ware, V. Dupé and F. R. Schubert, Dev. Dyn., 2015, 244, 1202–1214 CrossRef PubMed.
- L. J. Jones, J. E. McCutcheon, A. M. J. Young and W. H. J. Norton, Front. Behav. Neurosci., 2015, 9, 1–16 Search PubMed.
- A. V. Kalueff, A. M. Stewart and R. Gerlai, Trends Pharmacol. Sci., 2014, 35, 63–75 CrossRef CAS PubMed.
- K. Kodera and H. Matsui, Int. J. Mol. Sci., 2022, 23, 1399 CrossRef CAS PubMed.
- R. Nakajima, H. Hagihara and T. Miyakawa, Mol. Brain, 2021, 14, 1–5 CrossRef PubMed.
- R. Gerlai, J. Neurosci. Methods, 2014, 234, 54–58 CrossRef PubMed.
- B. D. Petersen, K. T. Bertoncello and C. D. Bonan, Front. Pharmacol., 2022, 13, 1–7 Search PubMed.
- Q. Shen, L. Truong, M. T. Simonich, C. Huang, R. L. Tanguay and Q. Dong, Behav. Brain Res., 2020, 391, 112625 CrossRef CAS PubMed.
- J. J. F. G. Hunt, S. C. Clarke, A. Fleming and J. D. Best, Neuropsychopharmacology, 2008, 33, 1206–1215 CrossRef PubMed.
- M. Q. Jin, D. Zhang, Y. Zhang, S. S. Zhou, X. T. Lu and H. T. Zhao, J. Zhejiang Univ., Sci., B, 2018, 19, 400–408 CrossRef CAS PubMed.
- A. Khalili and P. Rezai, Briefings Funct. Genomics, 2019, 18, 419–432 CrossRef PubMed.
- K. Mani and C. Y. Chen, Microfluid. Nanofluid., 2021, 25, 22 CrossRef CAS.
- A. Khalili, E. van Wijngaarden, K. Youssef, G. R. Zoidl and P. Rezai, Biotechnol. J., 2022, 17, 2100076 CrossRef CAS PubMed.
- S. Subendran, Y. C. Wang, Y. H. Lu and C. Y. Chen, Sci. Rep., 2021, 11, 13801 CrossRef CAS PubMed.
- Y. Lee, H. W. Seo, K. J. Lee, J.-W. Jang and S. Kim, Sensors, 2020, 20, 5903 CrossRef CAS PubMed.
- S. Subendran, C.-W. Kang and C. Y. Chen, Micromachines, 2021, 12, 68 CrossRef PubMed.
- B. Panigrahi and C.-Y. Chen, Micromachines, 2019, 10, 880 CrossRef PubMed.
- J. Xu, R. Casanave and S. Guo, Learn. Mem., 2021, 28, 228–238 CrossRef CAS PubMed.
- R. Candelier, M. Sriti Murmu, S. Alejo Romano, A. Jouary, G. Debrégeas and G. Sumbre, Sci. Rep., 2015, 5, 1–10 Search PubMed.
- A. Khalili, E. van Wijngaarden, G. Zoidl and P. Rezai, Biotechnol. J., 2021, 24, 423–432 Search PubMed.
- D. M. Higgs, A. K. Rollo, M. J. Souza and A. N. Popper, J. Acoust. Soc. Am., 2003, 113, 1145–1154 CrossRef PubMed.
- K. Mani, Y. C. Hsieh, B. Panigrahi and C. Y. Chen, Biomicrofluidics, 2018, 12, 021101 CrossRef PubMed.
- S. Kato, T. Nakagawa, M. Ohkawa, K. Muramoto, O. Oyama, A. Watanabe, H. Nakashima, T. Nemoto and K. Sugitani, J. Neurosci. Methods, 2004, 134, 1–7 CrossRef PubMed.
- A. A. Bhandiwad, D. G. Zeddies, D. W. Raible, E. Rubel and J. Sisneros, J. Exp. Biol., 2013, 216, 3504–3513 CrossRef PubMed.
- K. Mani and C. Y. Chen, Biomicrofluidics, 2021, 15, 014109 CrossRef CAS PubMed.
- J. M. Daggett, V. J. Brown and C. H. Brennan, Behav. Brain Res., 2019, 359, 190–196 CrossRef PubMed.
- R. E. Poulsen, L. A. Scholz, L. Constantin, I. Favre-Bulle, G. C. Vanwalleghem and E. K. Scott, Curr. Biol., 2021, 31, 1977–1987.e4 CrossRef CAS PubMed.
- R. M. Luca and R. Gerlai, Behav. Brain Res., 2012, 226, 66–76 CrossRef PubMed.
- R. M. Colwill and R. Creton, Rev. Neurosci., 2011, 22, 63–73 CrossRef PubMed.
- K. Y. Lee, S. J. Park, D. G. Matthews, S. L. Kim, C. A. Marquez, J. F. Zimmerman, H. A. M. Ardoña, A. G. Kleber, G. V. Lauder and K. K. Parker, Science, 2022, 375, 639–647 CrossRef CAS PubMed.
- A. Khalilia, E. van Wijngaardena, G. R. Zoidlb and P. Rezai, Sens. Actuators, A, 2021, 332, 113070 CrossRef.
- P. J. Steenbergen, J. Neurosci. Methods, 2018, 301, 52–61 CrossRef PubMed.
- A. R. Peimani, G. Zoidl and P. Rezai, Biomicrofluidics, 2018, 12, 014113 CrossRef PubMed.
- M. Takahashi, M. Inoue, M. Tanimoto, T. Kohashi and Y. Oda, Neurosci. Res., 2017, 121, 29–36 CrossRef PubMed.
- R. C. Eaton, R. A. Bombardieri and D. L. Meyer, J. Exp. Biol., 1977, 66, 65–81 CrossRef CAS PubMed.
- R. C. Eaton, R. K. Lee and M. B. Foreman, Prog. Neurobiol., 2001, 63, 467–485 CrossRef CAS PubMed.
- S. A. Weiss, S. J. Zottoli, S. C. Do, D. S. Faber and T. Preuss, J. Exp. Biol., 2006, 209, 4788–4801 CrossRef PubMed.
- C. Beppi, D. Straumann and S. Y. Bögli, Sci. Rep., 2021, 11, 846 CrossRef CAS PubMed.
- R. Spence, A. E. Magurran and C. Smith, Anim. Cogn., 2011, 14, 607–612 CrossRef PubMed.
- Y.-F. Wang, I.-W. Chen, S. Subendran, C.-W. Kang, B. Panigrahi, T.-F. Fu and C.-Y. Chen, Sci. Rep., 2020, 10, 16423 CrossRef PubMed.
- B. Panigrahi and C.-Y. Chen, Lab Chip, 2019, 19, 4033–4042 RSC.
- B. T. N. Hieu, N. T. N. Anh, G. Audira, S. Juniardi, R. A. D. Liman, O. B. Villaflores, Y. H. Lai, J. R. Chen, S. T. Liang, J. C. Huang and C. Der Hsiao, Int. J. Mol. Sci., 2020, 21, 1–12 Search PubMed.
- A. I. Faustino, A. Tacão-Monteiro and R. F. Oliveira, Sci. Rep., 2017, 7, 1–10 CrossRef PubMed.
- L. Breitzler, I. H. Lau, P. J. Fonseca and R. O. Vasconcelos, Hear. Res., 2020, 391, 107952 CrossRef PubMed.
- K. S. Liu and J. R. Fetcho, Neuron, 1999, 23, 325–335 CrossRef CAS PubMed.
- T. Kohashi and Y. Oda, J. Neurosci., 2008, 28, 10641–10653 CrossRef CAS PubMed.
- J. R. Fetcho and K. S. Liu, Ann. N. Y. Acad. Sci., 1998, 860, 333–345 CrossRef CAS PubMed.
- M. Takahashi, M. Narushima and Y. Oda, J. Neurosci., 2002, 22, 3929–3938 CrossRef CAS PubMed.
- D. Clift, H. Richendrfer, R. J. Thorn, R. M. Colwill and R. Creton, Zebrafish, 2014, 11, 455–461 CrossRef PubMed.
- R. Spence, A. E. Magurran and C. Smith, Anim. Cogn., 2011, 14, 607–612 CrossRef PubMed.
- M. A. Wolman, R. A. Jain, L. Liss and M. Granato, Proc. Natl. Acad. Sci. U. S. A., 2011, 108, 15468–15473 CrossRef CAS PubMed.
- M. S. Fanselow, Anim. Learn. Behav., 1990, 18, 264–270 CrossRef.
- J. M. Bailey, A. N. Oliveri and E. D. Levin, Physiol. Behav., 2016, 176, 139–148 Search PubMed.
- L. J. Guerra, A. M. B. do Amaral, V. A. de Quadros, T. da Luz Fiuza, D. B. Rosemberg, O. D. Prestes, R. Zanella, B. Clasen and V. L. Loro, Arch. Environ. Contam. Toxicol., 2021, 81, 255–264 CrossRef CAS PubMed.
- H. Jun, A. Bramian, S. Soma, T. Saito, T. C. Saido and K. M. Igarashi, Neuron, 2020, 107, 1095–1112.e6 CrossRef CAS PubMed.
Footnotes |
† Electronic supplementary information (ESI) available: ESI-text and ESI-supplementary videos. See DOI: https://doi.org/10.1039/d2lc00758d |
‡ These authors contributed equally to this work. |
|
This journal is © The Royal Society of Chemistry 2023 |