Chlorine speciation in complex hydrocarbon matrices using GC-ICP-MS/MS†
Received
2nd March 2023
, Accepted 28th June 2023
First published on 3rd July 2023
Abstract
In this study, the hyphenation of gas chromatography with ICP tandem mass spectrometry (GC-ICP-MS/MS) for the analysis of volatile organochlorides in complex hydrocarbon samples is described. H2 was used as a reaction gas in the MS/MS collision reaction cell in order to bring selectivity and sensitivity towards chlorine detection and the gas flows feeding the plasma were carefully optimized to limit the interferences due to coeluting hydrocarbons. The response towards organochlorides proved to be linear, equimolar against elemental chlorine on a large dynamic range with quantification limits in the range of 30–100 μgCl L−1 per peak. This compound independent calibration (CIC) method was successfully applied to the analysis of light petroleum samples as well as plastic pyrolysis oil light cuts with a good agreement with other total elemental chlorine analysis techniques such as X-ray fluorescence, thus attesting the strength of this technique for the untargeted analysis of organochlorides in complex hydrocarbon volatile samples.
Introduction
Chlorine (Cl) is naturally present in some process feeds (crude oils, plastic waste pyrolysis oils, etc.) or can be intentionally added in some refining units such as in the continuous catalyst regeneration (CCR) reforming unit.1 It can be in the form of inorganic salts (NaCl, MgCl2, CaCl2) or organic species.2 These compounds are responsible for numerous industrial issues including pipe or heat exchanger fouling due to the formation of ammonium chloride (NH4Cl) or green oils;1 poisoning or inhibition effects on catalysts3 and corrosion issues due to the formation of HCl for Cl concentrations as low as 1 mg kg−1.4,5 In conventional oil products such as reformates, organic Cl generally ranges from several μg kg−1 to few mg kg−1 whereas the Cl content in plastic pyrolysis oil is often much higher and ranges from several mg kg−1 to up to several hundreds of mg kg−1. This high Cl content mainly originates from unperfect plastic sorting and traces of polyvinyl chloride (PVC) in the plastic mix used to produce pyrolysis oils.6
Whereas inorganic chlorine can be removed to a certain extent by water extraction processes, the removal of organic chlorine is much more challenging and often requires dedicated processes or catalysts.7 As a consequence, adequate analytical techniques dedicated to chlorine monitoring are required. In 2013, Doyle et al.8 published a review on the total determination of chlorine in crude oil and petroleum derivatives. Several standard methods deal with total chlorine quantification in hydrocarbon samples.9 ASTM D4929 (ref. 10) describes three different procedures for organic chlorine including potentiometric titrations (a), microcoulometric titrations (b) or X-ray fluorescence (XRF) (c) with a limit of quantification (LOQ) above 1 mg kg−1 in washed naphtha. Combustion followed by microcoulometry according to UOP779 (ref. 11) or NF ISO 14077 (ref. 12) with LOQs respectively of 0.3 and 2 mg kg−1 is also used. For total chlorine in aromatics, ASTM D7536 (ref. 13) using monochromatic wavelength dispersive (MWD) XRF is often used with a LOQ of 0.7 mg kg−1. Focusing on inductively coupled plasma mass spectrometry (ICP-MS), Cl detection is still challenging because of Cl high ionization potential (12.97 eV) and the existence of severe polyatomic interferences (O2H+, SH+, ArH+).14 Very recently, Nelson et al.5 developed a mass shift method using tandem mass spectrometry (ICP-MS/MS) with H2 as the cell gas (35Cl → 35Cl1H2+) to quantify total chlorine in crude oils at very low levels. A LOQ of 40 μg kg−1 and a very good agreement with instrumental neutron activation analysis (INAA) data were obtained. However, for all the issues previously mentioned due to the presence of chlorine, the total content may be not sufficient and the monitoring of chlorine species all along the process scheme is often required to get a better insight on the observed phenomena.
Very recently, an isotope dilution headspace GC-MS method was developed to determine inorganic chloride in crude oils with a LOQ of 100 μg kg−1.15 Using mass balance, the contribution of organic chlorine to total Cl could be checked but no details on each chlorine species could be obtained. In the field of organic chlorine speciation for oil-derived samples, only a few studies have been reported. They are mostly based on gas chromatography (GC) for the separation of volatile organochlorides with an electron capture detector (ECD)16,17 or quadrupole time of flight mass spectrometry (QTOF/MS).18 However, none of this solution offers fully satisfactory results for chlorine speciation due to either poor sensitivity, low selectivity, non-universal response towards Cl species or the need of adequate commercially available and often expensive standards to calibrate the method. In 2020, a review focusing on the recent advances in GC-ICP-MS demonstrated the potential of GC-ICP-MS/MS for the speciation of some challenging elements (P, S, Si and Cl).14 Somoano-Blanco et al.19 and Nelson et al.20 developed a mass shift GC-ICP-MS/MS method using H2 for Cl-pesticides in vegetable based food and polychlorinated biphenyls in environmental samples respectively. In petroleum derivatives, S and Si speciation has been reported in light petroleum products using GC-ICP-MS/MS with a compound independent calibration (CIC) and a good mass balance regarding total content.21,22 However, no Cl speciation method based on GC-ICP-MS/MS for complex hydrocarbon matrices has been reported so far.
The present study aims at developing a GC-ICP-MS/MS method for Cl speciation in complex hydrocarbon matrices such as gasoline type samples or plastic pyrolysis oils. Using different matrices and commercially available chlorinated standards, GC-ICP-MS/MS parameters were carefully optimized to minimize the impact of the hydrocarbon matrix and lead to a CIC method. The features of the resulting analytical technique such as limits of quantification are presented as well as some representative analytical results obtained on real petroleum derivatives such as reformates or plastic pyrolysis gasoline cuts.
Experimental
Standards and samples
Chlorinated compounds used as standards were selected according to the boiling point range of light petroleum cuts and based on the previous identification of chlorinated compounds in naphtha by GC-ECD.17 These compounds were purchased from Sigma-Aldrich (L’Isle d’Abeau, France) or Interchim (Montluçon, France) with purities above 98%. Their raw formulas, chlorine percentage, boiling points, and retention times under the optimized operating conditions are reported in ESI (Table S1†). Calibration solutions were obtained by dilution in n-heptane (Normapur, VWR, Fontenay sous Bois, France) or real gasoline type liquids (G1 to G4) used as solvents. G1 is a commercial RON 98 gasoline purchased in Lyon (France) in summer 2018, whereas the reformate (G2), the fluid catalytic cracking gasoline (G3) and the hydrotreated FCC gasoline (G4) were provided by IFP Energies Nouvelles (Solaize, France). 4 real samples analyzed are a reformate from a CCR reforming unit and 3 plastic pyrolysis oil light cuts (A, B and C) provided by IFP Energies Nouvelles (Solaize, France). No internal standard was used to spike the samples prior to analysis.
Instrumentation
GC-ICP-MS/MS.
All experiments were carried out using an Agilent 7890B GC coupled to an Agilent 8800 ICP-MS/MS instrument using an Agilent GC-ICP-MS transfer line (Agilent Technologies, Japan). GC separation was performed on a HP-INNOWax column (60 m, 0.25 mm, 0.5 μm – Agilent JW, USA) using He as the carrier gas and split injection. The GC column outlet was connected via a T piece to a pre-heating line located inside the GC oven to mix the GC carrier gas flow with other gases. These gases are a mix of argon (carrier gas – CG) coming from the mass flow controller of the ICP-MS/MS and an Ar/O2 mixture (80/20 v/v) named optional gas (OG). CG is used to sweep the GC column effluents through the transfer line into the plasma while OG is added to the CG to burn off carbon deposits coming from the samples. More details about the GC-ICP-MS interface are available in our previous paper focusing on Si speciation.22 All the gases used for the ICP-MS/MS met Agilent specifications. H2 as the reaction gas was purchased from Linde (5.0 grade, purity > 99.999%). A mixture of Xe at 0.1% in He (Air liquide, France) was used as the tune gas to make the torch alignment of the GC-ICP-MS/MS (torch axis) to get the maximum sensitivity on the 124Xe → 124Xe m/z value. ICP-MS/MS lens tuning was also performed on 38Ar → 39ArH+ masses due to their proximity to the 35Cl → 35Cl1H2+ transition of interest. The optimized ICP-MS/MS operating conditions used in this study are summarized in Table 1. GC-ICP-MS/MS data acquisition and analysis were performed with Agilent MassHunter 4.2 software. An Agile2 integrator was used for the automatic integration of peaks and the minimum height peak value was set to 100 counts.
Table 1 Operating conditions of the GC-ICP-MS/MS system
GC conditions |
Injection |
1 μL in split 1 : 10 mode at 250 °C |
Carrier gas |
He at 5 mL min−1 |
Column |
HP-INNOWax (60 m, 0.25 mm, 0.5 μm) |
Oven program temperature |
40 °C (5 min) → 250 °C at 10 °C min−1 or isothermal at 100 °C |
Transfer line temp. Injector temp |
300 °C |
300 °C |
ICP-MS/MS detection conditions |
Cones type |
Pt cones |
Sample depth |
4 mm |
RF power |
1550 W |
Carrier gas flow (CG) |
Ar at 0.10 L min−1 |
Optional gas flow (OG) |
Ar/O2 (80/20) at 0.16 L min−1 |
Cell gas flow |
H2 at 1 mL min−1 |
Isotopes monitored (dwell time) Q1 → Q2 |
35Cl → 37ClH2+ (0.5 s) |
13C → 13C + (0.01 s) |
38Ar → 39ArH + (0.02 s) |
XRF measurements.
The total Cl content of plastic pyrolysis oils was determined according to an internal IFPEN method.23 A 4 kW Axios (Panalytical, Almelo, Netherlands) equipped with a Cr anode was used to perform the wavelength dispersive X-ray fluorescence (WDXRF) analysis. A minimum of 4 mL of solution was introduced in a cup equipped with a Mylar 6 μm film. The reported result is the mean of two independent measurements on two different cups.
Results and discussion
GC-ICP-MS/MS for chlorine speciation analysis aims at separating volatile organochlorides prior to their selective detection by ICP-MS/MS. In complex samples such as gasolines or plastic pyrolysis oils, it is often not possible to isolate Cl-containing compounds from hydrocarbons that are present in much higher amounts and almost induce a continuous flow of carbon reaching the plasma. Thus, it is of utmost importance to set operating parameters that minimize the effect of hydrocarbons on the chlorine detection while maintaining enough sensitivity.
Optimization of the GC-ICP-MS/MS operating conditions
In order to study the influence of carbon on the Cl response, the employed methodology consisted of injecting 2 chlorinated standard compounds, one of them being coeluted with a hydrocarbon. Chlorinated compounds as well as separation conditions were chosen to obtain fast analysis time during the optimization phase and to maximise the coelution between one of the chlorinated compounds and a hydrocarbon solvent. A solution called “optimization mix” containing 2-chloro-2-methylpropane and CHCl3 with concentrations of approximately 2 mg kg−1 of Cl per peak in n-heptane was prepared and injected on a polar HP-INNOWAX column using an isothermal GC separation at 100 °C and a carrier gas flow rate of 5 mL min−1. Under these conditions, the n-heptane solvent peak and 2-chloro-2-methylpropane co-eluted at 2.05 min whereas CHCl3 is eluted at 3.07 min. The GC injection conditions, torch sample depth (4 mm) and dwell times were set so as to promote sensitivity. All along the separation, the intensities of three transitions were monitored using H2 as a reaction gas in the collision cell: 35Cl → 37ClH2+, 13C → 13C+ and 38Ar → 39ArH+. The chlorine channel (noted 35→37Cl) allowed selective detection of chlorinated compounds, the carbon channel (noted 13→13C) allowed monitoring of the elution of hydrocarbons while the argon channel (noted 38→39Ar) gave an insight on the plasma response. The H2 cell gas flow rate was set to 1 mL min−1 as it was the lowest applicable value on the instrument. Higher tested H2 flow rates (up to 3 mL min−1) resulted in a much lower response for chlorine due to important ion losses in the reaction cell. This differs from other studies which report higher optimum values between 3 and 4.6 mL min−1 for chlorine detection.5,24 This discrepancy is not fully explained but it may be attributed to impurity traces in the H2 reaction gas even if the gas specifications for the instrument were met.
The optimization mix was injected under different plasma conditions with carrier (CG) and option gas (OG) flow rates ranging from 0.1 to 0.2 L min−1 for CG and 0.12 to 0.28 L min−1 for OG (Table 1). On the chlorine channel, the peak areas and heights for 2-chloro-2-methylpropane and CHCl3 were measured as well as the noise in the 0–0.9 min time interval. The sensitivity of the method was evaluated through the measurement of the signal to noise (S/N) ratio of the non-coeluted CHCl3 peak. As illustrated in Fig. 1 for CG = 0.2 L min−1 and OG = 0.24 L min−1, the elution of n-heptane results in a sharp intense peak on the carbon channel together with a negative peak on the argon channel. This negative peak can be related to a kind of plasma destabilization upon n-heptane arrival in the plasma. A criterion describing the plasma sensitivity towards carbon was defined as the ratio between the negative peak area A with the plasma mean intensity H.
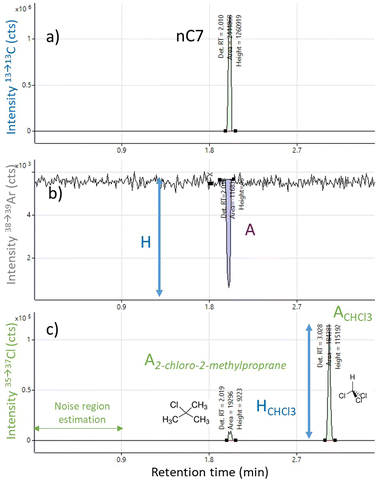 |
| Fig. 1 Chromatograms of the (a) carbon 13→13C, (b) argon 38→39Ar, and (c) chlorine 35→37Cl channels obtained for the injection of the optimization mix solution containing CHCl3 and 2-chloro-2-methylpropane in n-heptane with CG = 0.2 and OG = 0.24 L min−1 (maximum Cl sensitivity conditions). | |
Finally, the influence of carbon on chlorine detection was estimated by calculating the ratio between the 2-chloro-2-methylpropane peak area and the CHCl3 peak area. The lower is this ratio, the highest is the area of the solvent interfered peak compared to the non-interfered one. For the same quantity of chlorine in the two compounds, the ratio should be close to 1.
For all tested CG and OG gas flow rates, these 3 criteria were calculated and the surface response was plotted against CG and OG values (Fig. 2). The S/N ratio on CHCl3 depicts the ability of the analytical technique to detect non-coeluted chlorinated compounds in the absence of hydrocarbons. It is maximized for CG and OG values of 0.2 L min−1 and 0.24 L min−1 respectively. However, in this region, the plasma is very sensitive to carbon which is reflected by the high influence of carbon on both plasma and Cl detection. Under these conditions, as seen in Fig. 1, 2-chloro-2-methylpropane coeluted with n-heptane is barely detected and strong interferences from coeluting hydrocarbons present in real samples are expected. In contrast, operating conditions around CG = 0.1 L min−1 and OG = 0.16 L min−1 provide both satisfactory Cl sensitivity and very low influence of carbon on Cl detection and on plasma. No negative peak is observed when the solvent arrives at the detector and the area of the 2-chloro-2-methylpropane peak is close to its maximum (see Fig. S1 in ESI†). These conditions were considered as the optimal ones with the objective of developing the most robust method possible with minimum interferences from hydrocarbons. These optimized parameters were also found to be repeatable from one analytical campaign to the other one for several months and were used for the subsequent part of the study.
 |
| Fig. 2 (a) CHCl3 S/N ratio; (b) C influence on plasma and (c) C influence on Cl for different CG and OG gas flow rates. Red dots correspond to experimental values tested. Blue dots refer to Cl maximum sensitivity conditions (CG = 0.2 L min; OG = 0.24 L min−1) and optimized conditions (CG = 0.1 L min; OG = 0.16 L min−1). | |
GC-ICP-MS/MS as a compound independent calibration method (CIC) for chlorine speciation analysis
CIC methods are attractive methods since they do not require specific and often expensive, not stable, or not commercially available standards.14 A CIC method for chlorine speciation requires that quantification is not affected by the chemical structure of the detected compound but also that matrix effects are negligible.
Influence of the chemical structure of chlorinated species
To study the influence of the chemical structure of chlorinated species under the previously described optimized conditions, the response of 13 chlorinated standard compounds was first studied in n-heptane. These 13 standard compounds span a large variety of chemical functions as they contain one or several Cl atoms linked to alkyl chains, double bonds or aromatic rings and possess boiling points from 40 °C (dichloromethane) to 159 °C (1-chloroheptane). GC separation was performed on a polar HP-INNOWax column with an initial isothermal plateau at 40 °C during 5 min followed by an oven temperature ramp at 10 °C min−1 until 250 °C (Table 1). These conditions demonstrated a better separation for light volatile chlorinated compounds compared to traditionally used polydimethylsiloxane based apolar columns such as DB-5ms. Regarding injection conditions, the split ratio and helium carrier gas flow were set to 10
:
1 and 5 mL min−1 and 1 μL of sample was injected. This corresponds to a 54 cm s−1 average linear velocity, quite high compared to the optimal linear velocity according to Van Deemter diagrams for helium (20–40 cm s−1). However, this higher flow rate allowed the use of high split flows in the inlet (50 mL min−1) with low split ratios (1
:
10), resulting in narrower peaks for early eluting peaks. Lower split ratios or larger injection volume did not significantly improve Cl detection limits as broader peaks were obtained.
Calibration standards in n-heptane were injected in triplicate for concentrations between 0.02 and 15 mgCl L−1 per compound. Calibration lines with a linear y = a × x model are reported in Fig. S2 and S3.† All the compounds exhibited a linear response on the entire calibration dynamic range (from LOQ to around 10 to 15 mgCl L−1) with correlation coefficients above 0.999. Moreover, five independent calibrations in n-heptane were prepared and relative response factors, defined by the ratio between the slope of each compound calibration line and the one for 1,2-dichloroethane (Fig. S4†) taken as the reference, were measured (RRF1,2-dichloroethane = 1 by definition). The mean RRFs towards elemental chlorine reported in Table 2 were all comprised between 0.97 and 1.05 regardless the chemical structure of the quantified organochloride, demonstrating the ability of the designed GC-ICP-MS/MS method to give an equimolar response. Reproducibility was below 10% relative for all the standards except dichloromethane (12%) but this slightly higher reproducibility can be explained by dichloromethane high volatility and the inherent difficulty to prepare standards with accurate concentrations in the ppm range.
Table 2 Relative response factors versus 1,2-dichloroethane towards elemental chlorine for 13 chlorinated standard compounds under optimized GC-ICP-MS/MS conditions
Compound |
RRF/1,2-dichloroethanea |
5 replicates were taken into account.
|
2-Chloro-2-methylpropane |
1.05 ± 0.04 |
1-Chloropropane |
1.01 ± 0.05 |
1-Chlorobutane |
1.01 ± 0.03 |
Carbon tetrachloride |
1.00 ± 0.02 |
1-Chloro-2-methylbutane |
0.99 ± 0.02 |
Dichloromethane |
0.97 ± 0.12 |
1-Chloropentane |
1.00 ± 0.06 |
Trichloroethylene |
1.00 ± 0.05 |
Tetrachloroethylene |
1.01 ± 0.07 |
1,2-Dichloroethane |
1.00 ± 0.02 |
1-Chloroheptane |
1.02 ± 0.07 |
Chlorobenzene |
1.02 ± 0.06 |
1,1,2-Trichloroethane |
1.01 ± 0.04 |
Taking 1,2-dichloroethane calibration line as a reference for the quantification of all 13 injected organochlorides, limits of quantification (LOQ) expressed in μgCl L−1 were evaluated for each model compound.
The quantification limit was defined as the lowest concentration for which calculated recoveries from three replicate injections were comprised between 80% and 120%. Obtained LOQs in n-heptane are reported in Table 3. Except for dichloromethane, LOQs were below 100 μgCl L−1 with values as low as around 30 μgCl L−1 for the late eluting chlorinated compounds which gave sharper peaks. This is in good agreement with reported values from Nelson et al.5 for petroleum fractions.
Table 3 Limits of quantification for 13 chlorinated standard compounds in n-heptane under optimized conditions (1,2-dichloroethane used as the calibrant)
Compound |
LOQ (μgCl L−1) |
Calculated conc. (μgCl L−1) |
Recovery (%) |
2-Chloro-2-methylpropane |
76 |
70 |
93% |
1-Chloropropane |
94 |
97 |
103% |
1-Chlorobutane |
103 |
103 |
100% |
Carbon tetrachloride |
90 |
102 |
113% |
1-Chloro-2-methylbutane |
72 |
59 |
81% |
Dichloromethane |
206 |
206 |
100% |
1-Chloropentane |
85 |
89 |
104% |
Trichloroethylene |
99 |
100 |
101% |
Tetrachloroethylene |
92 |
104 |
113% |
1,2-Dichloroethane |
88 |
93 |
105% |
1-Chloroheptane |
34 |
33 |
95% |
Chlorobenzene |
32 |
37 |
117% |
1,1,2-Trichloroethane |
36 |
41 |
114% |
Evaluation of matrix effects
To study the interferences of coeluting hydrocarbons, a set of 4 other calibrations was prepared by spiking 4 chlorine-free gasoline samples ([Cl]total < 0.3 mg L−1 by UOP779) with 13 organochlorides to reach concentrations in the range of 0.02–15 mgCl L−1 per compound. The used gasoline samples originated from different refining processes (reforming, hydrodesulfurization, FCC, commercial blend) and exhibited different chemical compositions. Fig. 3 presents the obtained chromatograms on the chlorine (35→37Cl in green), carbon (13→13C in blue) and argon (38→39Ar in grey) channels for a spiked commercial gasoline with concentrations close to 100 μgCl L−1 per compound under the optimized GC-ICP-MS/MS conditions. On the chlorine channel, well resolved peaks were obtained except for CCl4, 1-chloro-2-methylbutane and tetrachloroethylene for which the peak shape was not fully Gaussian. On the argon channel, the signal intensity was very stable even when high hydrocarbon amounts exited the GC column. Finally, the carbon channel illustrates the complexity of the gasoline matrix (more than 200 compounds) and strong coelution zones for example between hydrocarbons and 1-chloroheptane or 2-chloro-2-methylpropane were observed. However, linear calibration slopes were obtained for all gasolines and all compounds on the entire investigated dynamic range. Moreover, measured RRFs versus 1,2-dichloroethane in n-heptane for all gasoline matrices and chlorinated compounds were close to 1.00 ± 0.10 with values ranging between 0.83 and 1.04 except for dichloromethane (Fig. 4 and Table S2†). Slightly lower RRFs were measured for dichloromethane (RRF = 0.76–0.84) most probably due to its high volatility. The small decreasing trend of the RRFs between G1 and G4 can be attributed to a drift of ICP-MS/MS sensitivity as the total experimental time to obtain these data was superior to 30 hours during which no recalibration was done. It is interesting to mention that similar responses were obtained on the INNOWax column where separation of organochlorides was fairly good and on a DB-1MS column (23.5 m, 0.25 mm, 0.25 μm) run with the same injection conditions and the same GC carrier gas flow rate regardless the GC separating conditions (isothermal at 35 °C or 340 °C). Under these latter conditions, organochlorides and n-heptane were eluted at the same time in less than 0.1 min (see Fig. S5–S7†). However, no significant influence of this large hydrocarbon quantity reaching the plasma within a very short time was noticed on chlorine response, attesting very low matrix effect even in the case of severe coelution with hydrocarbons.
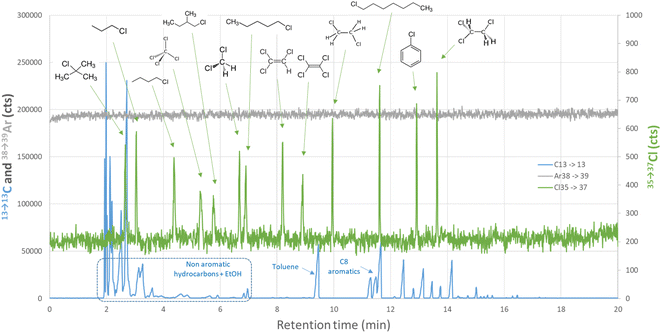 |
| Fig. 3 GC-ICP-MS/MS chromatograms under optimized conditions for the G1 gasoline spiked with 13 chlorinated compounds at around 100 μgCl L−1 per compound. Transitions monitored: 13→13C in blue, 38→39Ar in grey and 35→37Cl in green. | |
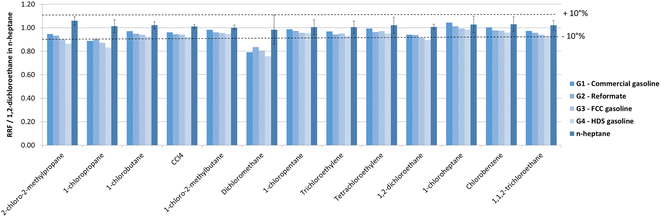 |
| Fig. 4 Relative response factor versus 1,2-dichloroethane (RRF) in n-heptane for 13 chlorinated compounds in 4 different gasolines (G1–G4) and in n-heptane. | |
As relative response factors towards 1,2-dichloroethane were close to 1 for any of the 13 chlorinated compounds in any tested hydrocarbon matrix, compound independent calibration method requirements were fulfilled. Consequently, the calibration curve of 1,2-dichloroethane in n-heptane can be used to obtain quantitative results for any detected peak under these GC-ICP-MS/MS conditions in any type of sample. Recovery values calculated by this way for 13 compounds spiked in 4 gasoline samples with concentrations around 200 μgCl L−1 per peak are reported in Table 4. Satisfactory recoveries (80–120%) were obtained for most of the chlorinated compounds. Similar results were obtained in the middle and the upper part of the calibration range (see Table S3 in the ESI†), thus demonstrating the ability of the developed method to accurately quantify organochlorides from several hundreds of ppb to ppm levels in any complex hydrocarbon matrices with a single compound calibration.
Table 4 Percentage of recovery for 13 chlorinated compounds spiked in 4 gasoline samples for concentrations close to 0.2 mgCl L−1
Compound |
[Cl] mgCl L−1 |
G1 |
G2 |
G3 |
G4 |
2-Chloro-2-methylpropane |
0.18 |
135% |
103% |
89% |
92% |
1-Chloropropane |
0.23 |
112% |
90% |
91% |
75% |
1-Chlorobutane |
0.25 |
111% |
93% |
93% |
96% |
Carbon tetrachloride |
0.22 |
103% |
101% |
94% |
96% |
1-Chloro-2-methylbutane |
0.18 |
100% |
96% |
92% |
95% |
Dichloromethane |
0.20 |
106% |
85% |
78% |
81% |
1-Chloropentane |
0.21 |
117% |
99% |
88% |
98% |
Trichloroethylene |
0.24 |
103% |
98% |
103% |
93% |
Tetrachloroethylene |
0.22 |
110% |
103% |
93% |
97% |
1,2-Dichloroethane |
0.21 |
118% |
89% |
95% |
93% |
1-Chloroheptane |
0.24 |
107% |
105% |
107% |
99% |
Chlorobenzene |
0.23 |
105% |
98% |
101% |
99% |
1,1,2-Trichloroethane |
0.25 |
99% |
95% |
98% |
93% |
Cl speciation in real samples with GC-ICP-MS/MS
The GC-ICP-MS/MS method was applied to study the speciation of organochlorides in 4 samples: one reformate and 3 pyrolysis plastic oil light cuts. The chromatograms are shown in Fig. 5. Quantification was performed using 1,2-dichloroethane in n-heptane as the calibrant.
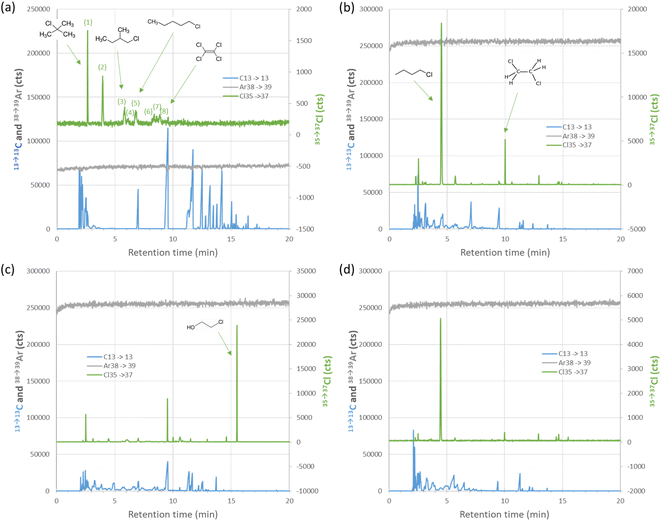 |
| Fig. 5 GC-ICP-MS/MS chromatograms under optimized conditions for (a) a reformate sample ([Cl] = 2.8 mg kg−1); (b) pyoil gasoline A ([Cl] = 58 mg kg−1); (c) pyoil gasoline B ([Cl] = 52 mg kg−1) and (d) pyoil gasoline C ([Cl] = 15 mg kg−1). Transitions monitored: carbon (13→13C) in blue, argon (38→39Ar) in grey and chlorine (35→37Cl) in green. | |
Reformate.
In the reformate sample, 8 chlorinated compounds were detected and quantified by GC-ICP-MS/MS (Fig. 5a). Four of these species were identified by retention time matching with standards: 2-chloro-2-methylbutane (1), 2-chloro-2-methylpropane (3), 1-chloropentane (5) and tetrachloroethylene (8) which was already identified in naphtha obtained from crude oil by Wu et al.17 by GC-ECD. Quantification of all chlorinated species including unknowns is reported in Table S4.† 2-Chloro-2-methylpropane and compound 2 are the main compounds and each accounts for approximately 30% of the total chlorine content. Compound 2 elutes between C3 and C4 linear chloroparaffin but after highly branched 2-chloro-2-methylpropane. As the elution of chlorinated species is driven by polarity with the INNOWax column and knowing that HCl reacts with olefins present in the reformate to form chlorinated species,2 compound 2 could be a branched C5 chloroparaffin. 1-Chloro-2-methylbutane (3), 1-chloropentane (5) and tetrachloroethylene (8) represent respectively around 15, 12 and 5% of the total chlorine content. The total quantity of the 3 other unknown compounds corresponded to around 10.6% of the total Cl content detected by GC-ICP-MS/MS. To validate the accuracy of the developed method, the sum of all peaks quantified by GC-ICP-MS/MS was compared to the total Cl content determined by other analytical techniques (Table 5). A 86% overall recovery was found for the reformate sample despite the quite low total Cl concentration (2.8 mg kg−1).
Table 5 Chlorine content quantified by GC-ICP-MS/MS (sum of peaks) on 4 different samples and comparison with other analytical techniques
Samples |
Density (g mL−1) |
[Cl] by XRF (mg kg−1) |
GC-ICP-MS/MS sum of peaks (mg kg−1) |
Cl recovery (%) |
Value determined according to ASTM D4929.
|
Reformate |
0.8106 |
2.8a |
2.4 ± 0.2 |
86 |
Pyoil A |
0.8034 |
58 ± 3 |
55 ± 5 |
95 |
Pyoil B |
0.7951 |
52 ± 3 |
52 ± 5 |
100 |
Pyoil C |
0.7277 |
15 ± 2 |
13 ± 2 |
88 |
Plastic pyrolysis oils.
3 different plastics pyrolysis oil (pyoil) gasoline cuts (A, B and C) with total chlorine contents between 15 and 58 mg kg−1 were analysed with the optimized GC-ICP-MS/MS method. The obtained chromatograms (Fig. 5b–d) show multiple peaks, some of them eluting at the same retention time as chlorinated standard compounds. 1-Chlorobutane and 1,2-dichloroethane were identified and quantified in pyoil A in relatively large amounts, while a dozen of other minor peaks were detected. The pyoil B chromatogram is characterized by a completely different speciation compared to the other two samples with an intense peak at 15.5 min. This compound has been further identified as 2-chloroethanol by GC × GC-MS experiments. Regarding pyoil C, it has shown a Cl profile close to pyoil A with 1-chlorobutane as the major constituent. Contrary to expected mechanisms of PVC pyrolysis,25 detected amounts of chlorobenzene at 12.9 min compared to other chlorinated compounds were very low. As for the reformate sample, the sum of all detected peaks for each sample was in good agreement with total chlorine measured by XRF with Cl recovery values between 88% and 100%. Finally, it is noteworthy that there are strong differences in the type and the distribution of chlorinated species present in plastics pyrolysis oils even for pyoils with comparable total Cl content. The proposed GC-ICP-MS/MS method enables thus to understand why two different pyoils may have different reactivities in a purification process for example and therefore is a powerful tool for process development and understanding. Even without proper identification, the CIC method provides quantification for each chlorinated species. It also allows identification of non-reactive or refractory chlorinated species along the process and is useful to direct the identification efforts towards these specific molecules.
Conclusions
The presence of chlorine is always a challenge in process units because of the serious problems caused such as corrosion issues. Thus, the aim of this work was to propose a speciation method for chlorine at trace levels in complex organic matrices. A GC-ICP-MS/MS instrumental setup has been carefully optimized for chlorine speciation in organic matrices in order to obtain a compound independent calibration analytical method with 1,2-dichloroethane used as an external calibrant in n-heptane. Limits of quantification between 30 and 100 μgCl L−1 were reached for numerous organochlorides in n-heptane with a linear, equimolar response and negligible matrix effects in the 0.1–15 mgCl L−1 per peak range. This method was successfully applied to varied complex organic samples including plastic pyrolysis oil gasoline cuts. In these samples, a very good agreement was obtained on the chlorine mass balance between the speciation results and the total content. GC-ICP-MS/MS thus proves to be complementary to other existing chlorine speciation analytical methods such as GC-ECD as it allies equimolar response, ease of calibration, selectivity towards chlorine and good sensitivity. Further work should be oriented to the applicability of the method on heavier organochlorides present in heavy cuts and the structural identification of detected compounds with complementary methods such as GC-HRMS or GC × GC-MS.
Author contributions
Vincent Souchon: conceptualization, data curation, formal analysis, investigation, methodology, supervision, writing – original draft, writing – review & editing; Marc Maleval: data curation, formal analysis, investigation, methodology; Fabien Chainet: conceptualization, data curation, formal analysis, investigation, methodology, writing – original draft, writing – review & editing; Charles-Philippe Lienemann: conceptualization, methodology, supervision, writing – review & editing.
Conflicts of interest
There are no conflicts to declare.
Acknowledgements
The authors wish to thank L. Ayouni and F. Bessueille (Université de Lyon, Institut des Sciences Analytiques, UMR 5280, CNRS, Université Lyon 1, ENS Lyon – 5, rue de la Doua, F-69100 Villeurbanne, France) for the access to the ICP-MS/MS instrument.
Notes and references
- O. K. Karan, M. A. Ay, K. Karhaman and A. Selmen, Petrol. Technol. Q., 2013, Q3, 29–33 Search PubMed.
- E. Pagliano, Z. Gajdosechova, F. Lopez-Linares and Z. Mester, Energy Fuels, 2021, 35, 894–897 CrossRef CAS.
- B. Didillon, J. Cosyns, C. Cameron, D. Uzio, P. Sarrazin and J. P. Boitaux, Stud. Surf. Sci. Catal., 1997, 111, 447–454 CrossRef CAS.
-
C. A. Shargay, NACE International Task Group 274, NACE International Publication 34105, 2005 Search PubMed.
- J. Nelson, L. Poirier and F. Lopez-Linares, J. Anal. At. Spectrom., 2019, 34, 1433–1438 RSC.
- M. Kusenberg, A. Eschenbacher, M. R. Djokic, A. Zayoud, K. Ragaert, S. de Meester and K. M. van Geem, Waste Manage., 2022, 138, 83–115 CrossRef CAS PubMed.
- M. Erfan, Petrol. Technol. Q. Catal., 2011, 1–10 Search PubMed.
- A. Doyle, A. Saavedra, M. L. Tristao, L. A. N. Mendes and R. Q. Aucélio, Spectrochim. Acta, Part B, 2013, 86, 102–107 CrossRef CAS.
- M. Davinder, Petrol. Technol. Q., 2020, Q1, 95–99 Search PubMed.
-
ASTM D4929-22, Standard Test Method for Determination of Organic Chloride Content in Crude Oil, ASTM International, West Conshohocken, PA, 2022, Available at: https://www.astm.org/Standards/D4929.htm Search PubMed.
-
UOP779, Chloride in Petroleum Distillates by Microcoulometry, UOP, 2008, Available at: https://www.astm.org/Standards/D4929.htm Search PubMed.
-
NF EN 14077, Petroleum Products — Determination of Organic Halogen Content — Oxidative Microcoulometric Method, EN, 2004, Available at: https://www.en-standard.eu/csn-en-14077-petroleum-products-determination-of-organic-halogen-content-oxidative-microcoulometric-method/ Search PubMed.
-
ASTM D7536-20, Standard Test Method for Chlorine in Aromatics by Monochromatic Wavelength Dispersive X-Ray Fluorescence Spectrometry, ASTM International, West Conshohocken, PA, 2020, Available at: https://www.astm.org/Standards/D7536.htm Search PubMed.
- J. García-Bellido, L. Freije-Carrelo, M. Moldovan and J. R. Encinar, TrAC Trends Anal. Chem., 2020, 130, 115963 CrossRef.
- Z. Gajdosechova, M. Dutta, F. Lopez-Linares, P. de Azevedo Mello, G. Dineck Iop, E. M. Moraes Flores, Z. Mester and E. Pagliano, Fuel, 2021, 285, 119167 CrossRef CAS.
- X. J. Fan, J. H. Zhu, H. F. Song and B. C. Wu, Pet. Sci. Technol., 2011, 29, 867 CrossRef CAS.
- B. Wu, Y. Li, X. Li and J. Zhu, Energy Fuels, 2015, 29, 1391–1396 CrossRef CAS.
-
F. David, B. Tienpont, P. Sandra, F. Chainet, M. Thomas, E. Lemaire and S. Nieto, Agilent Application Note 5991-5684EN, 2015 Search PubMed.
- L. Somoano-Blanco, P. Rodríguez-González, D. Pröfrock, A. Prange and J. I. García Alonso, Anal. Methods, 2015, 7, 9068–9075 RSC.
- J. Nelson, H. Hopfer, F. Silva, S. Wilbur, J. Chen, K. Shiota Ozawa and P. L. Wylie, J. Agric. Food Chem., 2015, 63, 4478–4483 CrossRef CAS PubMed.
- L. Freije-Carrelo, J. García-Bellido, F. Calderón-Celis, M. Moldovan and J. R. Encinar, Anal. Chem., 2019, 91, 7019–7024 CrossRef CAS PubMed.
- R. Sánchez, F. Chainet, V. Souchon, S. Carbonneaux, C.-P. Lienemann and J.-L. Todolí, J. Anal. At. Spectrom., 2020, 35, 2387–2394 RSC.
-
IFPEN method 2218, Determination of Total Chlorine Content in Petroleum Products Using WDXRF, 2011 Search PubMed.
- B. Lajin and W. Goessler, Anal. Chim. Acta, 2020, 1094, 11–17 CrossRef CAS PubMed.
- J. Yang, Y. Wu, J. Zhu, H. Yang, Y. Li, L. Jin and H. Hu, Fuel, 2023, 331, 125994 CrossRef CAS.
|
This journal is © The Royal Society of Chemistry 2023 |
Click here to see how this site uses Cookies. View our privacy policy here.