DOI:
10.1039/D3IM00071K
(Minireview)
Ind. Chem. Mater., 2023,
1, 475-485
Cutting-edge methods for amplifying the oxygen evolution reaction during seawater electrolysis: a brief synopsis
Received
29th June 2023
, Accepted 10th August 2023
First published on 17th August 2023
Abstract
Electrochemical water splitting has been considered a clean and continual way for hydrogen (H2) production. Direct seawater electrolysis is a potentially attractive technology due to the ample access to seawater and scarce freshwater resources in some regions. However, the presence of impurities (e.g., Cl−, Mg2+) and the resulting corrosion and side reactions, such as the chloride oxidation reaction (ClOR), makes seawater electrocatalysis more challenging than that of fresh or alkaline water due to competition with the oxygen evolution reaction (OER) at the anode. Consequently, much effort has been devoted to developing approaches to enhance OER performance and suppress the ClOR. In this minireview, we summarize three general strategies for enhancing OER activity and selectivity in seawater electrolysis based on three different concepts: (1) the sole development of robust and high-performance OER catalysts in pure seawater electrolytes, (2) the introduction of additives to seawater electrolytes (e.g., alkalis and/or salts without chloride) to enhance the potential equilibrium gap between the ClOR and OER in combination with regular highly active OER catalysts, and (3) a combination of approaches (1) and (2). Finally, the current challenges and potential opportunities for green H2 production from seawater electrolysis are briefly presented.
Keywords: Electrochemical seawater splitting; Alkaline seawater electrolysis; Oxygen evolution reaction; Hydrogen production; Electrocatalysts.
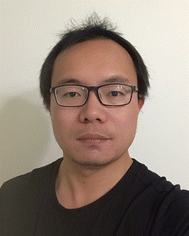 Xiang Lyu | Dr. Lyu joined the ORNL in 2021 as a postdoc research associate. Currently, he dedicates his research to PEMFCs, electrolysis in acidic/alkaline/seawater media, and CO2 reduction. He received his Ph.D. Degree in Chemical Engineering in 2020 from Ohio University, where he mainly worked on water electrolysis, fuel cells, and catalyst design and synthesis. |
1 Introduction
The growth of the global energy crisis, along with environmental pollution caused by fossil fuels, has strongly driven the pursuit of renewable energy sources.1–4 Green hydrogen (H2), produced from water electrolysis using renewable energy sources (e.g., wind, solar, and tide), accomplished with high-efficiency fuel cells,5–7 has been recognized as a promising alternative to fossil fuels because of its high energy density and free pollution.8–15 Water distribution issues may arise if vast amounts of purified water are used for water splitting to mitigate the global energy crisis.16,17 Thus, seawater is preferred to freshwater due to its high abundance on the earth compared to freshwater sources.18–20
In electrochemical water splitting, the oxygen evolution reaction (OER) at the anode – a four-electron transfer reaction – is more kinetically sluggish than the hydrogen evolution reaction (HER) at the cathode – a two-electron transfer reaction.21,22 Consequently, the OER determines the overall efficiency of water splitting.23,24 Additionally, the reactions at the anode in seawater media are more complex because of the presence of metal chlorides. Catalysts can be severely corroded by chlorine gas (Cl2, in acidic media) or hypochlorite (ClO−, in alkaline media) formed from the chloride oxidation reaction (ClOR), resulting in low electrochemical activity and poor durability.19,25–29 Therefore, the ClOR must be suppressed in electrochemical seawater splitting. The reactions at the anode in seawater splitting can be represented based on different electrolytes as follows:
Eqn (1)–(3) in acidic media:
| OER: 2H2O → O2 + 4H+ + 4e− E0 = 1.23 V vs. RHE | (1) |
| ClOR: 2Cl− → Cl2 + 2e− E0 = +1.36 V vs. RHE | (2) |
Eqn (3) and
(4) in alkaline media:
| OER: 4OH− → O2 + 2H2O + 4e− E0 = +1.23 V vs. RHE | (3) |
| ClOR: Cl− + 2OH− → ClO− + H2O + 2e−E0 = +1.72 V vs. RHE | (4) |
According to the thermodynamic potentials of
eqn (1)–(4), the selectivity for the OER and ClOR is dependent on the pH and chloride concentration. The OER equilibrium potential is only 130 mV higher than that of the ClOR in acidic media, while the ClOR possesses a kinetic advantage compared to the OER due to its two-electron transfer reaction, resulting in a considerable challenge for achieving high selectivity to the OER, especially at high current density and potential.
30 In contrast, alkaline seawater electrolysis is thermodynamically more favorable for the OER than the ClOR, with a potential difference of 490 mV, which is desirable for suppressing the ClOR. The corrosion of catalysts and substrates may still be a concern even with highly active OER catalysts in alkaline media, as the aggressive chloride ions can corrode
via metal chloride–hydroxide formation mechanisms.
31 Consequently, various strategies have been devoted to enhancing the OER and simultaneously suppressing the ClOR during seawater splitting in the past decade.
According to a literature review,32–38 approaches for enhancing OER selectivity in seawater splitting can be roughly categorized into three types: (1) solely developing catalysts with high OER activity and selectivity in pure seawater electrolytes (natural or simulated seawater), (2) the introduction of additives to seawater electrolytes (e.g., alkalis and/or salts without chloride) combined with regular highly active OER catalysts, where the OER becomes thermodynamically favorable, and (3) the combination of catalyst development and electrolyte preference, as described in types (1) and (2). In this minireview, we emphasize the three general types that have guided critical developments in seawater electrolysis for H2 production and their implications for future research. Two examples are given for each type of approach. Ultimately, we summarize the challenges and give perspectives on the seawater electrolysis strategies, which could be the potential direction to speed up seawater splitting for green H2 production.
2 Approaches for enhancing OER selectivity in seawater splitting
2.1 Solely developing robust catalysts with high OER activity and selectivity
The sole development of robust catalysts with high OER activity and selectivity is an ideal strategy for direct pure seawater electrolysis, although the requirement for this kind of catalyst to survive in pure seawater is super critical, especially at high current density and voltage. Most catalysts could not persist at high current density and voltage for a long time due to the corrosion caused by chloride and the resulting ClOR, so reports on this kind of catalyst are limited based on a literature review.39–42 Usually, two general principles can be applied to design this kind of catalyst: (1) developing robust OER catalysts with low overpotential and high current density (e.g., <490 mV at 500 mA cm−2) and (2) modifying the microenvironment near the anode surface via rational catalyst design to switch the potential equilibrium from the ClOR to the OER.
Chang et al. rationally designed iron and phosphor dual-doped nickel selenide nanoporous films (Fe,P–NiSe2 NFs) as bifunctional catalysts for the OER and HER for direct seawater electrolysis and observed excellent performance toward the OER, as shown in Fig. 1.43 The overpotential for the OER at 500 mA cm−2 is less than 400 mV with the obtained Fe,P–NiSe2 NF catalysts, and a high current density of 800 mA cm−2 with high OER selectivity (>90%) and long-term stability (200 h) was achieved at a cell voltage of 1.8 V. The authors claimed that the doping of Fe cations enhanced the activity and selectivity for the OER, and the doping of P anions improved the electronic conductivity and corrosion resistance by forming a passivation layer consisting of P–O species.
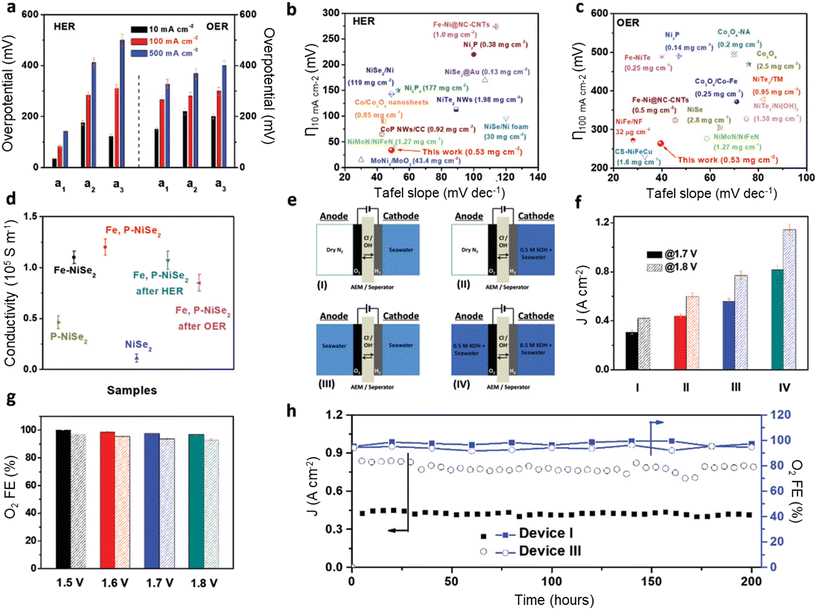 |
| Fig. 1 Seawater electrolyzer performance using bifunctional Fe,P–NiSe2 NF catalysts. (a) Comparison of the HER (left) and OER (right) overpotentials required to achieve different current densities for a1(Fe,P–NiSe2 NFs), a2 (Fe–NiSe2 NFs), and a3 (P–NiSe2 NFs); (b and c) comparison of Tafel slopes and overpotentials required to achieve current densities of 10 mA cm−2 for the HER (b) and 100 mA cm−2 for the OER (c) of Fe,P–NiSe2 NFs with those of benchmark catalysts; (d) the electrical conductivity of all samples; (e) schematics of AEM electrolyzers using different electrolyte feeding modes; (f) the current density of AEM electrolyzers at Ecell values of 1.7 and 1.8 V in different seawater feeding modes; (g) FE of O2 using the asymmetric device I (solid) and symmetric device III (dotted) feeding modes using natural seawater as a feedstock solution at different Ecell values; (h) long-term operation test of asymmetric device I and symmetric device III seawater electrolyzers showing high selectivity and stability at 1.8 V. Reprinted with permission from ref. 43. Copyright 2021 John Wiley and Sons. | |
Recently, Guo et al. reported that introducing a hard Lewis acid layer (for example, Cr2O3) over various transition metal oxide catalysts can manipulate their local reaction microenvironment (introducing OH− anions) at the anode surface in direct seawater electrolysis.44 A substantial activity enhancement was observed with this in situ artificially created local alkaline environment on the surface of Lewis acid-modified electrodes (Cr2O3–CoOx). Simultaneously, the ClOR was suppressed due to the low chloride concentration near the catalyst surface, as shown in Fig. 2. It can be seen that the performance of Cr2O3–CoOx in natural seawater significantly surpassed that of CoOx in natural seawater and approached that of CoOx in alkaline seawater electrolytes. Additionally, Cr2O3–CoOx exhibited much higher stability in natural seawater with high selectivity (>90%) compared with CoOx catalysts in both natural and alkaline seawater electrolytes. The authors found that the pH near the Cr2O3–CoOx anode surface (∼14) was much higher than that in the bulk electrolyte (∼8), and they attributed the high performance toward the OER to the local pH increase by the introduction of the hard Lewis acid Cr2O3, where the OER becomes thermodynamically favorable over the ClOR. This work provides a novel potential approach to design catalysts for direct seawater electrolysis rationally.
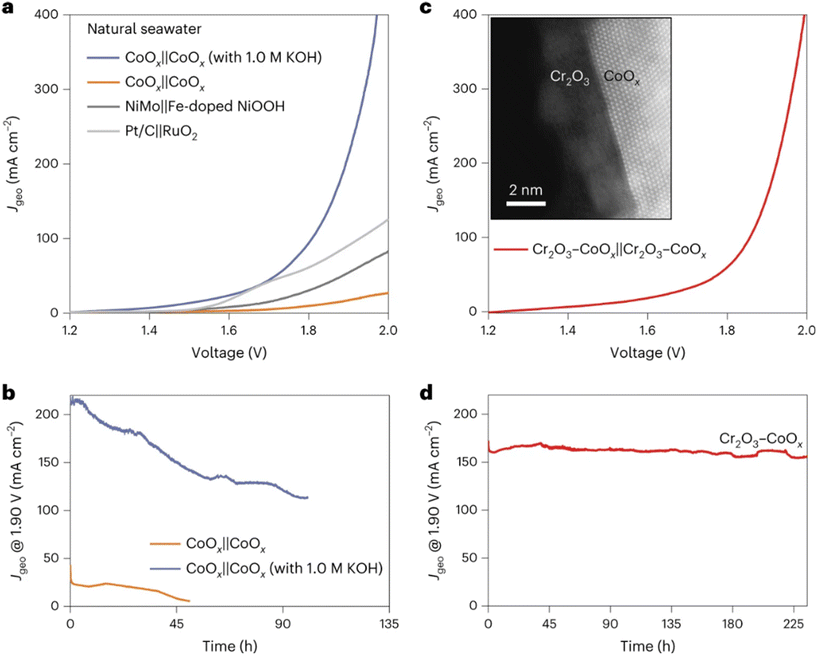 |
| Fig. 2 Seawater electrolysis performance of various catalysts. (a) Overall seawater splitting performance of CoOx, NiMo‖Fe-doped NiOOH and Pt/C‖RuO2; (b) long-term durability test of CoOx at 1.90 V in natural and alkaline seawater; (c) overall seawater splitting performance of Cr2O3–CoOx in natural seawater, with the inset showing the high-angle annular dark field-scanning transmission electron microscopy (HAADF-STEM) image of Cr2O3–CoOx; (d) long-term durability test of Cr2O3–CoOx at 1.90 V in natural seawater. Reprinted with permission from ref. 44. Copyright 2023 Springer Nature. | |
2.2 The introduction of additives to seawater electrolytes
As we described earlier, the selectivity for the OER and ClOR depends on the pH (H+) and chloride concentration, and the introduction of an alkali favors the selectivity to the OER over the ClOR. Consequently, alkaline seawater electrolysis has been investigated extensively in the past decade.45–56 We evaluated 316 stainless-steel (SS) mesh and Ni foam electrodes for the OER in alkaline (0.1, 0.5, and 1 M NaOH/KOH) seawater (simulated seawater, 0.5 M NaCl) electrolysis and observed that the overpotentials at 10 mA cm−2 for both electrodes decreased with the increase of alkali concentration.57 The lowest overpotential of 352 at 10 mA cm−2 was achieved with the SS mesh electrode, which was ∼150 mV less than that of the Ni foam electrode and comparable to those of commercial RuO2 and IrO2 catalysts. Recently, we reported that the performance of the 316 SS mesh electrode was promising and comparable to that of the 304 SS electrode in high alkalinity (1 M NaOH) natural seawater electrolyte, while it was much superior to the 304 SS electrode in pure natural and low alkalinity (0.1 M NaOH) seawater electrolytes regarding the electrocatalytic activity and corrosion resistance, as shown in Fig. 3.58 The finding indicated that the slight difference in material composition (e.g., Ni, Cr, and Mo) between 316 and 304 SS could cause a considerable difference in corrosion resistance for seawater electrolysis, even though their electrocatalytic activities toward the OER were similar in pure alkaline or highly alkaline seawater electrolytes, which suggests that both the OER performance and corrosion resistance are critical parameters in catalyst design for alkaline seawater electrolysis.
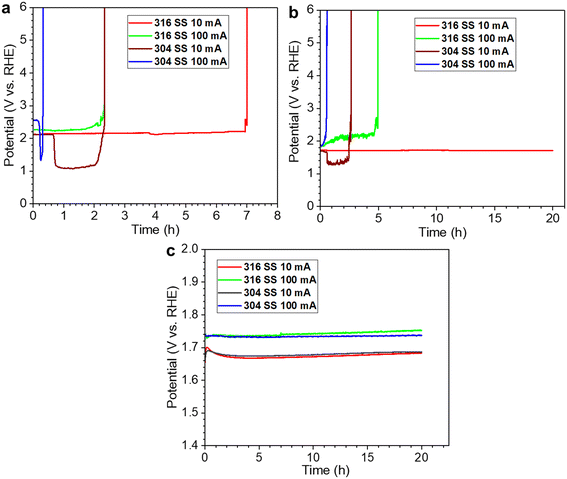 |
| Fig. 3 Stability tests with the 304 and 316 SS electrodes in different electrolytes: (a) pure natural seawater; (b) natural seawater containing 0.1 M NaOH; (c) natural seawater electrolyte containing 1 M NaOH. All currents shown here are also the current density, as 1 cm2 electrodes were used. Reprinted with permission from ref. 58. Copyright 2023 Elsevier. | |
Besides adding an alkali to the seawater electrolyte, salts without chloride (e.g., sulfate and phosphate) can also be added to suppress the ClOR and minimize the corrosion effect,36,59 as the presence of sulfate and phosphate anions near the anodic catalyst surface could decrease the local chloride concentration while these anions would not be oxidized. Ma et al. investigated the addition of sulfate for the OER with a Ni foam anode for alkaline seawater electrolysis and found that the addition of sulfate could significantly enhance the corrosion resistance, resulting in prolonged operating durability, as shown in Fig. 4.60 According to in situ experiments and theoretical simulations, they demonstrated that sulfate anions were preferentially adsorbed on the anode surface and then formed a negative charge layer, which repulsed the chloride anions via electrostatic repulsion. The adsorbed sulfate exhibited ca. 5 times stability and maintained a similar OER activity compared to the traditional alkaline seawater electrolyte. This work provides potential salt additives to seawater electrolytes, which can be a general approach for highly active OER catalysts in seawater electrolysis.
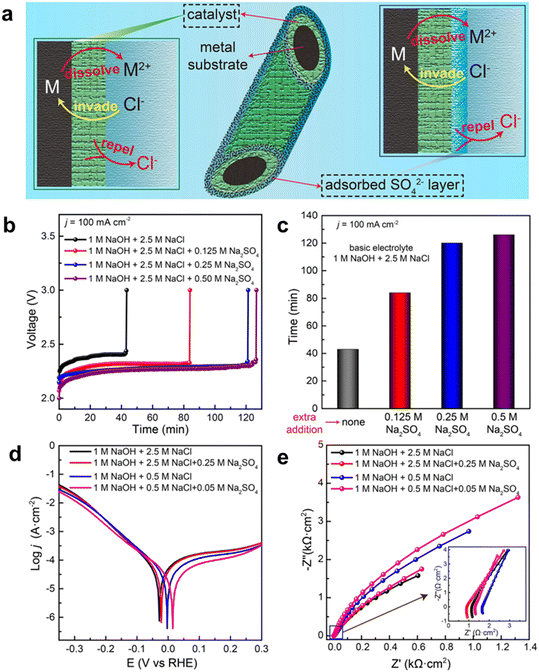 |
| Fig. 4 Schematic illustration of chloride repulsion and performance evaluation in various electrolytes. (a) Catalyst optimization (left) and electrolyte optimization (right) to protect the metal substrate from Cl− corrosion; (b) stability tests recorded at a constant current of 100 mA cm−2 for pure NF in electrolytes with different proportions of Na2SO4; (c) the durability of NF in different electrolytes; (d) and (e) Tafel plots and Nyquist impedance plots of NF in different electrolytes (1 M NaOH + 2.5 M NaCl with and without 0.25 M Na2SO4; 1 M NaOH + 0.5 M NaCl with and without 0.05 M Na2SO4). Reprinted with permission from ref. 60. Copyright 2021 John Wiley and Sons. | |
2.3 The combination of catalyst development and electrolyte preference
The corrosion of electrodes may still be a concern even with highly active OER catalysts in alkaline seawater media due to the metal chloride–hydroxide corrosion mechanism; the combination of robust and highly active OER catalysts and appropriate electrolyte preference is a promising way to achieve super OER performance and minimize electrode corrosion.61–71 Kuang et al. developed a multilayer anode containing a nickel–iron hydroxide electrocatalyst layer uniformly coated on a nickel sulfide layer on porous Ni foam (NiFe/NiSx–Ni), as shown in Fig. 5, and observed superior catalytic activity and corrosion resistance in alkaline seawater electrolysis operating at industrially required current densities (0.4 to 1 A cm−2) for over 1000 h.31 They observed that passivating layers were formed in the anode surface due to the in situ-generated carbonate-rich and polyatomic sulfate products, resulting in repelling chloride and exhibiting superior corrosion resistance in alkaline seawater electrolysis.
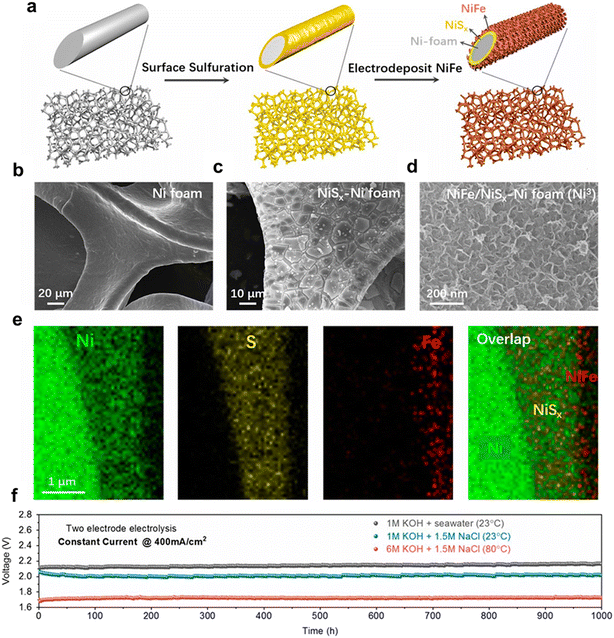 |
| Fig. 5 Fabrication and structure of the dual-layer NiFe/NiSx-Ni foam (Ni3) anode for seawater splitting. (a) Schematic drawing of the fabrication process, including a surface sulfuration step and in situ electrodeposition of NiFe; (b–d) SEM images of untreated nickel foam, NiSx formed on nickel foam, and electrodeposited NiFe on the NiSx surface; (e) elemental mapping of a cross-section of NiFe/NiSx on a Ni wire in the Ni foam, revealing the Ni wire, NiSx, and NiFe layers; (f) durability tests (1000 h) performed at a constant current of 400 mA cm−2 for the seawater-splitting electrolyzer in 1 M KOH + real seawater at room temperature and 6 M KOH electrolyte at 80 °C. Reproduced from ref. 31 with permission from the PANS. | |
Yu et al. reported a facile one-step approach for synthesizing S-doped Ni/Fe (oxy)hydroxide catalysts on a Ni foam substrate with high porosity and good hydrophilicity and observed that the prepared electrode exhibited extraordinary OER performance in alkaline seawater electrolysis, as shown in Fig. 6.72 The authors found that the doped S could enhance both the activity and stability of Ni/Fe (oxy)hydroxide catalysts toward the OER. The high activity may contribute to the optimization of the absorption energy of OER intermediates via changing the valence state of Ni/Fe by doping S, and the reason leading to superior corrosion resistance is still under investigation.
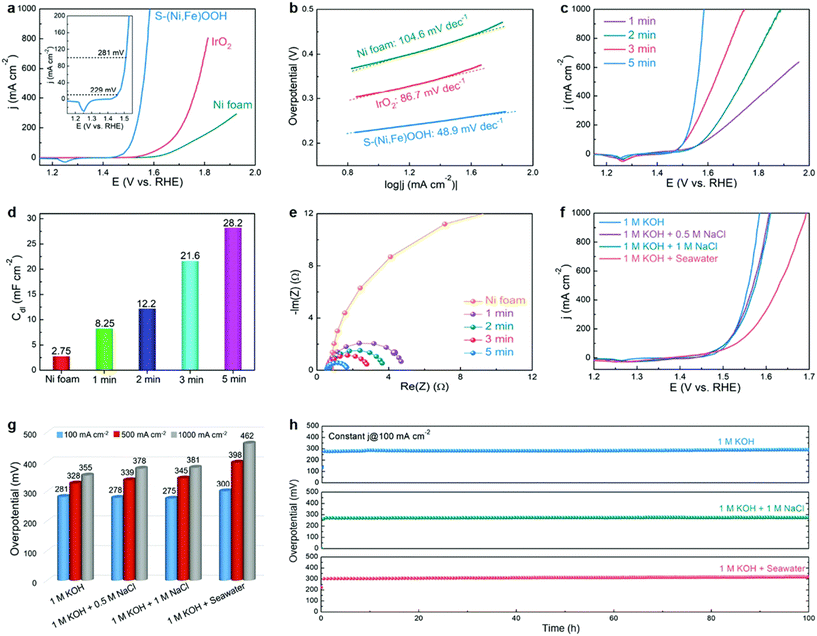 |
| Fig. 6 Performance evaluation of seawater electrolysis with various catalysts and electrolytes. (a) Polarization curves and (b) corresponding Tafel plots of the Ni foam, IrO2, and S–(Ni,Fe)OOH electrodes. The inset in (a) shows the polarization curve of S–(Ni,Fe)OOH over a small current density range; (c) polarization curves, (d) Cdl values, and (e) EIS Nyquist plots of the S–(Ni,Fe)OOH electrodes prepared using different reaction times; (f) polarization curves and (g) comparison of the overpotentials required to achieve current densities of 100, 500, and 1000 mA cm−2 for the S–(Ni,Fe)OOH electrode tested in different electrolytes; (h) long-term stability tests at a constant current density of 100 mA cm−2 for the S–(Ni,Fe)OOH electrode in different electrolytes. Reproduced from ref. 72 with permission from the Royal Society of Chemistry. | |
3 Summary and outlook
Electrochemical seawater splitting has been considered a clean and continual way to produce green H2 fuel from earth-abundant renewable seawater resources, which is vital for the future energy structure. However, the presence of impurities and their interference, especially high chloride content, make seawater electrocatalysis much more challenging than fresh or alkaline water electrocatalysis by competing with low hydroxide anion content for anodic reactions. The key is the development of effective strategies to enhance the selectivity and activity for the OER and suppress undesired electrochemical reactions mainly associated with the ClOR, which can lead to low OER selectivity and corrosion issues. In this minireview, we summarize three general approaches used in seawater electrolysis based on three concepts: (1) the sole development of robust and high-performance OER catalysts in pure seawater electrolytes, (2) the introduction of additives to seawater electrolytes (e.g., alkalis and/or salts without chloride) to favor the OER and suppress the ClOR, and (3) the combination of approaches (1) and (2).
The sole development of robust and high-performance OER catalysts is an ideal approach for direct pure seawater electrolysis, although the requirement for this kind of catalyst to survive is super critical, especially at high current density and voltage. The use of additives to seawater electrolytes (e.g., alkalis and/or salts without chloride) integrating with regular highly active OER catalysts is a potential way to realize green H2 production at the industrial level, although corrosion may still be a concern due to the metal chloride–hydroxide corrosion mechanism. The combination of catalyst development and electrolyte preference is the most practical approach for the large-scale production of green H2, but the cost is also the highest. Besides the selectivity and activity toward the OER in seawater electrolysis, the long-term durability of OER catalysts, which may play an even more important role in large-scale electrolysis industrialization, has been much less emphasized. Electrocatalysts for achieving long-term stability in natural seawater electrolytes face more challenges compared with those in seawater mimics (e.g., 0.5 M NaCl), as biofouling and trace metal deposition on both electrodes also hurt the performance for long-term operation in natural seawater electrolysis, and a regular clean of the system may be required. Therefore, the effect of natural seawater electrolytes on the long-term stability should be considered and addressed when developing OER catalysts for seawater electrolysis. We believe that the green H2 generated from seawater electrolysis will accelerate the transformation of the world's energy scheme and provide a clean energy future with the development of catalyst design, electrolyte preference, and electrolyzer optimization.
Disclaimer
This manuscript has been authored by UT-Battelle, LLC, under contract DE-AC05-00OR22725 with the US Department of Energy (DOE). The US government retains and the publisher, by accepting the article for publication, acknowledges that the US government retains a nonexclusive, paid-up, irrevocable, worldwide license to publish or reproduce the published form of this manuscript, or allow others to do so, for US government purposes. The DOE will provide public access to these results of federally sponsored research in accordance with the DOE Public Access Plan (http://energy.gov/downloads/doe-public-access-plan).
Conflicts of interest
The authors declare no conflict of interest.
Acknowledgements
This research at Oak Ridge National Laboratory (ORNL), managed by UT Battelle, LLC, for the U.S. Department of Energy (DOE) under contract DE-AC05-00OR22725, was sponsored by the US DOE H2NEW consortium and DOE Water Power Technologies Office (WPTO) Marine Energy seedling program.
References
- R. d’Amore-Domenech, Ó. Santiago and T. J. Leo, Multicriteria analysis of seawater electrolysis technologies for green hydrogen production at sea, Renewable Sustainable Energy Rev., 2020, 133, 110166 CrossRef.
- S. Khatun, H. Hirani and P. Roy, Seawater electrocatalysis: Activity and selectivity, J. Mater. Chem. A, 2021, 9, 74–86 RSC.
- A. K. Sarker, A. K. Azad, M. G. Rasul and A. T. Doppalapudi, Prospect of green hydrogen generation from hybrid renewable energy sources: A review, Energies, 2023, 16, 1556 CrossRef CAS.
- F. Song, J. W. Straten, Y.-M. Lin, Y. Ding, R. Schlögl, S. Heumann and A. K. Mechler, Binder-Free N-functionalized carbon electrodes for oxygen evolution reaction, ChemElectroChem, 2023, 10, e202201075 CrossRef CAS.
- X. Lyu, T. Van Cleve, E. Young, J. Li, H. Yu, D. A. Cullen, K. C. Neyerlin and A. Serov, Design of graded cathode catalyst layers with various ionomers for fuel cell application, J. Power Sources, 2023, 556, 232530 CrossRef CAS.
- T. Reshetenko, M. Odgaard, G. Randolf, K. K. Ohtaki, J. P. Bradley, B. Zulevi, X. Lyu, D. A. Cullen, C. J. Jafta and A. Serov, Design of PGM-free cathodic catalyst layers for advanced PEM fuel cells, Appl. Catal., B, 2022, 312, 121424 CrossRef CAS.
- J. Sharma, X. Lyu, T. Reshetenko, G. Polizos, K. Livingston, J. Li, D. L. Wood and A. Serov, Catalyst layer formulations for slot-die coating of PEM fuel cell electrodes, Int. J. Hydrogen Energy, 2022, 47, 35838–35850 CrossRef CAS.
- Z.-P. Wu, X. F. Lu, S.-Q. Zang and X. W. Lou, Non-noble-metal-based electrocatalysts toward the oxygen evolution reaction, Adv. Funct. Mater., 2020, 30, 1910274 CrossRef CAS.
- S. r. Dresp, F. Dionigi, M. Klingenhof and P. Strasser, Direct electrolytic splitting of seawater: Opportunities and challenges, ACS Energy Lett., 2019, 4, 933–942 CrossRef CAS.
- Z. Jia, X. Lyu, M. Zhao, J. Dang, L. Zhu, X. Guo, X. Wang, Z. Bai and L. Yang, In situ reconstructed Mo-doped amorphous FeOOH boosts the oxygen evolution reaction, Chem. – Asian J., 2023, 18, e202201305 CrossRef CAS PubMed.
- A. M. Díez, X. Lyu, M. Pazos, M. Á. Sanromán, G. McCool, O. I. Lebedev, Y. V. Kolen'ko and A. Serov, Retrofitting of carbon-supported bimetallic Ni-based catalysts by phosphorization for hydrogen evolution reaction in acidic media, Electrochim. Acta, 2023, 443, 141923 CrossRef.
- M. Khan, T. Al-Attas, S. Roy, M. M. Rahman, N. Ghaffour, V. Thangadurai, S. Larter, J. Hu, P. M. Ajayan and M. G. Kibria, Seawater electrolysis for hydrogen production: A solution looking for a problem?, Energy Environ. Sci., 2021, 14, 4831–4839 RSC.
- Q. Chen, Y. Yu, J. Li, H. Nan, S. Luo, C. Jia, P. Deng, S. Zhong and X. Tian, Recent progress in layered double hydroxide-based electrocatalyst for hydrogen evolution reaction, ChemElectroChem, 2022, 9, e202101387 CrossRef CAS.
- D. Guan, H. Xu, Q. Zhang, Y.-C. Huang, C. Shi, Y.-C. Chang, X. Xu, J. Tang, Y. Gu, C.-W. Pao, S.-C. Haw, J.-M. Chen, Z. Hu, M. Ni and Z. Shao, Identifying a universal activity descriptor and a unifying mechanism concept on perovskite
oxides for green hydrogen production, Adv. Mater., 2023, 2305074 CrossRef PubMed.
- H. Sun, X. Xu, H. Kim, W. Jung, W. Zhou and Z. Shao, Electrochemical water splitting: Bridging the gaps between fundamental research and industrial applications, Energy Environ. Mater., 2023, e12441 CrossRef.
- S. G. Simoes, J. Catarino, A. Picado, T. F. Lopes, S. Di Berardino, F. Amorim, F. Girio, C. Rangel and T. P. de Leao, Water availability and water usage solutions for electrolysis in hydrogen production, J. Cleaner Prod., 2021, 315, 128124 CrossRef CAS.
- J. Liu, S. Duan, H. Shi, T. Wang, X. Yang, Y. Huang, G. Wu and Q. Li, Rationally designing efficient electrocatalysts for direct seawater splitting: Challenges, achievements, and promises, Angew. Chem., Int. Ed., 2022, 61, e202210753 CrossRef CAS PubMed.
- X. Xiao, L. Yang, W. Sun, Y. Chen, H. Yu, K. Li, B. Jia, L. Zhang and T. Ma, Electrocatalytic water splitting: From harsh and mild conditions to natural seawater, Small, 2022, 18, 2105830 CrossRef CAS PubMed.
- T. u. Haq, M. Pasha, Y. Tong, S. A. Mansour and Y. Haik, Au nanocluster coupling with Gd-Co2B nanoflakes embedded in reduced TiO2 nanosheets: Seawater electrolysis at low cell voltage with high selectivity and corrosion resistance, Appl. Catal., B, 2022, 301, 120836 CrossRef CAS.
- Q. Zhou, L. Liao, H. Zhou, D. Li, D. Tang and F. Yu, Innovative strategies in design of transition metal-based catalysts for large-current-density alkaline water/seawater electrolysis, Mater. Today Phys., 2022, 26, 100727 CrossRef CAS.
- K. Shah, R. Dai, M. Mateen, Z. Hassan, Z. Zhuang, C. Liu, M. Israr, W. C. Cheong, B. Hu and R. Tu, Cobalt single atom incorporated in ruthenium oxide sphere: A robust bifunctional electrocatalyst for HER and OER, Angew. Chem., 2022, 134, e202114951 CrossRef PubMed.
- X. Xu, Y. Pan, L. Ge, Y. Chen, X. Mao, D. Guan, M. Li, Y. Zhong, Z. Hu, V. K. Peterson, M. Saunders, C.-T. Chen, H. Zhang, R. Ran, A. Du, H. Wang, S. P. Jiang, W. Zhou and Z. Shao, High-Performance perovskite composite electrocatalysts enabled by controllable interface engineering, Small, 2021, 17, 2101573 CrossRef CAS PubMed.
- J. Song, C. Wei, Z.-F. Huang, C. Liu, L. Zeng, X. Wang and Z. J. Xu, A review on fundamentals for designing oxygen evolution electrocatalysts, Chem. Soc. Rev., 2020, 49, 2196–2214 RSC.
- L. Fei, H. Sun, X. Xu, Y. Li, R. Ran, W. Zhou and Z. Shao, Understanding the bifunctional catalytic ability of electrocatalysts for oxygen evolution reaction and urea oxidation reaction: Recent advances and perspectives, Chem. Eng. J., 2023, 471, 144660 CrossRef CAS.
- J. Li, Y. Wang, H. Gao, S. Song, B. Lu, X. Tian, S. Zhou, Y. Yuan and J. Zang, Nickel boride/boron carbide particles embedded in boron-doped phenolic resin-derived carbon coating on nickel foam for oxygen evolution catalysis in water and seawater splitting, ChemSusChem, 2021, 14, 5499–5507 CrossRef CAS PubMed.
- S. Jiang, Y. Liu, H. Qiu, C. Su and Z. Shao, High selectivity electrocatalysts for oxygen evolution reaction and anti-chlorine corrosion strategies in seawater splitting, Catalysts, 2022, 12, 261 CrossRef CAS.
- L. Zhao, X. Li, J. Yu and W. Zhou, Design strategy of corrosion-resistant electrodes for seawater electrolysis, Materials, 2023, 16, 2709 CrossRef CAS PubMed.
- K. Hemmati, A. Kumar, A. R. Jadhav, O. Moradlou, A. Z. Moshfegh and H. Lee, Nanorod array-based hierarchical NiO microspheres as a bifunctional electrocatalyst for a selective and corrosion-resistance seawater photo/electrolysis system, ACS Catal., 2023, 13, 5516–5528 CrossRef CAS.
- L. Yang, C. Feng, C. Guan, L. Zhu and D. Xia, Construction of seaurchin-like structured Ag2Se-Ag2S-CoCH/NF electrocatalyst with high catalytic activity and corrosion resistance for seawater electrolysis, Appl. Surf. Sci., 2023, 607, 154885 CrossRef CAS.
- J. G. Vos, Z. Liu, F. D. Speck, N. Perini, W. Fu, S. Cherevko and M. T. M. Koper, Selectivity trends between oxygen evolution and chlorine evolution on iridium-based double perovskites in acidic media, ACS Catal., 2019, 9, 8561–8574 CrossRef CAS.
- Y. Kuang, M. J. Kenney, Y. Meng, W.-H. Hung, Y. Liu, J. E. Huang, R. Prasanna, P. Li, Y. Li and L. Wang, Solar-driven, highly sustained splitting of seawater into hydrogen and oxygen fuels, Proc. Natl. Acad. Sci. U. S. A., 2019, 116, 6624–6629 CrossRef CAS PubMed.
- J. Li, J. Sun, Z. Li and X. Meng, Recent advances in electrocatalysts for seawater splitting in hydrogen evolution reaction, Int. J. Hydrogen Energy, 2022, 47, 29685–29697 CrossRef CAS.
- L. Zhuang, J. Li, K. Wang, Z. Li, M. Zhu and Z. Xu, Structural buffer engineering on metal oxide for long-term stable seawater splitting, Adv. Funct. Mater., 2022, 32, 2201127 CrossRef CAS.
- Q. Mao, K. Deng, H. Yu, Y. Xu, Z. Wang, X. Li, L. Wang and H. Wang, In situ reconstruction of partially hydroxylated porous Rh metallene for ethylene glycol-assisted seawater splitting, Adv. Funct. Mater., 2022, 32, 2201081 CrossRef CAS.
- K. Deng, Q. Mao, W. Wang, P. Wang, Z. Wang, Y. Xu, X. Li, H. Wang and L. Wang, Defect-rich low-crystalline Rh metallene for efficient chlorine-free H2 production by hydrazine-assisted seawater splitting, Appl. Catal., B, 2022, 310, 121338 CrossRef CAS.
- M. Yu, J. Li, F. Liu, J. Liu, W. Xu, H. Hu, X. Chen, W. Wang and F. Cheng, Anionic formulation of electrolyte additive towards stable electrocatalytic oxygen evolution in seawater splitting, J. Energy Chem., 2022, 72, 361–369 CrossRef CAS.
- T. u. Haq and Y. Haik, S doped Cu2O-CuO nanoneedles array: Free standing oxygen evolution electrode with high efficiency and corrosion resistance for seawater splitting, Catal. Today, 2022, 400–401, 14–25 CrossRef CAS.
- G. Bahuguna, B. Filanovsky and F. Patolsky, Pioneering practical direct sea water splitting via an intrinsically-selective chlorine-phobic nickel polysulphide nanostructured electrocatalyst for pure oxygen evolution, Nano Energy, 2023, 111, 108439 CrossRef CAS.
- H. Abe, A. Murakami, S. Tsunekawa, T. Okada, T. Wakabayashi, M. Yoshida and M. Nakayama, Selective catalyst for oxygen evolution in neutral brine electrolysis: An oxygen-deficient manganese oxide film, ACS Catal., 2021, 11, 6390–6397 CrossRef CAS.
- H. Abe, T. Kobayakawa, H. Maruyama, T. Wakabayashi and M. Nakayama, Thin film coating of Mg-intercalated layered MnO2 to suppress chlorine evolution at an IrO2 anode in cathodic protection, Electrocatalysis, 2019, 10, 195–202 CrossRef CAS.
- W. Zheng, L. Y. S. Lee and K.-Y. Wong, Improving the performance stability of direct seawater electrolysis: From catalyst design to electrode engineering, Nanoscale, 2021, 13, 15177–15187 RSC.
- Y. Xu, H. Lv, H. Lu, Q. Quan, W. Li, X. Cui, G. Liu and L. Jiang, Mg/seawater batteries driven self-powered direct seawater electrolysis systems for hydrogen production, Nano Energy, 2022, 98, 107295 CrossRef CAS.
- J. Chang, G. Wang, Z. Yang, B. Li, Q. Wang, R. Kuliiev, N. Orlovskaya, M. Gu, Y. Du, G. Wang and Y. Yang, Dual-doping and synergism toward high-performance seawater electrolysis, Adv. Mater., 2021, 33, 2101425 CrossRef CAS PubMed.
- J. Guo, Y. Zheng, Z. Hu, C. Zheng, J. Mao, K. Du, M. Jaroniec, S.-Z. Qiao and T. Ling, Direct seawater electrolysis by adjusting the local reaction environment of a catalyst, Nat. Energy, 2023, 8, 264–272 CAS.
- S. Dresp, T. Ngo Thanh, M. Klingenhof, S. Brückner, P. Hauke and P. Strasser, Efficient direct seawater electrolysers using selective alkaline NiFe-LDH as OER catalyst in asymmetric electrolyte feeds, Energy Environ. Sci., 2020, 13, 1725–1729 RSC.
- S. Dresp, F. Dionigi, S. Loos, J. Ferreira de Araujo, C. Spöri, M. Gliech, H. Dau and P. Strasser, Direct electrolytic splitting of seawater: activity, selectivity, degradation, and recovery studied from the molecular catalyst structure to the electrolyzer cell level, Adv. Energy Mater., 2018, 8, 1800338 CrossRef.
- F. Dionigi, T. Reier, Z. Pawolek, M. Gliech and P. Strasser, Design criteria, operating conditions, and nickel–Iron hydroxide catalyst materials for selective seawater electrolysis, ChemSusChem, 2016, 9, 962–972 CrossRef CAS PubMed.
- Y. S. Park, J. Lee, M. J. Jang, J. Yang, J. Jeong, J. Park, Y. Kim, M. H. Seo, Z. Chen and S. M. Choi, High-performance anion exchange membrane alkaline seawater electrolysis, J. Mater. Chem. A, 2021, 9, 9586–9592 RSC.
- G. Amikam, P. Nativ and Y. Gendel, Chlorine-free alkaline seawater electrolysis for hydrogen production, Int. J. Hydrogen Energy, 2018, 43, 6504–6514 CrossRef CAS.
- F.-Y. Gao, P.-C. Yu and M.-R. Gao, Seawater electrolysis technologies for green hydrogen production: Challenges and opportunities, Curr. Opin. Chem. Eng., 2022, 36, 100827 CrossRef.
- X. H. Wang, Y. Ling, B. Wu, B. L. Li, X. L. Li, J. L. Lei, N. B. Li and H. Q. Luo, Doping modification, defects construction, and surface engineering: Design of cost-effective high-performance electrocatalysts and their application in alkaline seawater splitting, Nano Energy, 2021, 87, 106160 CrossRef CAS.
- J. Lu, C. Li, H. Wang, S. Ji, X. Wang and R. Wang, How to get to best oxygen evolution behavior from the electrolysis practice of the seawater, Int. J. Hydrogen Energy, 2021, 46, 12936–12943 CrossRef CAS.
- Y. S. Park, J.-Y. Jeong, M. J. Jang, C.-Y. Kwon, G. H. Kim, J. Jeong, J.-h. Lee, J. Lee and S. M. Choi, Ternary layered double hydroxide oxygen evolution reaction electrocatalyst for anion exchange membrane alkaline seawater electrolysis, J. Energy Chem., 2022, 75, 127–134 CrossRef CAS.
- T. He, Q. Liu, H. Fan, Y. Yang, H. Wang, S. Zhang, R. Che and E. Wang, Exploring the effect of ion concentrations on the electrode activity and stability for direct alkaline seawater electrolysis, Int. J. Hydrogen Energy, 2023, 48, 19385–19395 CrossRef CAS.
- J. Zhu, R. Lu, W. Shi, L. Gong, D. Chen, P. Wang, L. Chen, J. Wu, S. Mu and Y. Zhao, Epitaxially grown Ru clusters–nickel nitride heterostructure advances water electrolysis kinetics in alkaline and seawater media, Energy Environ. Mater., 2023, 6, e12318 CrossRef CAS.
- D. Wu, D. Chen, J. Zhu and S. Mu, Ultralow Ru incorporated amorphous cobalt-based oxides for high-current-density overall water splitting in alkaline and seawater media, Small, 2021, 17, 2102777 CrossRef CAS PubMed.
- X. Lyu, J. Li, C. J. Jafta, Y. Bai, C. P. Canales, F. Magnus, Á. S. Ingason and A. Serov, Investigation of oxygen evolution reaction with Ni foam and stainless-steel mesh electrodes in alkaline seawater electrolysis, J. Environ. Chem. Eng., 2022, 10, 108486 CrossRef CAS.
- X. Lyu, Y. Bai, J. Li, R. Tao, J. Yang and A. Serov, Investigation of oxygen evolution reaction with 316 and 304 stainless-steel mesh electrodes in natural seawater electrolysis, J. Environ. Chem. Eng., 2023, 11, 109667 CrossRef CAS.
- S. Bolar, S. Shit, N. Chandra Murmu and T. Kuila, Progress in theoretical and experimental investigation on seawater electrolysis: Opportunities and challenges, Sustainable Energy Fuels, 2021, 5, 5915–5945 RSC.
- T. Ma, W. Xu, B. Li, X. Chen, J. Zhao, S. Wan, K. Jiang, S. Zhang, Z. Wang and Z. Tian, The critical role of additive sulfate for stable alkaline seawater oxidation on nickel-based electrodes, Angew. Chem., 2021, 133, 22922–22926 CrossRef.
- L. Yu, Q. Zhu, S. Song, B. McElhenny, D. Wang, C. Wu, Z. Qin, J. Bao, Y. Yu and S. Chen, Non-noble metal-nitride based electrocatalysts for high-performance alkaline seawater electrolysis, Nat. Commun., 2019, 10, 1–10 CrossRef PubMed.
- P. Gayen, S. Saha and V. Ramani, Selective seawater splitting using pyrochlore electrocatalyst, ACS Appl. Energy Mater., 2020, 3, 3978–3983 CrossRef CAS.
- J. Li, T. Yu, K. Wang, Z. Li, J. He, Y. Wang, L. Lei, L. Zhuang, M. Zhu, C. Lian, Z. Shao and Z. Xu, Multiscale engineering of nonprecious metal electrocatalyst for realizing ultrastable seawater splitting in weakly alkaline solution, Adv. Sci., 2022, 9, 2202387 CrossRef CAS PubMed.
- L. Yu, Q. Zhu, S. Song, B. McElhenny, D. Wang, C. Wu, Z. Qin, J. Bao, Y. Yu, S. Chen and Z. Ren, Non-noble metal-nitride based electrocatalysts for high-performance alkaline seawater electrolysis, Nat. Commun., 2019, 10, 5106 CrossRef PubMed.
- H. J. Song, H. Yoon, B. Ju, D.-Y. Lee and D.-W. Kim, Electrocatalytic selective oxygen evolution of carbon-coated Na2Co1–xFexP2O7 nanoparticles for alkaline seawater electrolysis, ACS Catal., 2020, 10, 702–709 CrossRef CAS.
- M. Ning, F. Zhang, L. Wu, X. Xing, D. Wang, S. Song, Q. Zhou, L. Yu, J. Bao, S. Chen and Z. Ren, Boosting efficient alkaline fresh water and seawater electrolysis via electrochemical reconstruction, Energy Environ. Sci., 2022, 15, 3945–3957 RSC.
- H. Sun, J. Sun, Y. Song, Y. Zhang, Y. Qiu, M. Sun, X. Tian, C. Li, Z. Lv and L. Zhang, Nickel–cobalt hydrogen phosphate on nickel nitride supported on nickel foam for alkaline seawater electrolysis, ACS Appl. Mater. Interfaces, 2022, 14, 22061–22070 CrossRef CAS PubMed.
- N. Wen, Y. Xia, H. Wang, D. Zhang, H. Wang, X. Wang, X. Jiao and D. Chen, Large-scale synthesis of spinel NixMn3-xO4 solid solution immobilized with iridium single atoms for efficient alkaline seawater electrolysis, Adv. Sci., 2022, 9, 2200529 CrossRef CAS PubMed.
- H.-Y. Wang, J.-T. Ren, L. Wang, M.-L. Sun, H.-M. Yang, X.-W. Lv and Z.-Y. Yuan, Synergistically enhanced activity and stability of bifunctional nickel phosphide/sulfide heterointerface electrodes for direct alkaline seawater electrolysis, J. Energy Chem., 2022, 75, 66–73 CrossRef CAS.
- C. Wang, M. Zhu, Z. Cao, P. Zhu, Y. Cao, X. Xu, C. Xu and Z. Yin, Heterogeneous bimetallic sulfides based seawater electrolysis towards stable industrial-level large current density, Appl. Catal., B, 2021, 291, 120071 CrossRef CAS.
- E. Enkhtuvshin, K. M. Kim, Y.-K. Kim, S. Mihn, S. J. Kim, S. Y. Jung, N. T. Thu Thao, G. Ali, M. Akbar, K. Y. Chung, K. H. Chae, S. Kang, T. W. Lee, H. G. Kim, S. Choi and H. Han, Stabilizing oxygen intermediates on redox-flexible active sites in multimetallic Ni–Fe–Al–Co layered double hydroxide anodes for excellent alkaline and seawater electrolysis, J. Mater. Chem. A, 2021, 9, 27332–27346 RSC.
- L. Yu, L. Wu, B. McElhenny, S. Song, D. Luo, F. Zhang, Y. Yu, S. Chen and Z. Ren, Ultrafast room-temperature synthesis of porous S-doped Ni/Fe (oxy)hydroxide electrodes for oxygen evolution catalysis in seawater splitting, Energy Environ. Sci., 2020, 13, 3439–3446 RSC.
|
This journal is © Institute of Process Engineering of CAS 2023 |