DOI:
10.1039/D2IM00059H
(Review Article)
Ind. Chem. Mater., 2023,
1, 207-223
Perspective on oligomeric products from lignin depolymerization: their generation, identification, and further valorization†
Received
16th December 2022
, Accepted 9th February 2023
First published on 10th March 2023
Abstract
The present contribution emphasizes the formation of oligomeric products in various depolymerization approaches of lignin, namely reductive catalytic fractionation, oxidative catalytic fractionation, and pyrolysis. Three possible routes to form such oligomers in these depolymerization processes are summarized and compared from various studies conducted on model compounds. Next, the main identification techniques for characterizing oligomeric products are highlighted. Particular focus is given to 2D-HSQC-NMR, GPC, Maldi-TOF-MS and FT-ICR-MS, which represent the state-of-art characterization of lignin. Special attention was paid to the transferability of these techniques for depolymerized oligomeric lignin. Finally, both the existing and expected potential lignin valorization routes are discussed for these oligomers, and technical hurdles and recommendations are provided in an attempt to catalyze the development of new discoveries and enabling technologies.
Keywords: Lignin oligomer; Reductive and oxidative depolymerization; Biofuels; Characterization techniques; Lignin valorization.
1 Introduction
With the emphasis on transitioning away from fossil fuels and petroleum-based products, more and more research has moved toward exploiting renewable natural resources, and great interest has grown in applying the principles of “green chemistry” to valorize these materials, particularly via conversion to chemicals.1,2 Among the four source-independent categories of renewable materials (polysaccharides, lignin, triglycerides, and proteins), lignin is challenging to break down into chemically useful fragments, while the rest are relatively easy to decompose into their constituent building blocks.1,3–5 It is believed that natural lignin is an amorphous three-dimensional polymer comprising methoxylated phenylpropane structures, crossed-linked by C–O–C (β-O-4, α-O-4, 4-O-5) and C–C (β-1, β–β, 5–5) bonds.6 This structure intrinsically presents a unique challenge regarding its characterization, which then significantly hampered the establishment of lignin chemistry (e.g. radical-induced repolymerization). However, a progressive development of relative conversion technologies has been accomplished over the years;7–9 this is motivated by the fact that around 100 billion tons of lignin are naturally synthesized among plants in the biosphere, and approximately 1 billion is industrially produced per year as the side products.10,11 According to the existing pathways, various valuable chemical compounds can be produced through chemical treatments that aim to break down the lignin into low-molecular fragments; therefore, these depolymerization processes have recently drawn increasing attention in the field of lignin valorization.2,3,12,13
Common targeted products from lignin depolymerization are monomeric phenols, aromatics, cycloalkanes, and dicarboxylic acids.12,14,15 The large-scale of one such process can be exemplified by the industrial production of vanillin through oxidative catalytic depolymerization (OCD).13,16 The relatively low-cost of the substrate and the ease of operation counteract the fact that the yield of vanillin is often not high,3,17,18 which overall still makes this process economically viable.18 The same logic, however, may not be well applied to other lignin-valorization routes, such as reductive catalytic depolymerization (RCD), which is often a pre-treatment of lignin (via hydrogenolysis) if the final products are fuel-ranged cycloalkanes.19–21 A similar dilemma also exists in the thermochemical treatment of lignin, from which the pyrolysis process can yield only less than 15 wt% of monomeric phenolic compounds, leading to the rest of the products full of oligomeric phenols that are not GC-detectable.22,23 Regardless of the chemical treatments, oligomeric fractions from any lignin depolymerization are inevitably formed as the side products, and in many cases, can be the major products as well (Fig. 1). The inherent causes, although not very clear so far, have been extensively discussed in many studies.9,24–27 One of the commonly agreed viewpoints is the unavoidable participation of the crosslinking/repolymerization reactions during the proceeding of various lignin depolymerizations.28,29 Major efforts have been made to mitigate the rate of repolymerization for the individual process by 1) enhancing the efficiency of hydrogen transfer for RCD, 2) lowering the temperature for OCD, and 3) reducing the residence time for pyrolysis.30–36 All these attempts, however, could not eliminate the oligomers as the side products and therefore diverted our attention from studying pristine lignin to depolymerized lignin oligomers.
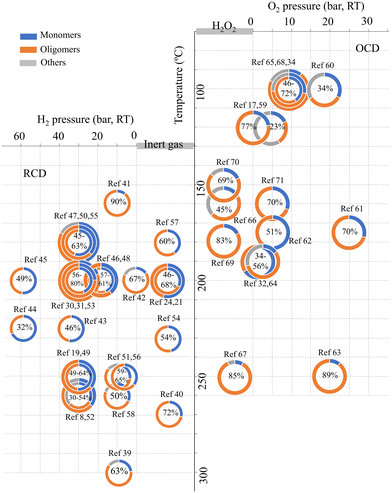 |
| Fig. 1 The yields of oligomeric products (orange-colored, the weight percentages of the oligomeric fractions are indicated inside the pie charts) from various reported depolymerization processes of lignin.8,17,19,21,24,30–32,34,39–71 | |
Currently, the available instrumental analysis of the oligomeric products from lignin depolymerization is usually inadequate because such products are neither volatile enough to be detected by gas chromatography techniques nor inherently heterogenous enough to be identified through the combined column separation and spectroscopy systems. A similar issue was previously identified to initialize the characterization of native lignin (e.g. lignin biosynthesis) but later circumvented by introducing the NMR techniques, in which a well-accepted protocol for analyzing the most common six interunit linkages in natural lignin was established.37,38 It is still ambiguous, however, whether such methods are well suited to characterizing the oligomeric products after depolymerization. As evidenced by model compound studies, there should be new chemical bonds presented in these oligomers based on the hypothesis that many of them are re-coupling and re-condensation products during depolymerizations. Therefore, in this short review, the current state-of-art instrumental analysis of these oligomeric products from lignin depolymerization is detailed, and our discussion focuses on the transferability of the techniques for natural lignin to the depolymerized oligomers in various processes. In addition, the current and existing valorization routes for the oligomers are listed and compared. Products that are derived from any other processes that are not designed for the depolymerization of lignin, such as industrial lignin generation and black liquor from the pulp industry, are beyond this scope and are not discussed in this review.
2 The generation of oligomeric products
2.1 Comparison between petro-based hydrocracking and lignin-based cracking and depolymerization
In the petro-based sector, the thermal cracking or hydrocracking-induced reactions were ensembled similarly to lignin depolymerization and were put together for comparison. The process is used to convert larger hydrocarbon molecules into smaller molecules under high hydrogen pressure and high temperature.72,73 Hydrocracking of saturated hydrocarbons could proceed by several mechanisms depending on the catalyst functionality.74,75 Even without a catalyst, thermal cracking occurs in chain reactions via radicals, in which β-scission is widely identified as the initiation step.76,77 Similar techniques were applied to lignin cracking or hydrocracking, in which fast pyrolysis and reductive catalytic fractionation or hydrogenolysis were developed. Under hydrocracking conditions, the aryl ether and unstable C–C bonds from the lignin structure are cleaved, often followed by hydro-dealkylation leading to the rupture of the alkyl side chain groups linked to the aromatic rings. A peculiarity in the lignin structure, however, is the pronounced presence of the hydroxyl group.78 Efficient oxidation leads to the formation of carbonyls that could result in the depolymerization of lignin, which is not derived from the petro-sector.3,79 The redox-neutral depolymerization is another noteworthy approach for lignin because it is a tandem catalytic dehydrogenation (oxidation)/C–O bond cleavage (reduction) that requires no added reagent.80 Several pioneered studies have indicated that the redox-neutral approach could also cleave the C–C bond (evidenced by lignin model compounds) with proper metal catalysts and ligands.81,82 Such a catalytic system has been applied to depolymerize dioxasolv lignin and alkaline lignin, affording aromatic ketones as the major monomer products.82,83
2.2 The chemistries during the formation of oligomeric products (thermally, reductively, oxidatively and redox-neutrally)
Extensive studies have reported that solid residues/char from various lignin depolymerization results from the repolymerization of the active lignin intermediates (monomers or oligomers). It is generally believed that lignin repolymerization occurs through the reaction of the lignin intermediate with electron-rich carbon atoms, such as the C-2 and C-6 of guaiacyl and syringyl rings, promoting the formation of new C–C linkages.24,37,47 However, it is not that explicit if the formation of tar-like lignin products (e.g. lignin oil and bio-oil) is solely due to the repolymerization as char; or simply a stabilization of those intermediates with the desired active agents, such as hydride, hydroxyl radical; or other small radical components (alkyl groups). Huang et al. investigated the effect of reaction time on the possible repolymerization during the catalytic depolymerization of alkali lignin in ethanol.39 The results indicate that the yield of the monomers and non-monomeric lignin oil (THF soluble tar) significantly increases with the extended reaction time, implying that tar is more likely from the forward depolymerization instead of the repolymerization. Studies from pyrolysis also suggest that both the phenolic hydroxyl group and unsaturated side chains are the most reactive structures present in the primary tar fraction.35,84–86 These chemical structures play an important role in repolymerization chemistry.39,86 It was particularly pointed out that the new carbon–carbon bonds are normally formed on the ortho- and para-positions via nucleophilic attack to the quinone methide intermediates during the condensation of many phenolic model compounds.84,86,87 The steric hindrance plays a role in oxidative cleavage reactions. Several possible routes that may lead to the formation of lignin oligomers are summarized in Fig. 2. Depending on the conditions, the final pathway for the formation of these oligomers can be a combination of various routes. For instance, under pyrolysis conditions, lignins are more likely to undergo all the routes and form the pyrolytic lignin, while route 1 or 3 is more often proposed in oxidative and the redox-neutral depolymerization of lignin depending on the reaction severity. In the case of RCD of lignin, route 3 is likely to prevail under low temperature, while higher temperature reportedly induces more of route 1 during depolymerization. Figueirêdo et al. used principal component analysis to confirm that temperature is a crucial parameter for the depolymerization of pyrolytic lignin.88 Using various wet chemical and spectroscopic methods, the pyrolytic lignin structure was elucidated by Bayerbach et al., who concluded that these oligomers emerge because of both recombination reactions (route 2) and thermal ejection of intact lignin fragments (route 3).89 For the oligomeric products from either RCD or OCD, it is still a debatable topic on exactly how they are generated at various reaction conditions, and advanced techniques are needed for detailed characterization to gain a better understanding of the types and nature of these oligomers.
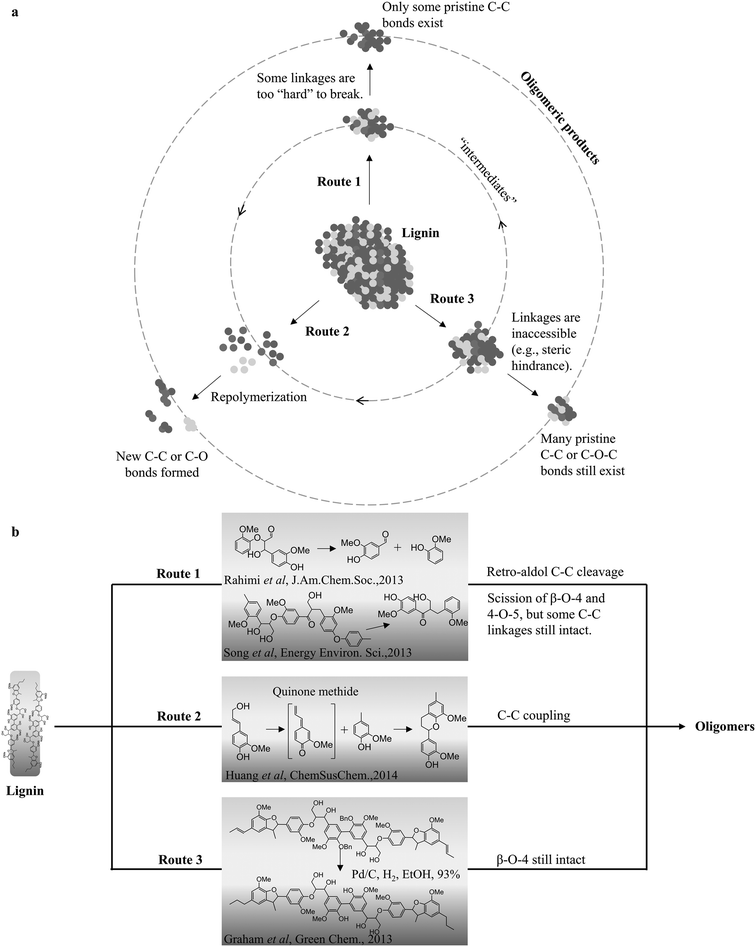 |
| Fig. 2 The proposed routes for the formation of oligomeric products during lignin depolymerization (a), evidenced by the respective model compound studies (b).24,39,79,90 | |
3 Identification of oligomeric products
3.1 The state-of-art
The state-of-the-art methods for characterizing these oligomeric products are Two-dimensional Heteronuclear Single Quantum Coherence nuclear magnetic resonance (2D-HSQC-NMR), gel permeation chromatography (GPC), matrix-assisted laser desorption/ionization-time of flight mass spectrometry (Maldi-TOF-MS), LTQ Orbitrap mass spectrometry (Elite) and Fourier-transform ion cyclotron resonance mass spectrometry(FT-ICR-MS), combined with other less informative instrumental analyses, such as UV/vis, UV-florescence, pyrolysis gas chromatography mass spectrum (py-GCMS), elemental analysis, thermogravimetric analysis (TGA) and Fourier-transform infrared spectroscopy(FTIR). It is desirable to identify each oligomer in the depolymerization products. A detailed comparison of the advantages and disadvantages of each technique has been thoroughly discussed in previous reviews. This paper focuses specifically on the recent developments in the characterization of oligomeric lignin products in this section.91,92
3.1.1 Chromatographic techniques (HPLC-MS, GC × GC–MS).
Owing to the large molecular weights of various lignin substrates, there are so far no identification techniques available that can provide complete structural information on these natural polymers. However, the depolymerization products of lignins, although diverse, can sometimes be a mixture of small monomers (such as phenol or guaiacol), which can be readily identified and even quantified by gas or liquid chromatography when attached to a mass spectrometer (such as GC–MS or HPLC–MS). The basic idea is that the depolymerized product mixture undergoes an effective separation through a column, and then each separated compound is transferred into the mass spectrometer for subsequent fragmentation. The fragmentation induced by the ionization of the molecule results in the formation of a characteristic mass spectrum for each compound with the information of the respective ionic fragment. In theory, such mass spectrum for each molecule should be unique and therefore offer the feasibility of identifying an unknown by solely comparing it with a known spectrum database. The comparisons are normally achieved using a database or library established with the mass spectrums of all the relevant commercially available chemical references. The higher the matching probability between the unknown and a known chemical, the more reliable the identification. Because most oligomeric products from lignin depolymerization are commercially unavailable, further identification can only rely on interpreting their mass spectrums, which can be very challenging owing to the complexity of the fragmentation chemistry of the oligomers. These techniques are therefore only limited to mostly lignin dimers, while the molecular size larger than a trimer is normally not detected by the GC–MS system. More often, a pre-derivatization via trimethylsilylation is used to improve the volatility needed to make the dimers GC-detectable.19 Until recently, GC × GC-MS or FID had been extensively applied in determining the dimers and trimers of lignin depolymerization products, but this technique is unsuitable for larger oligomers, which remain unidentified.27,39,88 LC–MS or HPLC–MS systems can separate oligomers to identify any known compounds. In practice, however, many unfamiliar molecular ion profiles are detected for the oligomeric lignin products, hampering their further identification. Synthetic model compounds are required to establish a database of oligomeric MS to make this analysis possible. Contrary to conventional liquid chromatography, supercritical fluid chromatography (SFC) uses supercritical CO2 as the mobile phase.93 To efficiently separate lignin oligomers, the SFC is often combined with reversed phase liquid chromatography.94 More recently, the newest protocol using the SFC to characterize lignin dimers has been developed and validated. The chromatogram showed baseline separation of all reference compounds in less than 10 min, and the response factors of the tested dimers did not differ significantly from one another, suggesting that this strategy is well suited for quantifying lignin dimers and oligomers.95
3.1.2 Molecular weight characterization (GPCMaldi-LC-MS, LTQ-Orbitrap Elite, ESI-MS, and FT-ICR-MS).
Another important index for characterizing these oligomeric products is the profile of their molecular weight (or molecular mass) distribution. The resulting average molecular weight is often used to measure the extent of depolymerization. Although several approaches, including GPC, Maldi-TOF, ESI-MS, and FT-ICR-MS, are often used for such purposes, so far, there is no census as to which approach would provide the most representative molecular weight of the lignin and its depolymerized oligomers. Both ESI-MS and Maldi-TOF are based on “soft ionization” methods in which ion formation does not lead to a significant loss of sample integrity. In general, mass spectrometry can provide structural information for many large molecules, such as peptides, and it is possible to determine known compounds quantitatively, especially when combined with chromatography. Owing to the complex nature of the lignin matrix, the resulting oligomers are often unknown; therefore, it is more common to conduct a semiquantitative evaluation with these mass spectrometry-based techniques.
The GPC has also been used extensively to characterize the molecular weight distribution although the mechanism differs from the MS-based techniques. In general, a set of standards is needed to calibrate the peaks arising from the GPC of an unknown sample. Often, a series of polystyrene standards are well accepted for use for such purposes, although they may not simulate the targeted lignin oligomers because of the distinct polarity and topological structure. For oligomeric lignin, the solvents are used to recover the product matter in terms of the resulting molecular weight that is reflected by the GPC. Van den Bosch et al. characterized the resulting oligomeric products that are recovered by dichloromethane from the RCD of Birch lignin. The GPC showed two major signals at circa 200 and 450 g mol−1 (based on polystyrene standards), suggesting successful depolymerization as the signal of the proto birch lignin appears above at least 1000 g mol−1.19 Huang et al. found that their oligomeric products recovered with THF (tetrahydrofuran) showed a molecular weight of around 900 g mol−1.39 One of the advantages of analyzing the oligomers is the avoided pre-derivatization step that is required for the natural lignin to increase the sample solubility in the eluent. It was proven that the THF can dissolve oligomers with a wide range of molecular weight, as evidenced by the work from Joffres et al.96 They characterized the oligomeric products (THF soluble) from depolymerization of a wheat straw soda lignin and concluded a bimodal distribution that the oligomers on average comprise 13 phenyl units compared to 27 units for the initial lignin.96 Similar bimodal distribution was also confirmed by the work from the Rinaldi group; the apparent molecular weight of the oligomeric lignin products was centered at approximately 300 and 1250 Da compared with the wide monomodal distribution for the original lignin with the Mw greater than 3000 Da.97 In addition to the main contribution to the reductively produced oligomers, GPC analysis also confirmed the presence of similar-sized molecules as the side products in oxidative and pyrolysis processes. For instance, Hafezisefat et al. used GPC to demonstrate the effectiveness of their oxidative depolymerization route in which the average molecular weight of the products could decrease from 511 Da to 287 Da, whereas the one from the reference (inert gas environment) increased from 505 Da to 661 Da.63 In addition, the oligomers from lignin pyrolysis had also been characterized by GPC, and many results indicated that the average molecular weight of the oligomeric products was in the range of 200–1000 g mol−1.98–101 Owing to the usage of other calibrants (high MW lignin oligomers are not commercially available), there are some uncertainty in the reported MW values from GPC analysis, and sometimes the results are merely comparative.102 This is because the separation in the GPC is not only driven by size exclusion but also by other interactions occurring because of lignin's heteropolymer nature and diverse functional groups.103–105 Therefore, the usage of the polystyrene standards cannot emulate the non-size-specific interactions and thus may result in a misleading calibration.
Unlike GPC, which relies heavily on the standards to calibrate, MS-based techniques are mostly considered direct measurements because each molecule can be individually estimated in theory. The accuracy and precision of the MS-based method, however, highly depend on the completeness of the ionization of all the oligomeric products. The ionization of lignin and its depolymerized products is significantly affected by the ionization interface, as pointed out in the work by Finch et al.106 They found that intense responses were obtained under positive APCI or negative ESI conditions. This result agrees with other similar studies in which only the negative ESI mode mass spectrometry was used to represent the lignin oligomers derived from the pyrolysis route.107,108 Kong et al. also discussed the limitation of using APPI ionization for the FT-ICR-MS characterization because only the species with molecular weights between 150 and 700 Da were counted from lignin depolymerization. This was due to the limited boiling point of the APPI.109 To compare the effect of depolymerization conditions, many reported ESI-MS data generally suggest that there is no distinct distribution in molecular weight for the oligomeric products from oxidative and reductive route.20,70 More recently, FT-ICR-MS and Maldi-TOF have received more attention for the characterization of the lignin depolymerization products because of their improved accuracy or enhanced reliability.110 Owing to the accuracy of the FT-ICR-MS, results can often be used to derive the molecular formula of components; then, the weighted average of carbon atom number, hydrogen atom number, oxygen atom number, and sulfur atom number can be calculated to form van Krevelen diagrams or other plots, such as heat maps, Kendrick mass defect, and carbon number versus double bond equivalent (DBE), to enhance the processing of the acquired data.91,111 Huber's group recently used the FT-ICR-MS data to plot the carbon number vs. DBE of the lignin oligomeric products and identified four main clusters corresponding to dimers (20 C, 10 DBE), trimers (30 C, 15 DBE), tetramers (39 C, 21 DBE), and pentamers (49 C, 25 DBE).112 Paananen et al. conducted a base-catalyzed oxidative depolymerization of Kraft lignin (softwood), and the FT-ICR-MS of the oxidation products showed the disappearance of signals over m/z 500, implying the efficient depolymerization of high-mass oligomers.110 A similar phenomenon was also reported by Zhang et al. in which catalytic transfer hydrogenolysis (RCD) was employed instead of oxidative depolymerization. Their FT-ICR-MS showed that the signal at m/z was over 500 and weakened after the hydrogenolysis of the Kraft lignin.113 Song et al. employed the Maldi-TOF technique to analyze oligomeric fractions from lignin alcoholysis (methanol) and indicated that the depolymerized products with molecular weight from m/z ca. 1100 to ca. 1600.24 In addition, Liu et al. detected oligomers with molecular weight mainly located from 248 to 496 m/z by Maldi-TOF from isopropanol-assisted lignin depolymerization.114 The molecular weight of the products from various depolymerization processes of lignin is displayed in Fig. 3. No direct relationship was observed between the temperature and the average molecular weight of the oligomers. For both techniques, the limitation of elucidating the structural information of the oligomers is due to the lack of standards for various lignin fragments. An attempt has been made to address the issue by some pioneer studies, in which a computational stochastic method was proposed to generate a molecular library for lignin that was used for supporting the assignment of potential candidate structures to compounds detected during FT-ICR-MS analysis.25 More recently, the LTQ orbitrap mass spectrometer is considered an alternative to FT-ICR-MS owing to its low operating cost. Although the mass resolution of orbitrap mass spectrometers is lower than that of FT-ICR-MS, the resolution for both Orbitrap Velos and Orbitrap Elite is sufficient to characterize the oligomers from pyrolysis oil.115
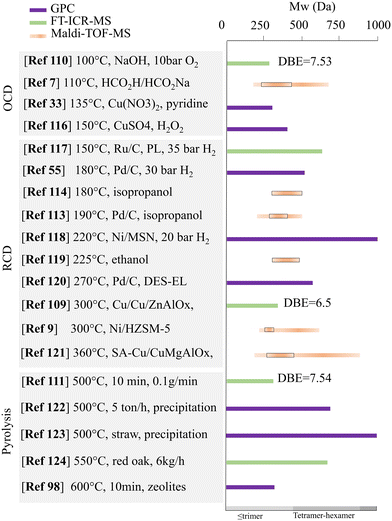 |
| Fig. 3 Molecular weight distribution (Maldi-TOF-MS) or the average molecular weight (GPC or FT-ICR-MS with the double bond equivalent value) for the products from various lignin depolymerization processes.7,9,33,55,98,109–111,113,114,116–124 | |
3.1.3 2D-NMR.
As the most useful technique, 2D-1H–13C NMR, specifically their HSQC, has been extensively used to characterize the interunit linkages of many lignin oligomers.67,120,125,126 Ideally, the 2D-HSQC spectra provide some information on the bond linkage between 1H and 13C. However, 13C has a natural abundance of just over 1%, and the major isotope (12C) is not NMR active, so very little proton signal is coupled.127 Owing to the weak sensitivity of the 13C nuclei, a very long relaxation delay is needed, and the signal-to-noise often remains poor even with very long acquisition times, thereby jeopardizing attempts to obtain reliable quantitative data.127 However, the 2D-HSQC is still considered state-of-the-art because it is the main technique used to identify the major interunit linkages, mainly β-O-4, β–β, β-5 and α-O-4, which had been confirmed by the respective model compounds. Additionally, this method is believed to be more effective in semi-quantifying the individual G/S/H unit and also G/S ratio in various lignins.128 For absolute quantification of the linkages and pendant units of lignin by 2D HSQC NMR spectra, it is still challenging as the signal integrations can be influenced by many parameters, such as T1 and T2 relaxation rates, carbon pulse offset effects, and multiplicity and magnitude of coupling constants.129,130Fig. 4 indicates the general identification of the interunit linkages in real lignin and the products from depolymerization by the 2D-NMR-HSQC method that was reported recently. For the oligomers from lignin depolymerization, many 2D-HSQC data suggest that within the oligomeric structures, more C–C bonds are presented with less C–O–C, and the repositioning of several functional groups (mainly methoxyl and hydroxyl) is also commonly observed in the newly formed oligomers. However, Ren et al. pointed out that new alkane C–C bonds were formed at the beginning of their hydrogenolysis reaction of the lignin but then decreased over time.131 Under pyrolysis conditions, it has been reported that β-O-4 in lignin is preferentially cleaved into small fragments that are then transformed into more stable C–C linkages.123 As recently reported for the RCD process, a clear correlation is observed between monomer distribution and the main linkages and aromatic unit constituents of the original lignin, as determined by 2D-HSQC-NMR samples.130 This correlation has also been well recognized in other lignin pyrolysis studies.87,132 It is noteworthy that the disappearance of β–β and β-5 signals in HSQC spectra does not necessarily imply depolymerization because, sometimes, the scission only occurs at their neighboring C–O–C positions (i.e., α-O-γ and α-O-4, respectively) with the C–C remain.96,97 Moreover, other new linkages, except for the well-known ones, have not been thoroughly reported but were possibly present in oligomeric production after lignin depolymerization. Ralph et al. mentioned three more interunit linkages in their papers, including 5–5 in the dibenzodioxocin type subunit, α-O-4 in the benzodioxane type, and β-1 in the spirodieneone type.37,38 However, these linkages have not been well reported elsewhere for various oligomeric lignin products.125,126,130
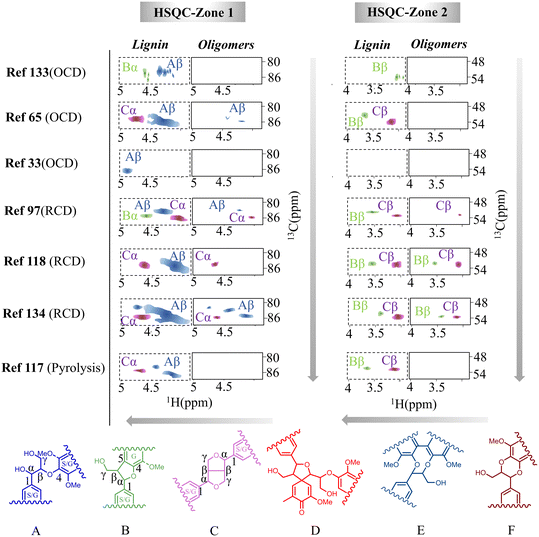 |
| Fig. 4 Characterization of the oligomeric products from various lignin depolymerization processes by 2D-HSQC-NMR analysis (the contour plots were adapted and normalized from each reference).33,65,97,117,118,133,134 | |
3.1.4 Other techniques.
Other techniques that could be used in characterizing the lignin oligomers, such as FTIR, TGA, UV-fluorescence and elemental analysis, are often considered to be auxiliary. These techniques can sometimes provide similar information from different perspectives or provide completely different dimensions. For instance, elemental analysis that provides the overall weight percentage of the carbon, hydrogen, nitrogen, and oxygen has been frequently conducted to form the van Krevelen plot to make the reaction pathways more indicative.135,136 It could also turn out to be a key piece in identifying an oligomer structure, especially when NMR and molecular weight had already been obtained. The fluorescence spectrum, however, is another technique to characterize polyphenols that are abundant in the oligomeric products of lignin depolymerization.137,138 This method is fast and effective in distinguishing oligomers with different molecular sizes although the corresponding quantification is still challenging. In addition, TGA is also a useful technique for displaying the boiling point curve of oligomeric products.121,139 Generally, the boiling point ranges of lignin monomer, dimer, and trimer were defined as 180–280 °C, 280–400 °C, and 400–500 °C, respectively, as referenced by the corresponding model compounds.139 A growing application of phosphitylation followed by 31P NMR can also be observed in the lignin characterization field to quantitatively analyze lignin structure, especially for the hydroxyl group.140,141
3.2 Challenges in identifying oligomeric products
Although many studies on characterizing lignin oligomers have been reported in the literature, key questions remain unresolved, particularly concerning our collective understanding of the exact structure of each oligomer. The major challenges in identifying the oligomers from various lignin depolymerization lie in the fact that most oligomers are mixed with the monomers in the produced lignin oils. Classical molecular weight characterization may not well represent the oligomeric class of interest owing to the relative difficulty in separating the monomers from the oligomers. Conventional liquid–liquid extraction, regardless of the solvents being used, ubiquitously recovered both components in one fraction. Recently, Stahl group attempted to push this boundary using centrifugal partition chromatography, offering access to isolated oligomers from the products.71 In addition, the resulting oligomeric products may have weak stability and react with each other to induce the aging phenomenon, as extensively reported in various bio-oils,85,142,143 posing an obstacle to fully understanding the intrinsic structure of the oligomers. Additionally, all the state-of-the-art techniques combined are still inadequate for distinguishing and then quantifying each oligomer. More efforts are needed on this aspect in the future, which is pivotal in utilizing these oligomeric products in many other applications.
4 Further valorization options for oligomeric products
Conventionally, depolymerization is considered to be one of the valorization routes for lignin by producing monomers as the only goal. With the development of this process, however, more efforts have been made in recent years to explore the valorization path for the side-produced oligomers.26,144 Possibly owing to the intrinsic nature of depolymerization, oligomeric products derived from different processes often have different fates in terms of their further valorization. For example, oligomers from RCD or OCD of lignin are often reported to be the precursors of polymer synthesis, while tar materials from pyrolysis are more likely to undergo thermal/catalytic decomposition for the production of smaller fragments.145–149 The reported pathways for the valorization of the oligomeric products can be generally categorized into three: 1) further depolymerization for more phenolic/aromatic monomers, 2) modification of lignin oligomers into value-added materials, and 3) decomposition to short-chain dicarboxylic acids or even hydrogen gas.
4.1 Enhancing the yield of monomers
Monomeric phenols derived from various lignin depolymerization processes can be readily converted into respective aromatics or cycloalkanes, which could potentially serve as renewable-carbon alternatives in chemical, materials, and fuel applications.150 As the side products, lignin oligomeric products can be valorized via a further depolymerization process. Huber's group conducted multistep hydrogenation of pyrolytic lignin, in which the oligomeric product was separated from the spent catalyst; then, a new hydrogenation step was repeated with fresh catalyst.125 A successful depolymerization process of the oligomeric products from the 1st step was observed in the next steps, resulting in an increase in monomer yield from 15.9% to 26.7%. This study perfectly exemplified the idea that many oligomeric lignins can be further fragmented to yield more monomers but not at their full scale. Even when undergoing multiple steps of hydrogenation, decent amounts of oligomers still exist in the final product.125 Evidenced by the 13C-NMR analysis, the final form of the oligomers exhibited more aliphatic C–C but fewer aromatics.125 The mechanism of the intrinsic transformation to oligomers after multiple hydrogenations is still unclear. According to the previously summarized mechanisms that accounted for the generation of oligomeric products, many chemical routes (i.e., hard bond, repolymerization, or yet incomplete depolymerization) could cause such issues, and more informative identifications are still needed to confirm accurate mechanisms. Wang et al. proposed a method to calculate the theoretical maximum yield of the monomer from lignin hydrogenolysis, as listed below:55
where Mn represents the average molar weight obtained from GPC analysis and N represents the polymerization degree calculated by Mn and theoretical general formulas for angiosperms (226.23 g mol−1).55 Experimentally, the yield of monomers from further hydrogenolysis of pyrolytic lignin (oligomers) was reported to be as high as 33 wt%.88 Song et al. optimized their nickel-based RCD and achieved a 48.5 wt% monomer yield from birch wood lignin.24 Additionally, many studies have been carried out to explore new possibilities for the cleavage of the relatively refractory carbon–carbon (C–C) interunit linkages under different conditions, aiming to maximize the monomer yield during depolymerization. For instance, Kim et al. had effectively activated the C–C bond cleavage in β-alkoxyl and β-1 lignin model dimers via copper-catalyzed oxidation although the same strategy had been tested with raw lignin.151 Ren et al. tested a similar approach for alkaline lignin at room temperature, and the 2D-HSQC-NMR results suggested a selective cleavage of the aliphatic C–C bond from the lignin although the dimeric-trimeric aromatic acids and aldehydes were identified as the main products.133
4.2 Modification of lignin oligomers into value-added materials
The chemical structure endows lignin oligomers with great potential as core components for functional materials.152,153 For instance, lignin oligomers can generate interesting photo physicochemical properties, attributed to the incorporated aromatic structures.154,155 More recently, Wan et al. developed an auto fabrication strategy to convert lignin into afterglow room temperature phosphorescence materials.156 Because the key step of the process was to oxidize lignin to aromatic chromophores, it offers the possibility that such a method could be implanted into the oligomeric lignin as well. In addition, owing to the lower molecular weight and dispersity index with high phenolic content, the depolymerized lignin oligomers could also serve as a great candidate to replace petroleum-derived aromatic precursors at least partially in the industrial synthesis of polymers. Among various depolymerization processes, the RCD of lignin has been widely accepted as a technology to effectively reduce the degree of lignin branching and the molecular weight of natural lignin.58,157 The resulting oligomeric lignin products, once coupled with an epoxide (such as epichlorohydrin), could readily transform a brown oily prepolymer, which is the main precursor for obtaining the epoxy resin adhesive.157 The low molecular weight lignin oligomers derived from the RCD process were also tested to fully replace the petroleum-based bisphenol A diglycidyl ether (hard to recycle) for synthesizing bio-based epoxy resins.158 In addition, oligomers from OCD routes have been discussed recently, and the resulting oligomers were used in epoxy resin synthesis or as adsorbents without further functionalization.149 Except for the direct use of the produced oligomers, some synthesis paths can be integrated into the depolymerization and produce phenolic foam in situ as evidenced by the work of Wang et al.159 A synergetic effect was proposed in this coprocessing that significantly inhibits lignin condensation and therefore enhanced the yields of the polymers. Xue et al. claimed that the oligomeric products from their lignin depolymerization are bio-polyols for the preparation of a bio-based rigid polyurethane foam.160 Chen et al. pointed out that the oligomeric products from their reductive depolymerization of lignin acted as the more active phenolic oil preferentially polymerized with formaldehyde compared to phenol.161 More recently, O'Dea et al. demonstrated the utility of the oligomeric products from the RCD of lignin in high-performance additive manufacturing via stereolithography 3D printing and highlighted its economic advantages over the conventional process.162
4.3 Decomposition into dicarboxylic acids or gaseous products
Valorization of oligomeric lignin products into dicarboxylic acids is another route to produce value-added chemicals. Interestingly, most related studies have used pyrolytic tar materials as the substrate for various decomposition processes. For example, Figueirêdo et al. demonstrated the concept of the ozonation of pyrolytic lignin for low molecular weight dicarboxylic acids and esters.163 In their study, the high reactivity of ozone in depolymerizing pyrolytic lignin was reported under mild conditions (0 °C, atmospheric pressure), and the optimized yield of dicarboxylic acids/esters was 45 wt% of the original pyrolytic lignin, implying the attractiveness of such a valorization route. As they pointed out, however, this approach requires a substrate that can be readily dissolved in methanol, which thus may not offer the same level of straightforwardness as any solid industrial lignin owing to its poor solubility in this solvent. However, the oligomeric products after the depolymerization could potentially serve as the ideal substrate because of the better solubility of oligomeric products in methanol.30,31,164 Additionally, the decomposition of tarry material over nickel-based catalysts has been extensively studied to improve the gas quality for biomass gasification. This offers an alternative path to utilize the oligomeric products from various lignin depolymerization processes, not limited to thermochemical conversion. According to Duc et al., enhanced depolymerization of the tar over nickel catalyst could result in a five-times increase in hydrogen gas yield after gasification.145 The tarry material in their work was just one form of oligomeric lignin being discussed in this study. Yang et al. applied the biochar-based nickel catalyst to lignin oligomers and successfully converted them into syngas with high purity.165 Therefore, it is reasonable to see this route as a promising valorization path for these oligomeric products although studies on this aspect are still very rare.
Several new ideas have recently been proposed and tested for utilizing lignin or its oligomers. For instance, Kim et al. recently reported the use of Kraft lignin as a photocatalyst that forms H2O2 by O2 reduction and H2O oxidation under visible light.166 It is, therefore, an attractive idea to implement a similar concept to the depolymerized lignin oligomer and test their performance in terms of the radical-scavenging activity and redox reactivity and to evaluate its potential as a photocatalyst as well. In addition, the low molecular weight lignin oligomers derived from solvent extraction showed high resistance to oxidative degradation, demonstrating the potential for utilizing depolymerized lignin oligomers as antioxidants.167 Another example to valorize lignin material is to use it as a battery binder, which normally requires materials that maintain a great insulation capability. This could also be a new research field to be developed for oligomeric products from lignin in the future. In addition, these aromatic bio-oligomers derived from lignin depolymerization offer many other striking properties, such as biodegradability, UV-blocking, antioxidant, and antimicrobial activities.26 Utilization that stems from any of these features could also be a promising path for the valorization of oligomeric products from lignin in the future.
5 Overview of addressing technical hurdles
The depolymerization of lignin provides access to a new dimension of the utilization of lignin-based materials. During the fractionation process, it is highly desirable to control the lignin structure, molecular weight, branching, and functional complexity to adapt lignin to various valorization processes. However, one of the biggest technical hurdles in achieving this potential is the difficulty in identifying all depolymerization products. The oligomers are indispensable pieces if one wants to gain an insightful mechanistic view of the process or to valorize them. Such difficulty could mainly be attributed to the fact that, as a natural biopolymer, the structural heterogeneity of lignin could significantly diversify the derived oligomeric products compared to the depolymerization of those petro-based polymers (e.g., polyethylene, polystyrene). Most of these oligomers are often classified as “unknown” compounds in the literature because there are no references that could be used to justify their characteristic index (e.g. retention time, chemical shift, and wavenumbers) analytically. It is, therefore, reasonable and pivotal to establish structural databases for identifying these oligomers in the future. One feasible route is to develop protocols for synthesizing various synthetic compounds as standards by the polymerization of individual monomers. Ciofi-Baffoni et al. initially successfully synthesized the β-O-4 containing oligomers to mimic the lignin structure for their enzymatic degradation tests.168 Kiyota et al. established a simple way to synthesize many lignin oligomers by mimicking the natural polymerization of lignin and used LC–MS to determine their structures and to build a library of lignin oligomer data.169 Later, Forsythe et al. developed an efficient synthetic method to prepare the model lignin hexamers and octamers containing three of the most common connectivity motifs found in native lignin (i.e., β-O-4, 5–5′, and β-5′).90 It is noteworthy that all these available standards may not well simulate the oligomeric products from lignin depolymerization because these oligomers often contain only a minor amount or none of the β-O-4, as reported in the literature.113,126,170 Therefore, more efforts should be made to establish databases specially designed for depolymerized oligomers, in which computational simulations are needed to generate hypothetical structures.
Another parallel effort should be made on lignin analytics, where major improvements in separation efficiency and fast but accurate interpretation are highly desired. The current state-of-art instruments include 2D-NMR and various high-resolution MS analyses but address the issue that the analytes cannot be well separated before the identification index is generated. The resulting structural information cannot be further translated to each compound, and more often, chromatography techniques are combined as complimentary separation processes to characterize individual oligomer. Even with more sophisticated LC–MS, it is still challenging to interpret each oligomer with high accuracy. Sometimes, one could circumvent such a dilemma if the goal is to interpret the reaction pathways. Data visualization techniques could facilitate the elucidation of the structural elements of lignin species. This could be exemplified by interpreting the FT-ICR-MS data using the concept of Kendrick mass defect plots, which provide systematic line-ups of the different lignin linkages to reveal hidden information from the MS analyses.25,137
In summary, the classical and most recent lignin depolymerization approaches are discussed in this perspective with the main emphasis on the formation, identification, and valorization of oligomeric products. Three major routes are summarized in this review to illustrate the generation of these oligomeric products from various depolymerization processes. According to the state-of-art characterization, these oligomeric products often contain minor or no aryl ether bond and are extensively present in the lignin. In addition, the molecular weight of the oligomers ranged from 250 to 1600 Da, implying a structure with up to 13 phenyl units. However, the lack of standards to identify the lignin oligomers is a challenge for the analysis of complex oligomeric products, especially when distinguishing each of the oligomers is an essential requirement. The establishment of a library for various possible lignin oligomeric structures is highly desirable.
Conflicts of interest
The authors declare no conflict of interest.
Acknowledgements
This work was part of the DOE Joint BioEnergy Institute (https://www.jbei.org) supported by the U.S. Department of Energy, Office of Science, Office of Biological and Environmental Research, through contract DE-AC02-05CH11231 between Lawrence Berkeley National Laboratory and the U.S. Department of Energy. This paper describes objective technical results and analysis. Any subjective views or opinions that might be expressed in the paper do not necessarily represent the views of the U.S. Department of Energy or the United States Government. The authors acknowledge the financial contribution from Sandia National Laboratories, which is a multi-mission laboratory managed and operated by National Technology & Engineering Solutions of Sandia, LLC, a wholly owned subsidiary of Honeywell International Inc., for the U.S. Department of Energy's National Nuclear Security Administration under contract DE-NA0003525.
References
- C. O. Tuck, E. Pérez, I. T. Horváth, R. A. Sheldon and M. Poliakoff, Valorization of biomass: Deriving more value from waste, Science, 2012, 337, 695–699 CrossRef CAS PubMed.
- Z. Sun, B. Fridrich, A. de Santi, S. Elangovan and K. Barta, Bright side of lignin depolymerization: Toward new platform chemicals, Chem. Rev., 2018, 118, 614–678 CrossRef CAS PubMed.
- C. A. E. Costa, C. A. Vega-Aguilar and A. E. Rodrigues, Added-value chemicals from lignin oxidation, Molecules, 2021, 26, 4602 CrossRef CAS PubMed.
- S. Kovács, T. Kasza, A. Thernesz, I. W. Horváth and J. Hancsók, Fuel production by hydrotreating of triglycerides on NiMo/Al2O3/F catalyst, Chem. Eng. J., 2011, 176–177, 237–243 CrossRef.
- K. Kandori, M. Oketani and M. Wakamura, Decomposition of proteins by photocatalytic Ti(IV)-doped calcium hydroxyapatite particles, Colloids Surf., B, 2013, 102, 908–914 CrossRef CAS PubMed.
- X. Huang, T. I. Koranyi, M. D. Boot and E. J. Hensen, Catalytic depolymerization of lignin in supercritical ethanol, ChemSusChem, 2014, 7, 2276–2288 CrossRef CAS PubMed.
- M. K. Chan, Q. Ye, Z. M. Png, H. N. Zeng, X. Wang and J. Xu, Valorization of lignin: Effective conversion of depolymerized lignin to oil by simple chemical modifications, Waste Biomass Valoriz., 2016, 8, 2029–2036 CrossRef.
- B. Yan, X. Lin, Z. Chen, Q. Cai and S. Zhang, Selective production of phenolic monomers via high efficient lignin depolymerization with a carbon based nickel-iron-molybdenum carbide catalyst under mild conditions, Bioresour. Technol., 2021, 321, 124503 CrossRef CAS PubMed.
- X. Liu, Z. Jiang, S. Feng, H. Zhang, J. Li and C. Hu, Catalytic depolymerization of organosolv lignin to phenolic monomers and low molecular weight oligomers, Fuel, 2019, 244, 247–257 CrossRef CAS.
- A. Tribot, G. Amer, M. A. Alio, H. de Baynast, C. Delattre, A. Pons, J.-D. Mathias, J.-M. Callois, C. Vial, P. Michaud and C.-G. Dussap, Wood-lignin: Supply, extraction processes and use as bio-based material, Eur. Polym. J., 2019, 112, 228–240 CrossRef CAS.
- A. Das, A. Rahimi, A. Ulbrich, M. Alherech, A. H. Motagamwala, A. Bhalla, L. da Costa Sousa, V. Balan, J. A. Dumesic, E. L. Hegg, B. E. Dale, J. Ralph, J. J. Coon and S. S. Stahl, Lignin conversion to low-molecular-weight aromatics via an aerobic oxidation-hydrolysis sequence: Comparison of different lignin sources, ACS Sustainable Chem. Eng., 2018, 6, 3367–3374 CrossRef CAS.
- R. Ma, Y. Xu and X. Zhang, Catalytic oxidation of biorefinery lignin to value-added chemicals to support sustainable biofuel production, ChemSusChem, 2015, 8, 24–51 CrossRef CAS PubMed.
- S. Kumaravel, P. Thiruvengetam, K. Karthick, S. S. Sankar, A. Karmakar and S. Kundu, Green and sustainable route for oxidative depolymerization of lignin: New platform for fine chemicals and fuels, Biotechnol. Prog., 2021, 37, e3111 CrossRef CAS PubMed.
- C. Liu, S. Wu, H. Zhang and R. Xiao, Catalytic oxidation of lignin to valuable biomass-based platform chemicals: A review, Fuel Process. Technol., 2019, 191, 181–201 CrossRef CAS.
- C. Cheng, J. Wang, D. Shen, J. Xue, S. Guan, S. Gu and K. Luo, Catalytic oxidation of lignin in solvent systems for production of renewable chemicals: A review, Polymers, 2017, 9, 240 CrossRef PubMed.
- J. D. P. Araújo, C. A. Grande and A. E. Rodrigues, Vanillin production from lignin oxidation in a batch reactor, Chem. Eng. Res. Des., 2010, 88, 1024–1032 CrossRef.
- K. Yamamoto, T. Hosoya, K. Yoshioka, H. Miyafuji, H. Ohno and T. Yamada, Tetrabutylammonium hydroxide 30-hydrate as novel reaction medium for lignin conversion, ACS Sustainable Chem. Eng., 2017, 5, 10111–10115 CrossRef CAS.
- M. Fache, B. Boutevin and S. Caillol, Vanillin production from lignin and its use as a renewable chemical, ACS Sustainable Chem. Eng., 2015, 4, 35–46 CrossRef.
- S. Van den Bosch, W. Schutyser, R. Vanholme, T. Driessen, S. F. Koelewijn, T. Renders, B. De Meester, W. J. J. Huijgen, W. Dehaen, C. M. Courtin, B. Lagrain, W. Boerjan and B. F. Sels, Reductive lignocellulose fractionation into soluble lignin-derived phenolic monomers and dimers and processable carbohydrate pulps, Energy Environ. Sci., 2015, 8, 1748–1763 RSC.
- S. K. Singh and J. D. Ekhe, Towards effective lignin conversion: HZSM-5 catalyzed one-pot solvolytic depolymerization/hydrodeoxygenation of lignin into value added compounds, RSC Adv., 2014, 4, 27971–27978 RSC.
- I. Klein, B. Saha and M. M. Abu-Omar, Lignin depolymerization over Ni/C catalyst in methanol, a continuation: Effect of substrate and catalyst loading, Catal. Sci. Technol., 2015, 5, 3242–3245 RSC.
- F. Stankovikj, A. G. McDonald, G. L. Helms and M. Garcia-Perez, Quantification of bio-Oil functional groups and evidences of the presence of pyrolytic humins, Energy Fuels, 2016, 30, 6505–6524 CrossRef.
- I. Hita, T. Cordero-Lanzac, T. Kekäläinen, O. Okafor, J. Rodríguez-Mirasol, T. Cordero, J. Bilbao, J. Jänis and P. Castano, In-depth analysis of raw bio-Oil and its hydrodeoxygenated products for a comprehensive catalyst performance evaluation, ACS Sustainable Chem. Eng., 2020, 8, 18433–18445 CrossRef CAS.
- Q. Song, F. Wang, J. Cai, Y. Wang, J. Zhang, W. Yu and J. Xu, Lignin depolymerization (LDP) in alcohol over nickel-based catalysts via a fragmentation–hydrogenolysis process, Energy Environ. Sci., 2013, 6, 994–1007 RSC.
- E. Terrell, V. Carré, A. Dufour, F. Aubriet, Y. Le Brech and M. Garcia-Pérez, Contributions to lignomics: stochastic generation of oligomeric lignin structures for interpretation of MALDI–FT-ICR-MS results, ChemSusChem, 2020, 13, 4428–4445 CrossRef CAS PubMed.
- M. Galkin, From stabilization strategies to tailor-made lignin macromolecules and oligomers for materials, Curr. Opin. Green Sustainable Chem., 2021, 28, 100438 CrossRef CAS.
- H. Dao Thi, K. Van Aelst, S. Van den Bosch, R. Katahira, G. T. Beckham, B. F. Sels and K. M. Van Geem, Identification and quantification of lignin monomers and oligomers from reductive catalytic fractionation of pine wood with GC × GC – FID/MS, Green Chem., 2022, 24, 191–206 RSC.
- J. Li, G. Henriksson and G. Gellerstedt, Lignin depolymerization/repolymerization and its critical role for delignification of aspen wood by steam explosion, Bioresour. Technol., 2007, 98, 3061–3068 CrossRef CAS PubMed.
- K. H. Kim and C. S. Kim, Recent efforts to prevent undesirable reactions from fractionation to depolymerization of lignin: Toward maximizing the value from lignin, Front. Energy Res., 2018, 6, 92 CrossRef.
- W. Schutyser, S. Van den Bosch, T. Renders, T. De Boe, S. F. Koelewijn, A. Dewaele, T. Ennaert, O. Verkinderen, B. Goderis, C. M. Courtin and B. F. Sels, Influence of bio-based solvents on the catalytic reductive fractionation of birch wood, Green Chem., 2015, 17, 5035–5045 RSC.
- E. M. Anderson, R. Katahira, M. Reed, M. G. Resch, E. M. Karp, G. T. Beckham and Y. Román-Leshkov, Reductive catalytic fractionation of corn stover lignin, ACS
Sustainable Chem. Eng., 2016, 4, 6940–6950 CrossRef CAS.
- H. Luo, E. P. Weeda, M. Alherech, C. W. Anson, S. D. Karlen, Y. Cui, C. E. Foster and S. S. Stahl, Oxidative catalytic fractionation of lignocellulosic biomass under non-alkaline conditions, J. Am. Chem. Soc., 2021, 143, 15462–15470 CrossRef CAS PubMed.
- J. Mottweiler, M. Puche, C. Rauber, T. Schmidt, P. Concepcion, A. Corma and C. Bolm, Copper- and vanadium-catalyzed oxidative cleavage of lignin using dioxygen, ChemSusChem, 2015, 8, 2106–2113 CrossRef CAS PubMed.
- Z. Zhang, P. Gogoi, Z. Geng, X. Liu and X. Du, Low temperature lignin depolymerization to aromatic compounds with a redox couple catalyst, Fuel, 2020, 281, 118799 CrossRef CAS.
- X. Lin, S. Sui, S. Tan, C. Pittman, J. Sun and Z. Zhang, Fast pyrolysis of four lignins from different isolation processes using Py-GC/MS, Energies, 2015, 8, 5107–5121 CrossRef CAS.
- J. O. Ighalo, F. U. Iwuchukwu, O. E. Eyankware, K. O. Iwuozor, K. Olotu, O. C. Bright and C. A. Igwegbe, Flash pyrolysis of biomass: A review of recent advances, Clean Technol. Environ. Policy, 2022, 24, 2349–2363 CrossRef CAS.
- J. Ralph, K. Lundquist, G. Brunow, F. Lu, H. Kim, P. F. Schatz, J. M. Marita, R. D. Hatfield, S. A. Ralph, J. H. Christensen and W. Boerjan, Lignins: Natural polymers from oxidative coupling of 4-hydroxyphenyl-propanoids, Phytochem. Rev., 2004, 3, 29–60 CrossRef CAS.
- R. Vanholme, B. Demedts, K. Morreel, J. Ralph and W. Boerjan, Lignin biosynthesis and structure, Plant Physiol., 2010, 153, 895–905 CrossRef CAS PubMed.
- X. Huang, T. I. Korányi, M. D. Boot and E. J. M. Hensen, Catalytic depolymerization of lignin in supercritical ethanol, ChemSusChem, 2014, 7, 2276–2288 CrossRef CAS PubMed.
- Y. Jiang, Z. Li, X. Tang, Y. Sun, X. Zeng, S. Liu and L. Lin, Depolymerization of cellulolytic enzyme lignin for the production of monomeric phenols over RANEY® Ni and acidic zeolite catalysts, Energy Fuels, 2015, 29, 1662–1668 CrossRef CAS.
- H. Konnerth, J. Zhang, D. Ma, M. H. G. Prechtl and N. Yan, Base promoted hydrogenolysis of lignin model compounds and organosolv lignin over metal catalysts in water, Chem. Eng. Sci., 2015, 123, 155–163 CrossRef CAS.
- F. Gao, J. D. Webb, H. Sorek, D. E. Wemmer and J. F. Hartwig, Fragmentation of lignin samples with commercial Pd/C under ambient pressure of hydrogen, ACS Catal., 2016, 6, 7385–7392 CrossRef CAS.
- T. Parsell, S. Yohe, J. Degenstein, T. Jarrell, I. Klein, E. Gencer, B. Hewetson, M. Hurt, J. I. Kim, H. Choudhari, B. Saha, R. Meilan, N. Mosier, F. Ribeiro, W. N. Delgass, C. Chapple, H. I. Kenttämaa, R. Agrawal and M. M. Abu-Omar, A synergistic biorefinery based on catalytic conversion of lignin prior to cellulose starting from lignocellulosic biomass, Green Chem., 2015, 17, 1492–1499 RSC.
- H. Luo, I. M. Klein, Y. Jiang, H. Zhu, B. Liu, H. I. Kenttämaa and M. M. Abu-Omar, Total utilization of Miscanthus biomass, lignin and carbohydrates, using earth abundant nickel catalyst, ACS Sustainable Chem. Eng., 2016, 4, 2316–2322 CrossRef CAS.
- J. Chen, F. Lu, X. Si, X. Nie, J. Chen, R. Lu and J. Xu, High yield production of natural phenolic alcohols from woody biomass using a Nickel-based catalyst, ChemSusChem, 2016, 9, 3353–3360 CrossRef CAS PubMed.
- T. Renders, S. Van den Bosch, T. Vangeel, T. Ennaert, S.-F. Koelewijn, G. Van den Bossche, C. M. Courtin, W. Schutyser and B. F. Sels, Synergetic effects of alcohol/water mixing on the catalytic reductive fractionation of poplar wood, ACS Sustainable Chem. Eng., 2016, 4, 6894–6904 CrossRef CAS.
- X. Huang, O. M. Morales Gonzalez, J. Zhu, T. I. Korányi, M. D. Boot and E. J. M. Hensen, Reductive fractionation of woody biomass into lignin monomers and cellulose by tandem metal triflate and Pd/C catalysis, Green Chem., 2017, 19, 175–187 RSC.
- Y. Zhai, C. Li, G. Xu, Y. Ma, X. Liu and Y. Zhang, Depolymerization of lignin via a non-precious Ni–Fe alloy catalyst supported on activated carbon, Green Chem., 2017, 19, 1895–1903 RSC.
- S. Van den Bosch, T. Renders, S. Kennis, S. F. Koelewijn, G. Van den Bossche, T. Vangeel, A. Deneyer, D. Depuydt, C. M. Courtin, J. M. Thevelein, W. Schutyser and B. F. Sels, Integrating lignin valorization and bio-ethanol production: On the role of Ni-Al2O3 catalyst pellets during lignin-first fractionation, Green Chem., 2017, 19, 3313–3326 RSC.
- I. Kumaniaev, E. Subbotina, J. Sävmarker, M. Larhed, M. V. Galkin and J. S. M. Samec, Lignin depolymerization to monophenolic compounds in a flow-through system, Green Chem., 2017, 19, 5767–5771 RSC.
- Y. Shao, Q. Xia, L. Dong, X. Liu, X. Han, S. F. Parker, Y. Cheng, L. L. Daemen, A. J. Ramirez-Cuesta, S. Yang and Y. Wang, Selective production of arenes via direct lignin upgrading over a niobium-based catalyst, Nat. Commun., 2017, 8, 16104 CrossRef PubMed.
- L.-P. Xiao, S. Wang, H. Li, Z. Li, Z.-J. Shi, L. Xiao, R.-C. Sun, Y. Fang and G. Song, Catalytic hydrogenolysis of lignins into phenolic compounds over carbon nanotube supported molybdenum oxide, ACS Catal., 2017, 7, 7535–7542 CrossRef CAS.
- E. M. Anderson, M. L. Stone, M. J. Hülsey, G. T. Beckham and Y. Román-Leshkov, Kinetic studies of lignin solvolysis and reduction by reductive catalytic fractionation decoupled in flow-through reactors, ACS Sustainable Chem. Eng., 2018, 6, 7951–7959 CrossRef CAS.
- X. Ouyang, X. Huang, J. Zhu, M. D. Boot and E. J. M. Hensen, Catalytic conversion of lignin in woody biomass into phenolic monomers in methanol/water mixtures without external hydrogen, ACS Sustainable Chem. Eng., 2019, 7, 13764–13773 CrossRef CAS.
- S. Wang, W.-X. Li, Y.-Q. Yang, X. Chen, J. Ma, C. Chen, L.-P. Xiao and R.-C. Sun, Unlocking structure–reactivity relationships for catalytic hydrogenolysis of lignin into phenolic monomers, ChemSusChem, 2020, 13, 4548–4556 CrossRef CAS PubMed.
- Y. Wu, Z. Lin, X. Zhu, X. Hu, M. Gholizadeh, H. Sun, Y. Huang, S. Zhang and H. Zhang, Hydrogenolysis of lignin to phenolic monomers over Ru based catalysts with different metal-support interactions: Effect of partial hydrogenation of C(sp2)-O/C, Fuel, 2021, 302, 121184 CrossRef CAS.
- Z. Dou, Z. Zhang and M. Wang, Self-hydrogen transfer hydrogenolysis of native lignin over Pd-PdO/TiO2, Appl. Catal., B, 2022, 301, 120767 CrossRef CAS.
- B. Luo, L. Zhou, Z. Tian, Y. He and R. Shu, Hydrogenolysis
of cornstalk lignin in supercritical ethanol over N-doped micro-mesoporous biochar supported Ru catalyst, Fuel Process. Technol., 2022, 231, 107218 CrossRef CAS.
- H. Deng, L. Lin, Y. Sun, C. Pang, J. Zhuang, P. Ouyang, J. Li and S. Liu, Activity and stability of perovskite-type oxide LaCoO3 catalyst in lignin catalytic wet oxidation to aromatic aldehydes process, Energy Fuels, 2009, 23, 19–24 CrossRef CAS.
- K. Stärk, N. Taccardi, A. Bösmann and P. Wasserscheid, Oxidative depolymerization of lignin in ionic liquids, ChemSusChem, 2010, 3, 719–723 CrossRef PubMed.
- S. Liu, Z. Shi, L. Li, S. Yu, C. Xie and Z. Song, Process of lignin oxidation in an ionic liquid coupled with separation, RSC Adv., 2013, 3, 5789–5793 RSC.
- W. Schutyser, J. S. Kruger, A. M. Robinson, R. Katahira, D. G. Brandner, N. S. Cleveland, A. Mittal, D. J. Peterson, R. Meilan, Y. Román-Leshkov and G. T. Beckham, Revisiting alkaline aerobic lignin oxidation, Green Chem., 2018, 20, 3828–3844 RSC.
- P. Hafezisefat, J. K. Lindstrom, R. C. Brown and L. Qi, Non-catalytic oxidative depolymerization of lignin in perfluorodecalin to produce phenolic monomers, Green Chem., 2020, 22, 6567–6578 RSC.
- Y. Zhao, Q. Xu, T. Pan, Y. Zuo, Y. Fu and Q.-X. Guo, Depolymerization of lignin by catalytic oxidation with aqueous polyoxometalates, Appl. Catal., A, 2013, 467, 504–508 CrossRef CAS.
- X. Du, A. W. Tricker, W. Yang, R. Katahira, W. Liu, T. T. Kwok, P. Gogoi and Y. Deng, Oxidative catalytic fractionation and depolymerization of lignin in a one-pot single-catalyst system, ACS Sustainable Chem. Eng., 2021, 9, 7719–7727 CrossRef CAS.
- I. Hasegawa, Y. Inoue, Y. Muranaka, T. Yasukawa and K. Mae, Selective production of organic acids and depolymerization of lignin by hydrothermal oxidation with diluted hydrogen peroxide, Energy Fuels, 2011, 25, 791–796 CrossRef CAS.
- B. Du, B. Liu, X. Wang and J. Zhou, A comparison of phenolic monomers produced from different types of lignin by phosphotungstic acid catalysts, ChemistryOpen, 2019, 8, 643–649 CrossRef CAS PubMed.
- H. Gao, J. Wang, M. Liu, S. Wang, W. Li, Q. An, K. Li, L. Wei, C. Han and S. Zhai, Enhanced oxidative depolymerization of lignin in cooperative imidazolium-based ionic liquid binary mixtures, Bioresour. Technol., 2022, 357, 127333 CrossRef CAS PubMed.
- W. Jeon, I.-H. Choi, J.-Y. Park, J.-S. Lee and K.-R. Hwang, Alkaline wet oxidation of lignin over Cu-Mn mixed oxide catalysts for production of vanillin, Catal. Today, 2020, 352, 95–103 CrossRef CAS.
- X. Ouyang, T. Ruan and X. Qiu, Effect of solvent on hydrothermal oxidation depolymerization of lignin for the production of monophenolic compounds, Fuel Process. Technol., 2016, 144, 181–185 CrossRef CAS.
- M. Alherech, S. Omolabake, C. M. Holland, G. E. Klinger, E. L. Hegg and S. S. Stahl, From lignin to valuable aromatic chemicals: Lignin depolymerization and monomer separation via centrifugal partition chromatography, ACS Cent. Sci., 2021, 7, 1831–1837 CrossRef CAS PubMed.
-
J. G. Speight, in Handbook of industrial hydrocarbon processes (Second Edition), ed. J. G. Speight, Gulf Professional Publishing, Boston, 2nd edn, 2020, pp. 95–142, DOI:10.1016/B978-0-12-809923-0.00003-5.
- P. Šimáček and D. Kubička, Hydrocracking of petroleum vacuum distillate containing rapeseed oil: Evaluation of diesel fuel, Fuel, 2010, 89, 1508–1513 CrossRef.
- J. Weitkamp, Catalytic hydrocracking—Mechanisms and versatility
of the process, ChemCatChem, 2012, 4, 292–306 CrossRef CAS.
- S. Sartipi, M. Makkee, F. Kapteijn and J. Gascon, Catalysis engineering of bifunctional solids for the one-step synthesis of liquid fuels from syngas: A review, Catal. Sci. Technol., 2014, 4, 893–907 RSC.
- J. Rey, C. Bignaud, P. Raybaud, T. Bučko and C. Chizallet, Dynamic features of transition states for β-scission reactions of alkenes over acid zeolites revealed by AIMD simulations, Angew. Chem., Int. Ed, 2020, 59, 18938–18942 CrossRef CAS PubMed.
- M. V. Frash, V. B. Kazansky, A. M. Rigby and R. A. van Santen, Cracking of hydrocarbons on zeolite catalysts: Density functional and Hartree–Fock Calculations on the mechanism of the β-scission reaction, J. Phys. Chem. B, 1998, 102, 2232–2238 CrossRef CAS.
- R. J. A. Gosselink, A. Abächerli, H. Semke, R. Malherbe, P. Käuper, A. Nadif and J. E. G. van Dam, Analytical protocols for characterisation of sulphur-free lignin, Ind. Crops Prod., 2004, 19, 271–281 CrossRef CAS.
- A. Rahimi, A. Azarpira, H. Kim, J. Ralph and S. S. Stahl, Chemoselective metal-free aerobic alcohol oxidation in lignin, J. Am. Chem. Soc., 2013, 135, 6415–6418 CrossRef CAS PubMed.
- J. M. Nichols, L. M. Bishop, R. G. Bergman and J. A. Ellman, Catalytic C–O bond cleavage of 2-aryloxy-1-arylethanols and its application to the depolymerization of lignin-related polymers, J. Am. Chem. Soc., 2010, 132, 12554–12555 CrossRef CAS PubMed.
- T. vom Stein, T. den Hartog, J. Buendia, S. Stoychev, J. Mottweiler, C. Bolm, J. Klankermayer and W. Leitner, Ruthenium-catalyzed C-C bond cleavage in lignin model substrates, Angew. Chem., Int. Ed., 2015, 54, 5859–5863 CrossRef CAS PubMed.
- Y. Liu, C. Li, W. Miao, W. Tang, D. Xue, C. Li, B. Zhang, J. Xiao, A. Wang, T. Zhang and C. Wang, Mild redox-neutral depolymerization of lignin with a binuclear Rh complex in water, ACS Catal., 2019, 9, 4441–4447 CrossRef CAS.
- Y. Liu, C. Li, W. Miao, W. Tang, D. Xue, J. Xiao, T. Zhang and C. Wang, Rhodium-terpyridine catalyzed redox-neutral depolymerization of lignin in water, Green Chem., 2020, 22, 33–38 RSC.
- H. Kawamoto, S. Horigoshi and S. Saka, Pyrolysis reactions of various lignin model dimers, J. Wood Sci., 2007, 53, 168–174 CrossRef CAS.
- S. P. Mun and C. S. Ku, Pyrolysis GC-MS analysis of tars formed during the aging of wood and bamboo crude vinegars, J. Wood Sci., 2009, 56, 47–52 CrossRef.
- T. Hosoya, H. Kawamoto and S. Saka, Secondary reactions of lignin-derived primary tar components, J. Anal. Appl. Pyrolysis, 2008, 83, 78–87 CrossRef CAS.
- M. Asmadi, H. Kawamoto and S. Saka, Thermal reactions of guaiacol and syringol as lignin model aromatic nuclei, J. Anal. Appl. Pyrolysis, 2011, 92, 88–98 CrossRef CAS.
- M. B. Figueirêdo, Z. Jotic, P. J. Deuss, R. H. Venderbosch and H. J. Heeres, Hydrotreatment of pyrolytic lignins to aromatics and phenolics using heterogeneous catalysts, Fuel Process. Technol., 2019, 189, 28–38 CrossRef.
- R. Bayerbach and D. Meier, Characterization of the water-insoluble fraction from fast pyrolysis liquids (pyrolytic lignin). Part IV: Structure elucidation of oligomeric molecules, J. Anal. Appl. Pyrolysis, 2009, 85, 98–107 CrossRef CAS.
- W. G. Forsythe, M. D. Garrett, C. Hardacre, M. Nieuwenhuyzen and G. N. Sheldrake, An efficient and flexible synthesis of model lignin oligomers, Green Chem., 2013, 15, 3031–3038 RSC.
- R. Zhang, Y. Qi, C. Ma, J. Ge, Q. Hu, F. J. Yue, S. L. Li and D. A. Volmer, Characterization of lignin compounds at the molecular level: Mass spectrometry analysis and raw data processing, Molecules, 2021, 26, 178 CrossRef CAS PubMed.
- J. S. Lupoi, S. Singh, R. Parthasarathi, B. A. Simmons and R. J. Henry, Recent innovations in analytical methods for the qualitative and quantitative assessment of lignin, Renewable Sustainable Energy Rev., 2015, 49, 871–906 CrossRef CAS.
- J. Hertzog, C. Mase, M. Hubert-Roux, C. Afonso, P. Giusti and C. Barrère-Mangote, Characterization of heavy products from lignocellulosic biomass pyrolysis by chromatography and fourier transform mass spectrometry: A review, Energy Fuels, 2021, 35, 17979–18007 CrossRef CAS.
- M. Sun, M. Sandahl and C. Turner, Comprehensive on-line two-dimensional liquid chromatography × supercritical fluid chromatography with trapping column-assisted modulation for depolymerised lignin analysis, J. Chromatogr. A, 2018, 1541, 21–30 CrossRef CAS PubMed.
- D. Papp, T. Rukkijakan, D. Lebedeva, T. Nylander, M. Sandahl, J. S. M. Samec and C. Turner, Single-standard quantification strategy for lignin dimers by supercritical fluid chromatography with charged aerosol detection, Anal. Chem., 2023, 95, 1436–1445 CAS.
- B. Joffres, C. Lorentz, M. Vidalie, D. Laurenti, A. A. Quoineaud, N. Charon, A. Daudin, A. Quignard and C. Geantet, Catalytic hydroconversion of a wheat straw soda lignin: Characterization of the products and the lignin residue, Appl. Catal., B, 2014, 145, 167–176 CrossRef CAS.
- P. Ferrini and R. Rinaldi, Catalytic biorefining of plant biomass to non-pyrolytic lignin bio-oil and carbohydrates through hydrogen transfer reactions, Angew. Chem., Int. Ed., 2014, 53, 8634–8639 CrossRef CAS PubMed.
- H. Ben and A. J. Ragauskas, One step thermal conversion of lignin to the gasoline range liquid products by using zeolites as additives, RSC Adv., 2012, 2, 12892–12898 RSC.
- W. Yin, R. H. Venderbosch, S. He, M. V. Bykova, S. A. Khromova, V. A. Yakovlev and H. J. Heeres, Mono-, bi-, and tri-metallic Ni-based catalysts for the catalytic hydrotreatment of pyrolysis liquids, Biomass Convers. Biorefin., 2017, 7, 361–376 CrossRef CAS.
- R. J. M. Westerhof, S. R. G. Oudenhoven, X. Hu, H. J. Heeres, C.-Z. Li, M. Garcia-Perez and S. R. A. Kersten, Biofuel and methyl levulinate from biomass-derived fractional condensed pyrolysis oil and alcohol, Energy Technol., 2017, 5, 205–215 CrossRef CAS.
- F. de Miguel Mercader, M. J. Groeneveld, S. R. A. Kersten, C. Geantet, G. Toussaint, N. W. J. Way, C. J. Schaverien and K. J. A. Hogendoorn, Hydrodeoxygenation of pyrolysis oil fractions: process understanding and quality assessment through co-processing in refinery units, Energy Environ. Sci., 2011, 4, 985–997 RSC.
- A. A. Andrianova, N. A. Yeudakimenka, S. L. Lilak, E. I. Kozliak, A. Ugrinov, M. P. Sibi and A. Kubátová, Size exclusion chromatography of lignin: The mechanistic aspects and elimination of undesired secondary interactions, J. Chromatogr. A, 2018, 1534, 101–110 CrossRef CAS PubMed.
-
B. Hortling, E. Turunen and P. Kokkonen, Molar mass and size distribution of lignins, in Handbook Of Size Exclusion Chromatography And Related Techniques: Revised And Expanded, 2003, vol. 91, p. 359 Search PubMed.
- A. J. Ragauskas, C. K. Williams, B. H. Davison, G. Britovsek, J. Cairney, C. A. Eckert, W. J. Frederick, J. P. Hallett, D. J. Leak, C. L. Liotta, J. R. Mielenz, R. Murphy, R. Templer and T. Tschaplinski, The path forward for biofuels and biomaterials, Science, 2006, 311, 484–489 CrossRef CAS PubMed.
- J. Banoub, G.-H. Delmas Jr., N. Joly, G. Mackenzie, N. Cachet, B. Benjelloun-Mlayah and M. Delmas, A critique on the structural analysis of lignins and application of novel tandem mass spectrometric strategies to determine lignin sequencing, J. Mass Spectrom., 2015, 50, 5–48 CrossRef CAS PubMed.
- K. B. H. Finch, R. M. Richards, A. Richel, A. V. Medvedovici, N. G. Gheorghe, M. Verziu, S. M. Coman and V. I. Parvulescu, Catalytic hydroprocessing of lignin under thermal and ultrasound conditions, Catal. Today, 2012, 196, 3–10 CrossRef CAS.
- Y. Han, D. N. McIlroy and A. G. McDonald, Hydrodeoxygenation of pyrolysis oil for hydrocarbon production using nanospring based catalysts, J. Anal. Appl. Pyrolysis, 2016, 117, 94–105 CrossRef CAS.
- F. Stankovikj, A. G. McDonald, G. L. Helms, M. V. Olarte and M. Garcia-Perez, Characterization of the water-soluble fraction of woody biomass pyrolysis oils, Energy Fuels, 2017, 31, 1650–1664 CrossRef CAS.
- X. Kong, C. Liu, X. Wang, Y. Fan, W. Xu and R. Xiao, Production of oxygen-containing fuels via supercritical methanol hydrodeoxygenation of lignin bio-oil over Cu/CuZnAlOx catalyst, Appl. Energy, 2022, 316, 119129 CrossRef CAS.
- H. Paananen, E. Eronen, M. Mäkinen, J. Jänis, M. Suvanto and T. T. Pakkanen, Base-catalyzed oxidative depolymerization of softwood kraft lignin, Ind. Crops Prod., 2020, 152, 112473 CrossRef CAS.
- L. Xiao, S. Hu, H. Han, Q. Ren, L. He, L. Jiang, S. Su, Y. Wang and J. Xiang, An insight into the OPAHs and SPAHs formation mechanisms during alkaline lignin pyrolysis at different temperatures, J. Anal. Appl. Pyrolysis, 2021, 156, 105104 CrossRef CAS.
- D. J. McClelland, P. H. Galebach, A. H. Motagamwala, A. M. Wittrig, S. D. Karlen, J. S. Buchanan, J. A. Dumesic and G. W. Huber, Supercritical methanol depolymerization and hydrodeoxygenation of lignin and biomass over reduced copper porous metal oxides, Green Chem., 2019, 21, 2988–3005 RSC.
- H. Zhang and S. Fu, Highly efficient catalytic transfer hydrogenolysis for the conversion of Kraft lignin into bio-oil over heteropoly acids, Green Chem., 2022, 24, 6619–6630 RSC.
- Q. Liu, P. Li, N. Liu and D. Shen, Lignin depolymerization to aromatic monomers and oligomers in isopropanol assisted by microwave heating, Polym. Degrad. Stab., 2017, 135, 54–60 CrossRef CAS.
- M. Staš, J. Chudoba, D. Kubička and M. Pospíšil, Chemical characterization of pyrolysis bio-oil: Application of orbitrap mass spectrometry, Energy Fuels, 2015, 29, 3233–3240 CrossRef.
- J. Dai, G. N. Styles, A. F. Patti and K. Saito, CuSO4/H2O2-catalyzed lignin depolymerization under the irradiation of microwaves, ACS Omega, 2018, 3, 10433–10441 CrossRef CAS PubMed.
- D. J. McClelland, A. H. Motagamwala, Y. Li, M. R. Rover, A. M. Wittrig, C. Wu, J. S. Buchanan, R. C. Brown, J. Ralph, J. A. Dumesic and G. W. Huber, Functionality and molecular weight distribution of red oak lignin before and after pyrolysis and hydrogenation, Green Chem., 2017, 19, 1378–1389 RSC.
- X. Si, J. Chen, F. Lu, X. Liu, Y. Ren, R. Lu, H. Jiang, H. Liu, S. Miao, Y. Zhu, X. Luo and J. Xu, Immobilized Ni clusters in mesoporous aluminum silica nanospheres for catalytic hydrogenolysis of lignin, ACS Sustainable Chem. Eng., 2019, 7, 19034–19041 CrossRef CAS.
- A. Agarwal, Y.-T. Jo and J.-H. Park, Hybrid microwave-ultrasound assisted catalyst-free depolymerization of Kraft lignin to bio-oil, Ind. Crops Prod., 2021, 162, 113300 CrossRef CAS.
- L. Das, M. Li, J. Stevens, W. Li, Y. Pu, A. J. Ragauskas and J. Shi, Characterization and catalytic transfer hydrogenolysis of deep eutectic solvent extracted sorghum lignin to phenolic compounds, ACS Sustainable Chem. Eng., 2018, 6, 10408–10420 CrossRef CAS.
- X. Kong, C. Liu, Y. Fan, W. Xu and R. Xiao, Production of jet fuel precursors from waste kraft lignin with a complex copper acid catalyst, ACS Sustainable Chem. Eng., 2021, 10, 495–507 CrossRef.
- M. B. Figueirêdo, R. H. Venderbosch, H. J. Heeres and P. J. Deuss, In-depth structural characterization of the lignin fraction of a pine-derived pyrolysis oil, J. Anal. Appl. Pyrolysis, 2020, 149, 104837 CrossRef.
- S. Li, Z. Luo, W. Wang, K. Lu, Y. Yang and X. Liang, Characterization of pyrolytic lignin and insight into its formation mechanisms using novel techniques and DFT method, Fuel, 2020, 262, 116516 CrossRef CAS.
- M. R. Rover, P. A. Johnston, L. E. Whitmer, R. G. Smith and R. C. Brown, The effect of pyrolysis temperature on recovery of bio-oil as distinctive stage fractions, J. Anal. Appl. Pyrolysis, 2014, 105, 262–268 CrossRef CAS.
- W. Chen, D. J. McClelland, A. Azarpira, J. Ralph, Z. Luo and G. W. Huber, Low temperature hydrogenation of pyrolytic lignin over Ru/TiO2: 2D HSQC and 13C NMR study of reactants and products, Green Chem., 2016, 18, 271–281 RSC.
- C. Mattsson, S.-I. Andersson, T. Belkheiri, L.-E. Åmand, L. Olausson, L. Vamling and H. Theliander, Using 2D NMR to characterize the structure of the low and high molecular weight fractions of bio-oil obtained from LignoBoost™ kraft lignin depolymerized in subcritical water, Biomass Bioenergy, 2016, 95, 364–377 CrossRef CAS.
- S. Heikkinen, M. M. Toikka, P. T. Karhunen and I. A. Kilpeläinen, Quantitative 2D HSQC (Q-HSQC) via suppression of J-dependence of polarization transfer in NMR spectroscopy: Application to wood lignin, J. Am. Chem. Soc., 2003, 125, 4362–4367 CrossRef CAS PubMed.
- M. Huang, J. Xu, Z. Ma, Y. Yang, B. Zhou, C. Wu, J. Ye, C. Zhao, X. Liu, D. Chen and W. Zhang, Bio-BTX production from the shape selective catalytic fast pyrolysis of lignin using different zeolite catalysts: Relevance between the chemical structure and the yield of bio-BTX, Fuel Process. Technol., 2021, 216, 106792 CrossRef CAS.
- M. Sette, R. Wechselberger and C. Crestini, Elucidation of lignin structure by quantitative 2D NMR, Chem. – Eur. J., 2011, 17, 9529–9535 CrossRef CAS PubMed.
- Z. Wang and P. J. Deuss, Catalytic hydrogenolysis of lignin: The influence of minor units and saccharides, ChemSusChem, 2021, 14, 5186–5198 CrossRef CAS PubMed.
- T. Ren, S. You, M. Zhang, Y. Wang, W. Qi, R. Su and Z. He, Improved conversion efficiency of Lignin-to-Fuel conversion by limiting catalyst deactivation, Chem. Eng. J., 2021, 410, 128270 CrossRef CAS.
- A. Izumi and K.-I. Kuroda, Pyrolysis-mass spectrometry analysis of dehydrogenation lignin polymers with various syringyl/guaiacyl ratios, Rapid Commun. Mass Spectrom., 1997, 11, 1709–1715 CrossRef CAS.
- X. Ren, P. Wang, X. Han, G. Zhang, J. Gu, C. Ding, X. Zheng and F. Cao, Depolymerization of lignin to aromatics by selectively oxidizing cleavage of C–C and C–O bonds using CuCl2/polybenzoxazine catalysts at room temperature, ACS Sustainable Chem. Eng., 2017, 5, 6548–6556 CrossRef CAS.
- H. Guo, Z. Qi, Y. Liu, H. Xia, L. Li, Q. Huang, A. Wang and C. Li, Tungsten-based catalysts for lignin depolymerization: the role of tungsten species in C–O bond cleavage, Catal. Sci. Technol., 2019, 9, 2144–2151 RSC.
- S. Wan, T. Pham, S. Zhang, L. Lobban, D. Resasco and R. Mallinson, Direct catalytic upgrading of biomass pyrolysis vapors by a dual function Ru/TiO2catalyst, AIChE J., 2013, 59, 2275–2285 CrossRef CAS.
- J. O. Strüven and D. Meier, Hydrocracking of organosolv lignin in subcritical water to useful phenols employing various RANEY® nickel catalysts, ACS Sustainable Chem. Eng., 2016, 4, 3712–3721 CrossRef.
- E. Terrell and M. Garcia-Perez, Novel strategy to analyze fourier transform ion cyclotron resonance mass spectrometry data of biomass pyrolysis oil for oligomeric structure assignment, Energy Fuels, 2020, 34, 8466–8481 CrossRef CAS.
- Y. Han, A. P. P. Pires, M. Denson, A. G. McDonald and M. Garcia-Perez, Ternary phase diagram of water/bio-oil/organic solvent for bio-oil fractionation, Energy Fuels, 2020, 34, 16250–16264 CrossRef CAS.
- J. Li, P. H. Galebach, J. K. Johnson, T. Fredriksen, A. Wittrig, X. Bai, H. Yang and G. W. Huber, Supercritical methanol depolymerization and hydrodeoxygenation of pyrolytic lignin over reduced copper porous metal oxides, Green Chem., 2020, 22, 8403–8413 RSC.
- M. Balakshin and E. Capanema, On the quantification of lignin hydroxyl groups with 31P and 13C NMR spectroscopy, J. Wood Chem. Technol., 2015, 35, 220–237 CrossRef CAS.
- C. Peng, Q. Chen, H. Guo, G. Hu, C. Li, J. Wen, H. Wang, T. Zhang, Z. K. Zhao, R. Sun and H. Xie, Effects of extraction methods on structure and valorization of corn stover lignin by a Pd/C catalyst, ChemCatChem, 2017, 9, 1135–1143 CrossRef CAS.
- J. Cai, M. M. Rahman, S. Zhang, M. Sarker, X. Zhang, Y. Zhang, X. Yu and E. H. Fini, Review on aging of bio-oil from biomass pyrolysis and strategy to slowing aging, Energy Fuels, 2021, 35, 11665–11692 CrossRef CAS.
- J. Meng, A. Moore, D. Tilotta, S. Kelley and S. Park, Toward understanding of bio-oil aging: Accelerated aging of bio-oil fractions, ACS Sustainable Chem. Eng., 2014, 2, 2011–2018 CrossRef CAS.
- T. I. Korányi, B. Fridrich, A. Pineda and K. Barta, Development of ‘Lignin-First’ approaches for the valorization of lignocellulosic biomass, Molecules, 2020, 25, 2815 CrossRef PubMed.
-
L. Duc, K. Morishita and T. Takar, in Biomass now - Sustainable growth and use, 2013, ch. 11, DOI:10.5772/55356.
- B. Tian, S. Du, F. Guo, Y. Dong, S. Mao, L. Qian and Q. Liu, Synthesis of biomimetic monolithic biochar-based catalysts for catalytic decomposition of biomass pyrolysis tar, Energy, 2021, 222, 120002 CrossRef CAS.
- J. E. Q. Quinsaat, E. Feghali, D. J. van de Pas, R. Vendamme and K. M. Torr, Preparation of mechanically robust bio-based polyurethane foams using depolymerized native lignin, ACS Appl. Polym. Mater., 2021, 3, 5845–5856 CrossRef CAS.
- K. Van Aelst, E. Van Sinay, T. Vangeel, Y. Zhang, T. Renders, S. Van den Bosch, J. Van Aelst and B. F. Sels, Low molecular weight and highly functional RCF lignin products as a full bisphenol a replacer in bio-based epoxy resins, Chem. Commun., 2021, 57, 5642–5645 RSC.
- U. Junghans, J. J. Bernhardt, R. Wollnik, D. Triebert, G. Unkelbach and D. Pufky-Heinrich, Valorization of lignin via oxidative depolymerization with hydrogen peroxide: Towards carboxyl-rich oligomeric lignin fragments, Molecules, 2020, 25, 2717 CrossRef CAS PubMed.
- K. Barta, G. R. Warner, E. S. Beach and P. T. Anastas, Depolymerization of organosolv lignin to aromatic compounds over Cu-doped porous metal oxides, Green Chem., 2014, 16, 191–196 RSC.
- S. A. Kim, S. E. Kim, Y. K. Kim and H.-Y. Jang, Copper-catalyzed oxidative cleavage of the C–C bonds of β-alkoxy alcohols and β-1 compounds, ACS Omega, 2020, 5, 31684–31691 CrossRef CAS PubMed.
- S. Bertella and J. S. Luterbacher, Lignin Functionalization for the Production of Novel Materials, Trends Chem., 2020, 2, 440–453 CrossRef CAS.
- P. Figueiredo, K. Lintinen, J. T. Hirvonen, M. A. Kostiainen and H. A. Santos, Properties and chemical modifications of lignin: Towards lignin-based nanomaterials for biomedical applications, Prog. Mater. Sci., 2018, 93, 233–269 CrossRef CAS.
- W. Li, Z. Chen, H. Yu, J. Li and S. Liu, Wood-derived carbon materials and light-emitting materials, Adv. Mater., 2021, 33, 2000596 CrossRef CAS PubMed.
- J. Li, W. Liu, X. Qiu, X. Zhao, Z. Chen, M. Yan, Z. Fang, Z. Li, Z. Tu and J. Huang, Lignin: a sustainable photothermal block for smart elastomers, Green Chem., 2022, 24, 823–836 RSC.
- K. Wan, B. Tian, Y. Zhai, Y. Liu, H. Wang, S. Liu, S. Li, W. Ye, Z. An, C. Li, J. Li, T. D. James and Z. Chen, Structural materials with afterglow room temperature phosphorescence activated by lignin oxidation, Nat. Commun., 2022, 13, 5508 CrossRef CAS PubMed.
- H. Zhang, T. Chen, Y. Li, Y. Han, Y. Sun and G. Sun, Novel lignin-containing high-performance adhesive for extreme environment, Int. J. Biol. Macromol., 2020, 164, 1832–1839 CrossRef CAS PubMed.
- K. Van Aelst, E. Van Sinay, T. Vangeel, Y. Zhang, T. Renders, S. Van den Bosch, J. Van Aelst and B. F. Sels, Low molecular weight and highly functional RCF lignin products as a full bisphenol a replacer in bio-based epoxy resins, Chem. Commun., 2021, 57, 5642–5645 RSC.
- G. Wang, X. Liu, J. Zhang, W. Sui, J. Jang and C. Si, One-pot lignin depolymerization and activation by solid acid catalytic phenolation for lightweight phenolic foam preparation, Ind. Crops Prod., 2018, 124, 216–225 CrossRef CAS.
- B.-L. Xue, P.-L. Huang, Y.-C. Sun, X.-P. Li and R.-C. Sun, Hydrolytic depolymerization of corncob lignin in the view of a bio-based rigid polyurethane foam synthesis, RSC Adv., 2017, 7, 6123–6130 RSC.
- S. Chen, Y. Xin and C. Zhao, Multispectroscopic analysis in the synthesis of lignin-based biophenolic resins, ACS Sustainable Chem. Eng., 2021, 9, 15653–15660 CrossRef CAS.
- R. M. O'Dea, P. A. Pranda, Y. Luo, A. Amitrano, E. O. Ebikade, E. R. Gottlieb, O. Ajao, M. Benali, D. G. Vlachos, M. Ierapetritou and T. H. Epps, Ambient-pressure lignin valorization to high-performance polymers by intensified reductive catalytic deconstruction, Sci. Adv., 2022, 8, eabj7523 CrossRef PubMed.
- M. B. Figueirêdo, P. J. Deuss, R. H. Venderbosch and H. J. Heeres, Valorization of pyrolysis liquids: Ozonation of the pyrolytic lignin fraction and model components, ACS Sustainable Chem. Eng., 2019, 7, 4755–4765 CrossRef.
- S. Qiu, M. Wang, Y. Fang and T. Tan, Reductive catalytic fractionation of lignocellulose: when should the catalyst meet depolymerized lignin fragments?, Sustainable Energy Fuels, 2020, 4, 5588–5594 RSC.
- H. Yang, Y. Cui, T. Han, L. Sandström, P. Jönsson and W. Yang, High-purity syngas production by cascaded catalytic reforming of biomass pyrolysis vapors, Appl. Energy, 2022, 322, 119501 CrossRef CAS.
- J. Kim, T.
V. T. Nguyen, Y. H. Kim, F. Hollmann and C. B. Park, Lignin as a multifunctional photocatalyst for solar-powered biocatalytic oxyfunctionalization of C–H bonds, Nat. Synth., 2022, 1, 217–226 CrossRef.
- A. Tagami, C. Gioia, M. Lauberts, T. Budnyak, R. Moriana, M. E. Lindström and O. Sevastyanova, Solvent fractionation of softwood and hardwood kraft lignins for more efficient uses: Compositional, structural, thermal, antioxidant and adsorption properties, Ind. Crops Prod., 2019, 129, 123–134 CrossRef CAS.
- S. Ciofi-Baffoni, L. Banci and A. Brandi, Synthesis of oligomeric mimics of lignin, J. Chem. Soc., Perkin Trans. 1, 1998, 3207–3218, 10.1039/A805027I.
- E. Kiyota, P. Mazzafera and A. C. H. F. Sawaya, Analysis of soluble lignin in sugarcane by ultrahigh performance liquid chromatography–tandem mass spectrometry with a do-it-yourself oligomer database, Anal. Chem., 2012, 84, 7015–7020 CrossRef CAS PubMed.
- J.-M. Yuan, H. Li, L.-P. Xiao, T.-P. Wang, W.-F. Ren, Q. Lu and R.-C. Sun, Valorization of lignin into phenolic compounds via fast pyrolysis: Impact of lignin structure, Fuel, 2022, 319, 123758 CrossRef CAS.
|
This journal is © Institute of Process Engineering of CAS 2023 |