DOI:
10.1039/D2IM00056C
(Minireview)
Ind. Chem. Mater., 2023,
1, 93-105
Multicomponent catalyst design for CO2/N2/NOx electroreduction
Received
8th December 2022
, Accepted 28th December 2022
First published on 3rd January 2023
Abstract
Electroreduction of small molecules such as CO2, N2, and NO3− is one of the promising routes to produce sustainable chemicals and fuels and store renewable energy, which could contribute to our carbon neutrality goal. Emerging multicomponent electrocatalysts, integrating the advantages of individual components of catalysts, are of great importance to achieve efficient electroreduction of small molecules via activation of inert bonds and multistep transformation. In this review, some basic issues in the electroreduction of small molecules including CO2, N2, and NO3− are briefly introduced. We then discuss our fundamental understanding of the rule of interaction in multicomponent electrocatalysts, and summarize three models for multicomponent catalysts, including type I, “a non-catalytically active component can activate or protect another catalytic component”; type II, “all catalytic components provide active intermediates for electrochemical conversion”; and type III, “one component provides the substrate for the other through conversion or adsorption”. Additionally, an outlook was considered to highlight the future directions of multicomponent electrocatalysts toward industrial applications.
Keywords: Green chemistry; Green carbon science; Electrocatalysis; Synergetic effect.
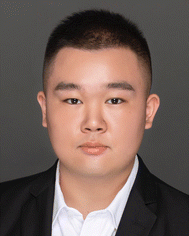 Shunhan Jia | Shunhan Jia received his B.S. degree in Chemistry from Nankai University working with Prof. Zhiqiang Niu on the design and fabrication of flexible aqueous zinc-ion batteries. After his undergraduate research stay in Prof. Enzheng Shi's group at Westlake University focusing on 2D halide perovskite heterostructures, he started to pursue his Ph.D. under the supervision of Prof. Buxing Han and Prof. Xiaofu Sun at the Institute of Chemistry, Chinese Academy of Sciences (CAS). His research interests now center on multicomponent catalysts and the transformation of inert molecules (e.g., CO2 and N2) into value-added chemicals using renewable energy. |
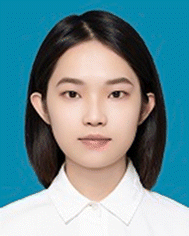 Limin Wu | Limin Wu received her B.E. degree in Materials Science and Engineering from Hebei University of Technology. She started to pursue her Ph.D. under the supervision of Prof. Xiaofu Sun at the Institute of Chemistry, Chinese Academy of Sciences (CAS). Her research is focused on the utilization and conversion of nitrate. |
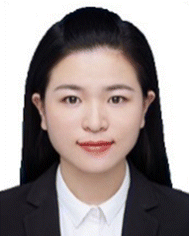 Liang Xu | Liang Xu received her Ph.D. degree in Chemical Engineering and Technology from Beijing University of Chemical Technology in 2019. Currently, she is working as a post-doctoral fellow with Prof. Buxing Han and Prof. Xiaofu Sun at the Institute of Chemistry, Chinese Academy of Sciences (CAS). Her research interests mainly focus on the electrocatalytic conversion of CO2/NOx into high value-added chemicals. |
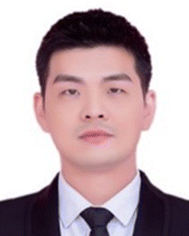 Xiaofu Sun | Xiaofu Sun received his B.S. degree in Chemistry from Nankai University in 2011 and M.S. degree in Physical Chemistry from Renmin University of China in 2014. He earned his Ph.D. degree at the Institute of Chemistry, Chinese Academy of Sciences (CAS) in 2017, and did postdoctoral research at Nanyang Technological University. He has been a professor at the Institute of Chemistry, CAS, since 2019. His current research interests cover utilization and conversion of CO2/NOx, applications of green solvents (e.g., H2O, ionic liquids), and design and synthesis of novel catalysts and catalytic systems. |
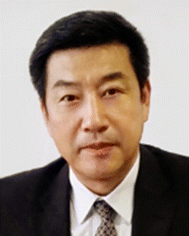 Buxing Han | Buxing Han received his Ph.D. degree from the Institute of Chemistry, Chinese Academy of Sciences (CAS) in 1988, and did postdoctoral research from 1989 to 1991 at the University of Saskatchewan, Canada. He has been a professor at the Institute of Chemistry, CAS, since 1993. He is an Academician of CAS and a Fellow of the Royal Society of Chemistry, a titular member of the Organic and Biomolecular Chemistry Division, IUPAC, and was the Chairman of the IUPAC Subcommittee on Green Chemistry from 2008 to 2012. He works in the interdisciplinary area of Physical Chemistry and Green Chemistry. His research interests include properties of green solvent systems and applications of green solvents in chemical reactions and materials science. |
1 Introduction
The chemical industry plays a crucial role in the historical evolution of society, but it causes emerging environmental concerns and skyrocketing CO2 emissions.1–3 Recently, green chemistry and chemical engineering have opened sustainability possibilities through transforming renewable feedstocks, such as CO2 and NOx (N2 and NO3−), into environmentally friendly chemicals, including syngas, hydrocarbons, oxygenates and ammonia. However, the existence of inert bonds, e.g., the C
O bond in CO2 and the N
N bond in N2, brings both restricted thermodynamics and sluggish kinetics to the activation and conversion of these molecules to synthesize sustainable chemicals.4–6 Among the developed strategies, the electrochemical conversion method provides a universal carbon-neutral route to efficiently upgrade green chemical sources with inert bonds to chemicals and fuels under ambient conditions harnessing clean energy.7 Previous studies proved that CO2 could be electrochemically converted to value-added chemicals and fuels, such as formic acid, CO, methanol, ethene and ethanol.8–10 Recently, electrochemical synthesis of ammonia from N2 and NO3− has been also developed, giving new life to NH3 synthesis.
The active components of catalysts are the essential building blocks of the modern chemical research and chemical industry. In a typical electrocatalytic process, active components could serve as intermediates between carriers and the adsorbed intermediates to promote the redox half-reaction.11 Monocomponent electrocatalysts face the disadvantages of poor stability, limited activity, and restricted reaction processes. Thus, the multicomponent design of electrocatalysts has attracted widespread attention.12 In this review, we first briefly introduced basic issues in the electroreduction of small molecules including CO2, N2, and NO3−, which will be taken as typical examples for further discussion. We then systematically shared our fundamental understanding of three models for multicomponent catalysts as is shown in Scheme 1.
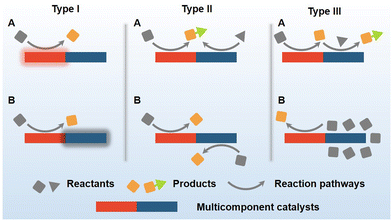 |
| Scheme 1 Models of multicomponent electrocatalysts. | |
Type I: a non-catalytic active component can activate or protect another catalytic component.
Type II: all catalytic components provide active intermediates for electrochemical conversion.
Type III: one component provides the substrate for the other through conversion or adsorption.
Further, centering around the electroreduction of small molecules, we presented recent developments in multicomponent catalysts using the listed models. Future directions were finally discussed for applying multicomponent electrocatalysts in the industrial utilization of renewable chemical sources through highly efficient activation and conversion of inert bonds.
2 Electrochemical reduction of CO2/N2/NOx
2.1 Electroreduction of CO2 to chemicals and fuels
CO2 is the main greenhouse gas but is also demonstrated as a promising C1 chemical source. Due to its controlled pathways, simple reaction unit, green process, and scale-up potential, the electrochemical CO2 reduction reaction (CO2RR) is a promising way to convert CO2 to value-added chemicals and fuels with H2O as the proton source and store increasingly produced clean electricity.13 Researchers have succeeded to obtain CO, methanol (CH3OH), formic acid (HCOOH), methane (CH4), ethanol (CH3CH2OH), ethene (C2H4), and other chemicals from the CO2RR.13–15 The carbon energy index (CEI, Fig. 1A) is newly defined in green carbon science to describe carbon-containing molecules with their atomic ratio (C/C, H/C, and O/C).2 Understandably, molecules with larger NH/NC are favorable to release energy. A semiquantitative overview of common CO2RR products is presented in Fig. 1B with data from ref. 16 and 17. The techno-economic analysis of the CO2RR over various catalysts, electrolytes, and cells with accurate data has been well discussed in several literature reports, which will not be discussed in detail in the current minireview.16–20 Briefly, obvious disadvantages exist in HCOOH compared to other products. Despite the high CEI of CH4, high electricity cost hinders the further application of CO2-to-CH4. Conversely, CO, CH3OH, and C2 chemicals from the CO2RR are economically feasible, technologically accessible, and of great potential in the carbon-neutral future.
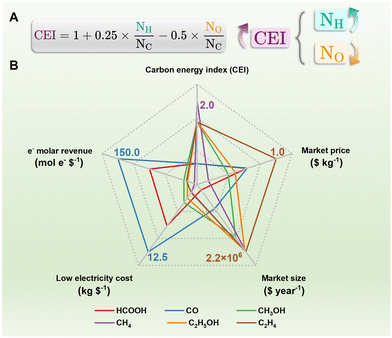 |
| Fig. 1 (A) The formula to calculate the carbon energy index (CEI) of different carbon-containing molecules. NX (X = C, H, and O): the number of X atoms in the molecule. (B) An overview of common CO2RR products from the perspective of both chemistry and economics. | |
As is shown in Fig. 2A, the electroreduction of CO2 with H2O to various target molecules is a complex proton-coupled electron transfer process.15 Generally, the CO2 molecule could first transform into CO2˙− and *CO after being adsorbed into the electrode guided by different catalysts. The CO2˙− and *CO intermediates could directly desorb from the electrode for the formation of C1 products such as HCOOH and CO. However, to produce more useful products discussed in Fig. 2A, multi-step electroreduction with active H species, C–C coupling, and multi-step transformation are necessary, in which multicomponent catalysts summarized here are thus needed.
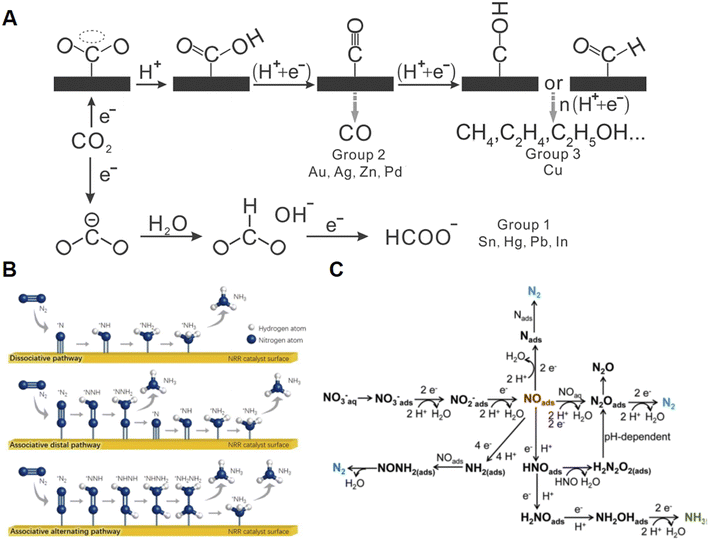 |
| Fig. 2 (A) The possible pathways of multi-step activation and transformation of CO2 to various value-added products. Reprinted with permission from ref. 15. Copyright 2016, John Wiley & Sons, Inc.; Proposed roadmap of multi-step electroreduction of (B) N2 and (C) NO3− towards NH3 synthesis. Reprinted with permission from ref. 22 and 23. Copyright 2019, Elsevier and Copyright 2021, the Royal Society of Chemistry. | |
2.2 Electroreduction of N2 and NO3− to NH3
The industrial Haber–Bosch process is of great importance to modern society since it could produce useful NH3 from N2 and H2. Nonetheless, highly energy-intensive, huge CO2 emissions from the generation of H2 and centralized facilities are the main drawbacks of the current Haber–Bosch process making it unsustainable. Electrochemical reduction of N2 and its derivatives such as NO3− with H2O as the proton source provides a sustainable and carbon-neutral way to synthesize NH3.6 Nonetheless, activation and conversion of the inert bonds in N2 and NO3− are inherently challenging because of their thermodynamic stability.21 The electrochemical N2 reduction reaction (NRR) in Fig. 2B is usually based on dissociative and associative mechanisms.22 In the dissociative mechanism, N2 molecules adsorbed on the electrode surface could undergo the activation of the N–N bond first with high energy input. Meanwhile in the associative mechanism, two nitrogen atoms could link with each other until the formation of one NH3 molecule. In addition, these two pathways could occur alternatingly through the in-turn hydrogenation of two nitrogen atoms to form two NH3 molecules. As for the nitrate reduction reaction (NITRR) shown in Fig. 2C, the conversion of NO3− to *NO2− is the first step, followed by the reduction of *NO2− to *NO, which could undergo multistep transformation to NH3 with *NH2OH as the key intermediate.23,24 Hence, both the NRR and NITRR are also complex and require multicomponent electrocatalysts to drive the reactions with enhanced efficiency. In addition, electroreduction of other NOx molecules, such as NO (x = 1) and NO2− (x = 2), to NH3 has been also reported but will not be discussed in the current review.25–28
3 Emerging multicomponent catalysts
3.1 Type I multicomponent catalysts
3.1.1 Models of multicomponent catalysts.
Multicomponent catalysts, as the name implies, have at least two components, but it is worth noting that not all the components in a multicomponent catalyst act as catalytic sites for catalytic reactions. The main characteristic of a type I (Scheme 1) multicomponent catalyst is that only one component acts as the active center during the catalytic reaction. The other component could enhance the activity and stability of the catalytic reaction by activating the main component or preventing its degradation. For instance, as is shown in type I-A, in a multicomponent catalyst, A/B, A is the true active site, while B has only low or virtually no catalytic activity. In the catalytic reaction, A could be activated by B to A+, so it has high activity. For instance, researchers discovered that B could tune the oxidation state of the A site, which may be favorable to electrocatalysts.8 In some cases, like type I-B, A is not a stable catalytic site in the reaction, such as Aδ+ in electroreduction reactions. A can downgrade to A− and thus become inactive. Here, by converting B to B−, B could be used as a sacrificing component. Typically, through electro-transfer between A and B, A could be more stable, and the catalytic reaction can continue at an ideal rate, which is important for the activation and transformation of inert bonds. In the following section, recent advances in multicomponent electrocatalysts with one active site and a secondary component that could enhance and stabilize the main component in the electroreduction of CO2, N2, and NO3− will be taken as examples to present the application of this model (Fig. 3 and 4). Type I multicomponent catalysts could be formed through doping, direct synthesis, and in situ phase separation. Compared with traditional immobilized catalysts, type I multicomponent catalysts could not only provide a substrate to active sites, but could also enhance the activity of catalytic sites through the interaction. Type I multicomponent catalysts in electroreduction of small molecules could promote the reactions through enhancing substrate adsorption, stabilizing key intermediates, and inhibiting side reactions (such as the hydrogen evolution reaction, HER).
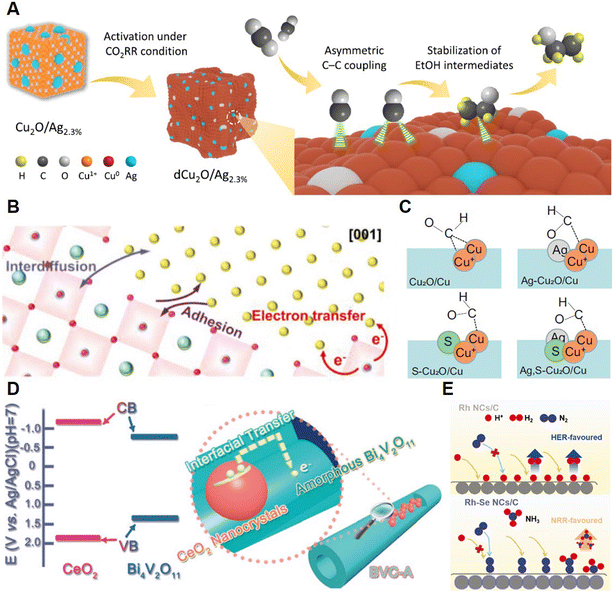 |
| Fig. 3 (A) Illustration of promoted CO2-to-C2H5OH on a dCu2O/Ag2.3% electrocatalyst. Reprinted with permission from ref. 29. Copyright 2022, Springer Nature; (B) Interaction between perovskite oxides and Cu nanoparticles. Reprinted with permission from ref. 30. Copyright 2022, John Wiley & Sons, Inc.; (C) *CHO intermediates on different Cu-based electrocatalysts. Reprinted with permission from ref. 31. Copyright 2022, Springer Nature; (D) Schematic for interfacial charge transfer in BVC-A. Reprinted with permission from ref. 33. Copyright 2018, John Wiley & Sons, Inc.; (E) Different NRR pathways on Rh-based catalysts before and after adding the Se component. Reprinted with permission from ref. 34. Copyright 2020, John Wiley & Sons, Inc. | |
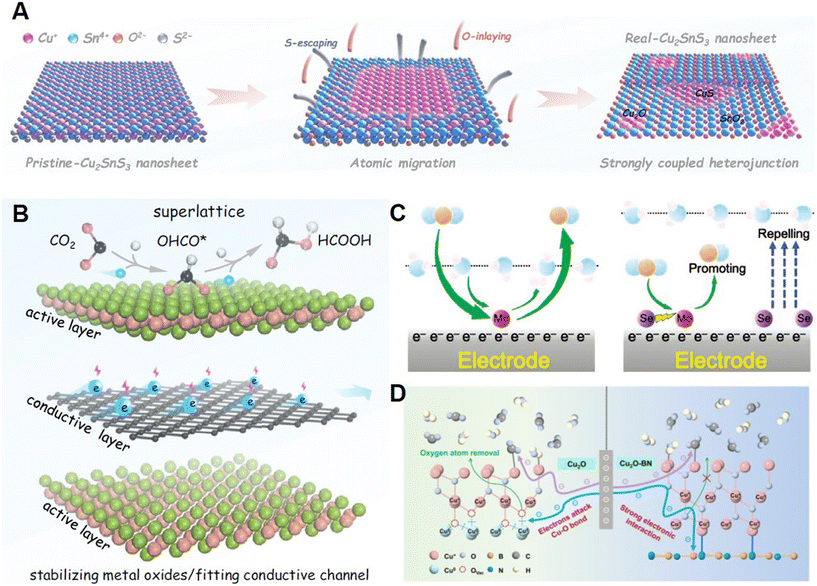 |
| Fig. 4 (A) Schematic for the in situ phase separation of Cu2SnS3 to SnO2@CuS and SnO2@Cu2O heterojunctions with enhanced performance. Reprinted with permission from ref. 35. Copyright 2021, John Wiley & Sons, Inc.; (B) Illustration of a multicomponent superlattice constructed from a metal oxide layer and a conductive layer. Reprinted with permission from ref. 36. Copyright 2022, Springer Nature; (C) Reaction pathway of the CO2RR on the Mo–Se multicomponent electrocatalyst. Reprinted with permission from ref. 37. Copyright 2022, John Wiley & Sons, Inc.; (D) Cu active sites protected by strong electronic interaction during the CO2RR. Reprinted with permission from ref. 38. Copyright 2022, John Wiley & Sons, Inc. | |
3.1.2 Applications in electrochemical reactions.
3.1.2.1 Type I-A.
As is shown in Fig. 3A, Qiao and co-workers recently reported the electroreduction of CO2 to C2H5OH on a multicomponent electrocatalyst containing Ag and CuO.29 The Ag-modified CuO could achieve the production of C2H5OH at 326.4 mA cm−2 at −0.87 V vs. RHE with a faradaic efficiency (FE) of 40.8%. The outstanding performance was attributed to the CuO activated by Ag, in which the CuAg alloy could be formed under a reductive potential. The strength of *CO (including *CObridge and *COatop) was tuned by the Ag component and thus provided an asymmetric and highly efficient C–C coupling pathway to C2H2OH production. Zhu et al. explored the utilization of La0.4Sr0.4Ti0.9O3−δ perovskite oxide socketed with sub-3 nm Cu nanoparticles (LSTr-Cu) for reduction of CO2 to C2+ chemicals.30 Strong interaction between the perovskite and the Cu component was found to be critical to the enhancement of electrochemical performance (Fig. 3B). During the epitaxial growth, Cu nanoparticles, the active site of the CO2RR, could diffuse into the lattice of LSTr and thus be tuned by the LSTr component by electrotransfer with Ti4+/Ti3+ and the moving d-band center. Compared with the physical mixture of Cu and perovskite, LSTr-Cu had enhanced performance for C2+ production up to 6.2 fold. Our group recently presented an in situ dual doping strategy to achieve efficient CO2-to-CH3OH electrocatalysis with Ag,S–Cu2O/Cu, which could achieve a FE of methanol of 67.4% with a current density of 122.7 mA cm−2 in an H-type cell using an ionic liquid-based electrolyte.31 The S anion could tune the electronic structure and morphology of the Cu active sites to favor the *CHO intermediate adsorbed and the CH3OH pathway. Besides, the Ag cation could suppress the HER. Their interactions with the Cu active sites could thus enhance the current density and selectivity of the CO2RR (Fig. 3C). Wang and co-workers recently reported that Ru sites dispersed on Cu nanowires could catalyze the NITRR at the industrial-relevant current density of 1 A cm−2 with a high FE of 93%.32 The Ru sites were proved to be the catalytic sites of the NITRR while the Cu component could boost the reactivity of the Ru sites through suppressing the HER.
The Yu group reported a noble-metal-free multicomponent electrocatalyst, amorphous phase Bi4V2O11/CeO2 (BVC-A), for a highly efficient NRR.33 Significant defects on Bi4V2O4 could play a role as active sites, while CeO2 could serve as the trigger for the formation of the amorphous phase. More importantly, as is shown in Fig. 3D, interfacial electron transfer from CeO2 to Bi4V2O4 enabled by type I band alignment established in the BVC-A catalyst could boost its NRR performance remarkably since the average yield of NH3 could reach 23.21 mg h−1 mgcat.−1 with a FE of 10.16%, which is 3 times better than that of the Bi4V2O11/CeO2 hybrid with a crystalline phase. Huang et al. recently utilized a surface chalcogenation strategy to build multicomponent catalysts with Rh–Se nanocrystals (Rh–Se NCs) which thus promoted the NRR performance.34 Surface chalcogenation could boost the performance of Rh–Se NCs by reducing the apparent activation energy of reactant molecules and inhibiting the HER (Fig. 3E). A rather low valence state was constructed for the critical redox inversion induced by the multicomponent core–shell structure of Rh–Se NCs, which could boost the FE by 15 times to 13.3% with an NH3 yield of 175.6 mg h−1 gRh−1.
3.1.2.2 Type I-B.
Zhai and coworkers reported the construction of SnO2@CuS and SnO2@Cu2O heterojunctions through in situ structure evolution of CuSnS3 nanosheets as an efficient electrocatalyst for CO2-to-HCOOH, with Sn4+ as the active site, as is presented in Fig. 4A. Sn4+ was well protected by the electro-self-flow to Cu+ during electrolysis.35 The delocalization of Sn-based active sites enabled by charge transfer could boost the coupling between H* and HCOO*, thereby accelerating the kinetics of the CO2RR and exhibiting excellent electrochemical performance of 83.4% HCOOH formation in a wide potential range (−0.6 V to −1.1 V). The Zhai group also reported a tangible superlattice (Fig. 4B) consisting of two sublayers including [Bi2O2]2+ and [Cu2Se2]2− for the protection of Bi active sites in the [Bi2O2]2+ layer with the conductive [Cu2Se2]2− sublayer.36 Compared to the self-reduced Bi metal, the Bi active sites in the [Bi2O2]2+ layer could accept electrons rapidly transferring from the [Cu2Se2]2− layer and be protected in the CO2RR. Moreover, in the [Bi2O2]2+ layer, Bi p orbitals and O p orbitals were proved to have unique overlaps by theoretical calculations that could enhance the adsorption of the OCHO* intermediate. Hence, in a wide potential range from −0.4 to −1.1 V, BiCuSeO superlattices could exhibit ideal FE to HCOOH of >90%. Chen and co-workers recently demonstrated a Mo–Se dual single-atom electrocatalyst (MoSA–SeSA) for the CO2RR producing CO at a wide potential of −0.4 to −1.0 V with a FE of >90%.37 As is presented in Fig. 4C, MoSA was proved to be the active site here since it could interact with CO2 and its intermediates during the CO2RR, while SeSA could promote the performance of MoSA. Operando and theoretical studies revealed that SeSA could tune the electronic structure via long-range electro-delocalization, which is essential to avoid the inhibition of MoSA caused by the strong CO adsorption. Additionally, SeSA could also suppress the HER through repelling H2O molecules and accelerate the CO2 transport for MoSA to boost the CO2RR. Yan and co-workers utilized hexagonal boron nitride (h-BN) nanosheets to decorate Cu2O nanoparticles with Cu+ active sites with enhanced stability.38 Through using the h-BN substrate, the C2H4/CO ratio in the CO2RR could be improved 1.62 times in contrast to traditional Cu2O catalysts. As presented in Fig. 4D, interaction in the multicomponent catalysts is critical to performance improvement. The h-BN substrate could strengthen the Cu–O bond and receive partial electron density from Cu2O. As a result, the Cu+ species could be stabilized through its interaction with h-BN during long-term CO2RR. Zhu and colleagues developed a novel framework by assembling [Zr48Ni6] nano-cages, which could extract AuCl4− from electronic waste, due to its high surface area and high stability in solvents.39 More importantly, this kind of framework adsorbing AuCl4− could be converted to efficient multicomponent catalysts loading low-cost Au nanoparticles with controlled sizes. The catalysts could exhibit excellent FE of CO as high as 95.2% at a current density of 102.9 mA cm−2, which could be attributed to the confinement effect provided by the nanoclusters of [Zr48Ni6] protecting Au nanoparticles from deactivation. Recently, our group discovered an in situ periodic regeneration method to develop long-term stable electrocatalysts for the CO2RR.40 A positive potential pulse with a short period was utilized to generate Cu+ active sites through the interaction with an electrolyte containing halide ions. The halide anion participated in the formation and stabilization of the highly active catalysts. This method could enhance the performance of the CO2RR on commercial Cu-based catalysts with a FE of 81.2% and a current density of 22.7 mA cm−2 with a stability of more than 36 h.
3.2 Type II multicomponent catalysts
3.2.1 Models of multicomponent catalysts.
In type II multicomponent catalysts, two components could participate in the catalytic reaction at the same time providing active intermediates for the conversion of substrate molecules. Specifically, type II-A could supply different intermediates for product formation. For example, although the HER is the main side reaction of most small molecule electroreduction reactions and needs to be suppressed, active proton species play an important role in the electroreduction of small molecules since water could serve as the green proton source in the aqueous system. However, it is difficult for traditional monocomponent catalysts to generate several types of species at the same time. Multicomponent catalysts are thus demanded to generate various intermediates in the reactions. In the CO2RR, reactive hydrogen species could enhance the conversion of CO2 to value-added products with multiple electron transfer processes such as alcohols. In addition, reactive hydrogen species are also very important for the electrochemical hydrogenation of inert bonds in N2. Type II-B catalysts could simultaneously produce the same product, thus improving the performance of the reaction. In multicomponent catalysts, the synergistic effect of each component has been widely studied and proved to promote the reaction, making the performance of the multicomponent catalysts better than the effect of the two components acting separately. Additionally, the interaction between the loaded component and the substrate is also very important in this type of multicomponent catalyst. In the traditional impregnated catalysts, the substrate could not provide active sites, while recent studies showed that the activity of the catalyst substrates could boost the electroreduction efficiently. Hence, type II multicomponent catalysts could drive the electroreduction of small molecules with enhanced efficiency and produce more valuable products, which would be introduced in detail in the next subsection.
3.2.2 Applications in electrochemical reactions.
3.2.2.1 Type II-A.
Our group reported the efficient conversion of CO2 to methanol on Mo–Bi bimetallic chalcogenide (MBC) in ionic liquid-based electrolytes through synergistic effects confirmed by control experiments. CO and H2 intermediates were generated at Bi and Mo sites, respectively, and these intermediates were proved to be important in the production of methanol. The FE of methanol on MBC catalysts could reach 71.2% with a current density of 12.1 mA cm−2.41 Guo and co-workers recently reported phthalocyanines polymerized through the solid ion thermal utilizing Scholl reaction (Fig. 5A) with carbon nanotubes as the substrates, which could provide both CoN4 sites and H2Pc sites for the CO2RR.42 As is shown in Fig. 5B, in the phthalocyanine catalysts, CoN4 could serve as the active site to interact with CO2 molecules while H2Pc sites could boost the CO2RR as the donors of protons and electrons. As a result, the performance of phthalocyanines could reach a FE of 97% with a large current density of >200 mA cm−2. Sargent et al. explored the doping of hydroxide and oxide to Cu catalysts that could tune the adsorption energy of hydrogen species on the Cu surface through the synthesis of Ce(OH)x-doped-Cu catalysts.43 As is presented in Fig. 5C, hydroxide and oxide dopants could enhance the hydrogenation of *HCCOH to produce ethanol products by providing proton intermediates. Competently, Ce(OH)x-doped-Cu catalysts could reach an ethanol partial current density of 128 mA cm−2 with a FE of 43% and a high ethanol/ethylene ratio. The Cheng group reported a nanoporous NiSb alloy as an efficient multicomponent catalyst for the NRR.44 As is presented in Fig. 5D, the Ni sites could enhance the hydrogenation of N2 and the Sb sites could separate the active sites for N2 adsorption and active proton species generation, which is helpful to the activation of inert N2 molecules. Due to the separated active sites, the NRR performance on the NiSb alloy could reach a high NH3 yield rate of 56.9 μg h−1 mg−1 with a FE of 48.0%.
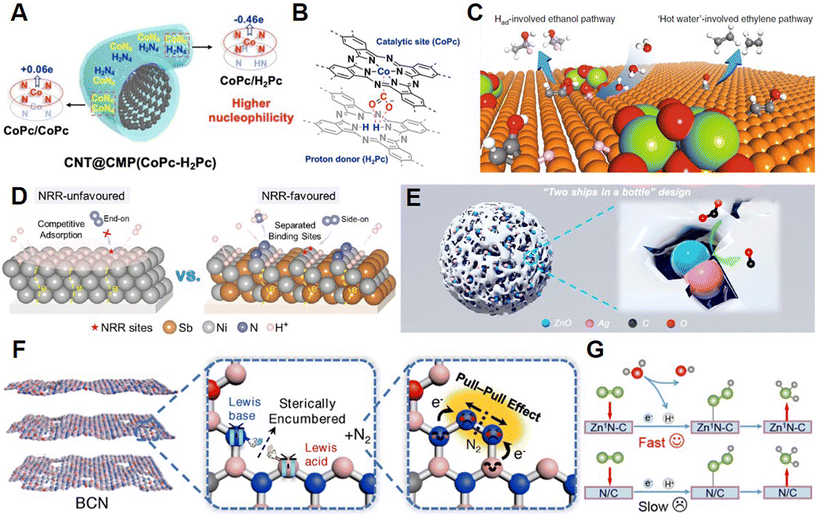 |
| Fig. 5 (A) Structure and (B) catalytic mechanism of the CNT@CMP(CoPc–H2Pc) electrocatalyst. Reprinted with permission from ref. 42. Copyright 2022, John Wiley & Sons, Inc.; (C) Proton-involved CO2-to-CH2H5OH pathway on the Ce(OH)x-doped-Cu electrocatalyst. Reprinted with permission from ref. 43. Copyright 2019, Springer Nature; (D) Comparison of the NRR on different Ni-based catalysts. Reprinted with permission from ref. 44. Copyright 2021, John Wiley & Sons, Inc.; (E) “Two ships in a bottle” design strategy of Zn–Ag–O catalysts for the CO2RR. Reprinted with permission from ref. 46. Copyright 2021, the American Chemical Society; (F) Advantages of BCN with frustrated Lewis pairs in the NRR. Reprinted with permission from ref. 48. Copyright 2022, John Wiley & Sons, Inc.; (G) Comparison of NRR pathways on Zn1N–C and N/C. Reprinted with permission from ref. 49. Copyright 2022, John Wiley & Sons, Inc. | |
3.2.2.2 Type II-B.
Recently, defective CuO-supported atomic Sn sites were synthesized as superior electrocatalysts for CO2-to-CH3OH transformation aided by the cooperation of multiple sites including Sn, oxygen vacancies, and the CuO support, which could generate CH3OH at the same time.45 As a result, the multicomponent catalyst could exhibit a methanol FE of 88.6% with a current density of 67.0 mA cm−2. In Fig. 5E, Chen and colleagues proposed a “two ships in a bottle” design and synthesized a multicomponent Zn–Ag–O catalyst for the CO2RR with a high energy efficiency of 60.9%, a CO FE of 94.1%, and ideal stability.46 The excellent performance of Zn–Ag–O could be attributed to the enhanced adsorption of *COOH intermediates on the surface of Zn and Ag domains. Additionally, the surfaces could also hinder the detachment and agglomeration of catalysts during the CO2RR. The unique porous structure in metal–organic frameworks could provide a useful platform for multicomponent catalysts.47 Zhu and co-workers reported a porous 3D In-MOF, {(Me2NH2)[In(BCP)]·2DMF}n (V11), with two types of channels that could transfer CO2 to HCOOH with a FE of 76%.44 Researchers discovered that carbon particles produced from methylene blue molecules could be introduced to the channels of V11 through pyrolysis. Multicomponent catalysts containing V11 and carbon particles could improve both the FE (from 76.0% to 90.1%) and current density (2.2 times) of HCOOH generation, because of the enhanced charge and mass transfer and sufficiently exposed active sites. The Dai group presented a defective boron carbon nitride (BCN) catalyst with both Lewis acid and base sites for the NRR, which has long-term stability and could exhibit a high FE of 18.9%, and the yield of NH3 could reach 20.9 μg h−1 mgcat−1.48 The frustrated Lewis pairs (FLPs) in BCN catalysts could provide the possibility for the formation of a six-membered ring intermediate during the NRR, as is shown in Fig. 5F, enabling the efficient N2 cleavage through pull–pull efficiency. Hou and collaborators reported hollow porous N-doped carbon nanofibers anchoring single Zn(I) sites (Zn1N–C) for the NRR.49 As is presented in Fig. 5G, the isolated Zn sites and nearby graphitic N sites could synergistically activate and convert the inert N2 molecules by decreasing the energy barrier of the *NNH intermediate formation, which was proved to be the rate-limiting step in the NRR. Therefore, the yield of NH3 production on Zn1N–C could reach 16.1 μg h−1 mgcat.−1 with a FE of 11.8%. The Zhang group demonstrated a highly efficient NITRR on a multicomponent Cu/Cu2O catalyst in situ derived from CuO with a FE as high as 95.8% and an excellent selectivity of 82%.50 Electron transfer from Cu2O to Cu led to *NOH intermediate adsorption at the interface, which was attributed to the promoted NH3 production and suppressed HER.
3.3 Type III multicomponent catalysts
3.3.1 Models of multicomponent catalysts.
The type III multicomponent catalysts emphasize the sequential action of different catalytic sites in the electroreduction of small molecules. For example, type III-A multicomponent catalysts could convert the substrate molecules in tandem with the migration of intermediates. Each active site could catalyze the individual step in the reaction, which could be coupled into the whole reaction. This effect is important because of the ability to boost key processes in the electroreduction of small molecules such as C2+ product generation from in situ generated CO intermediates and efficient hydrogenation of NO2− intermediates in the NITRR to produce NH3 with an enhanced yield rate. The catalytic sites with different components could provide active sites for the above-mentioned steps. Materials including alloys, bimetallic heterostructures, and framework materials could serve as efficient catalysts for this subtype of catalyst. In type III-B, active sites could also boost the reaction with the help of components that could enhance the adsorption of substrate molecules such as CO2, N2, and NO3−. These substrates usually suffer from low solubility and (or) concentration in the electrolyte, which could hinder their electroreduction with the limitation of mass transfer. Thus, the component in the catalysts that could provide high local concentration through adsorption could assist in the promotion of electrochemical performance of real active sites fed with more substrates. Porous materials, which could provide ideal gas adsorption due to their high specific surface area, were proved to be effective providers of adsorption sites in type III-B catalysts. In addition, catalysts with unique electric fields and nanostructures were also reported.
3.3.2 Applications in electrochemical reactions.
3.3.2.1 Type III-A.
Fan and collaborators demonstrated the delicate synthesis of Ag–Cu Janus nanocatalysts (Ag65–Cu35 JNS-100) that could reduce CO2 to C2+ products.51 As is shown in Fig. 6A, CO2 could transfer to CO on the Ag sites, followed by the CO spillover and dimerization on the Cu component. Compared with Cu nanocubes, Ag65–Cu35 JNS-100 could exhibit enhanced FE of C2+ products. The Yu group demonstrated a tandem catalyst for CO2-to-CO through anchoring single atomic Cu sites and Cu nanoclusters on the carbon support doped with N and S.52 The catalyst (Cu–S1N3) could exhibit a CO FE of 100% under optimized conditions, which was attributed to the generation of *CO2− intermediates on CuN4 sites and the hydrogenation of *CO2− on Cu clusters (Fig. 6B). Bao and coworkers reported the CO2RR producing CH4 using copper-free catalysts consisting of phthalocyanine (CoPc) and zinc–nitrogen–carbon (Zn–N–C) sites, which had much better performance than catalysts with one type of site alone.53 CO2 was proved to be reduced to CO on the CoPc sites, while in situ generated CO could be fed to Zn–N–C sites as substrates to produce CH4. Recently, Wu and coworkers reported that segmented gas-diffusion electrodes (s-GDEs) with multiple components could enhance the conversion of CO2 to C2+ chemicals through the prolonged residence time of CO intermediates enabled by the segment at the inlet.54 The Ag component could transfer CO2 to CO, while Cu sites could utilize the in situ generated CO intermediates diffused from the surface of Ag to produce the C2+ products through the formation of C–C coupling. Based on this strategy that could increase the yield of both CO intermediates and C2+ fine products at the same time, researchers developed a Cu/Fe–N–C s-GDE, which could exhibit a high partial current density of C2+ production of ∼1 A cm−2 with a FE of 90%. The Schuhmann lab demonstrated a tandem catalyst for NH3 synthesis from NO3− with a core–shell catalyst with Cu/CuOx and Co/CoO phases.55 As is presented in Fig. 6C, NO3− could first be reduced on the Cu-based sites to NO2−, while NH3 could be generated from NO2− on the surface of Co sites. The high local concentration of NO2− provided by Cu sites could boost the yield of NH3 production obviously. Consequently, a high yield of NH3 of 1.17 mmol cm−2 h−1 could be reached on the multicomponent catalysts with a tandem reduction.
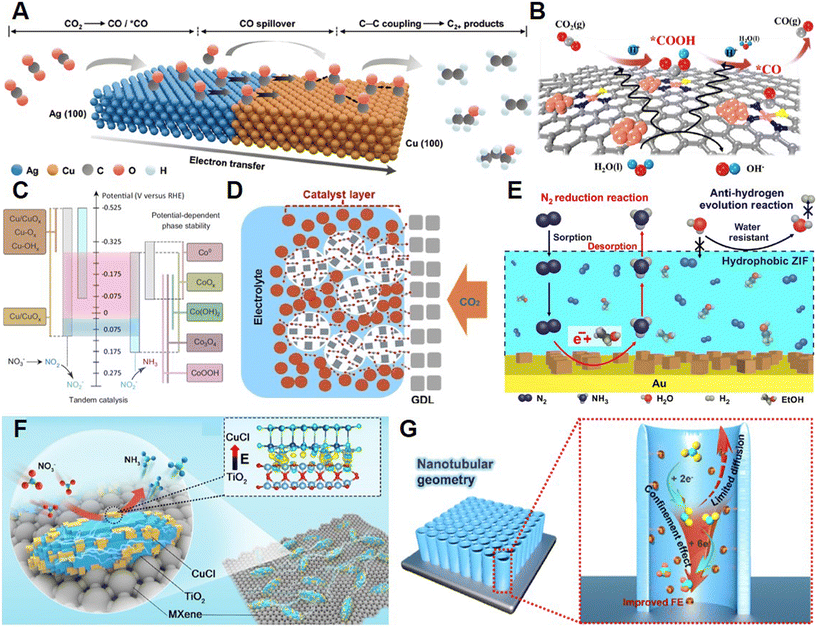 |
| Fig. 6 (A) Proposed reaction mechanism for production of C2 molecules on the Ag65–Cu35 JNS-100 catalyst. Reprinted with permission from ref. 51. Copyright 2022, John Wiley & Sons, Inc.; (B) Tandem CO2-to-CO transformation pathway through Cu-S1N3/Cux. Reprinted with permission from ref. 52. Copyright 2021, John Wiley & Sons, Inc.; (C) Intermediates and their conversion over the CuCoSP tandem catalyst during the NO3RR. Reprinted with permission from ref. 55. Copyright 2022, Springer Nature; (D) CO2 diffusion in GDE enhanced by CC3. Reprinted with permission from ref. 56. Copyright 2022, John Wiley & Sons, Inc.; (E) N2 diffusion on the surface of catalysts encapsulated with ZIF. Reprinted with permission from ref. 57. Copyright 2018, American Association for the Advancement of Science; (F) Built-in electric field in CuCl_BEF catalysts enabling enhanced NITRR. Reprinted with permission from ref. 58. Copyright 2021, John Wiley & Sons, Inc.; (G) Illustration of the nanoreactor for NITR with enhanced FE. Reprinted with permission from ref. 59. Copyright 2022, Elsevier. | |
3.3.2.2 Type III-B.
Recently, our group reported porous organic cages (POCs) as an additive for the CO2RR on Cu catalysts since the limited solubility of CO2 in aqueous solution could hinder the mass transfer and thus reduce the performance.56 CO2 could be adsorbed in the pores of POCs, followed by its diffusion to the surface of Cu catalytic sites. Compared with the CO2 diffusion from an aqueous electrolyte, it was more favorable to diffuse from the hydrophobic pores of the POCs. The enhanced attachment between CO2 and the Cu surface enabled the enhanced CO2RR performance and decreased the HER. As a result, the FE of C2+ chemicals on Cu modified with CC3 (one of the POCs) could reach 76% with a high current density of 1.7 A cm−2. Ling and coworkers designed a reticular chemistry approach for selective NRR, in which zeolitic imidazolate framework-71 (ZIF) was introduced to the surface of NRR electrocatalysts (Fig. 6D).57 The multicomponent catalysts could enhance the interactions between N2 molecules and active sites, while the HER could be suppressed. The catalysts coated with ZIF could exhibit a selectivity to NH3 of ∼90% and an enhanced FE of 11%. The Lu group synthesized a multicomponent catalyst via stacking CuCl and TiO2 on an MXene substrate (CuCl_BEF), which had a built-in electric field because of the electron transfer from TiO2 to CuCl.58 The unique built-in electric field could assist the accumulation of NO3− around TiO2 sites with positive charge and produce a high local NO3− concentration to the Cu catalytic sites (Fig. 6E). Therefore, the electrochemical production of NH3 on CuCl_BEF could reach a selectivity of 98.6% and a high yield of 64.4 h−1 in low NO3− concentration electrolyte (100 mg L−1). Recently, Li and colleagues demonstrated a multicomponent catalyst consisting of CuOx active sites and a TiO2 nanotube reactor (TiO2 NTs/CuOx) for the NITRR, in which TiO2 could hinder the diffusion of NO2− by-products and thus boost the reduction of NO2− on CuOx sites and produce NH3 with higher selectivity.59 The maximum FE of the NITRR on TiO2 NTs/CuOx could reach 92.23% and a high NH3 yield rate of 1241.81 μg h−1 cm−2 could be achieved.
4 Summary and outlook
As discussed above, the electrochemical reduction of small molecules on multicomponent catalysts could contribute to the carbon neutrality and sustainability goals of our society through producing value-added chemicals with renewable sources, and is substantially becoming a crucial research area. However, large-scale and industrial applications of these emerging electrochemical reactions such as the CO2RR, NRR, and NITRR have not been realized to date, which is caused by their low energy efficiency, high cost, and unsatisfactory economic benefits. Additionally, some essential scientific and technological issues need to be addressed in the future, some of which are highlighted as follows:
First, there is no doubt that synthesizing multicomponent electrocatalysts with high reaction activity, ideal selectivity, long-term stability, and low cost is a long-range objective for researchers to advance the electroreduction of small molecules to industry. For instance, the reduction of CO2 to long-chain organic chemicals is also important, which is proposed to be promoted by multicomponent catalysts. Additionally, reduction of CO2/N2 with low partial pressure or undesired impurities and NOx− at low concentration would be enabled by multicomponent catalysts. On the other hand, more research should be conducted to study the structure–property relationship and the interaction of different components in these catalysts.
Second, electrolytes are also an important unit in the electrochemical reduction of small molecules besides catalysts, which could be designed together toward an efficient electrolyte–catalyst system.60 The rules in the coupling of electrolytes and catalysts for electrochemical transformation are needed to be explored.
Third, compared with the direct electroreduction of small molecules with water, few research studies have been conducted on the co-reduction of these molecules and their electrochemical coupling with other organic compounds. Recently, electrochemical synthesis of carboxylic acid, carbonate, urea, amine, and other organic chemicals from the electroreduction of small molecules was reported, but these reactions are still in the starting stage.61–67 Multicomponent catalysts have the advantages of producing C-/N-containing intermediates simultaneously and promoting the C–N coupling.68 Hence, more attention should be paid to developing new routes for electrochemical synthesis using multicomponent catalysts.
Fourth, the electroreduction of small molecules is very complex and our current knowledge is very limited to understand the reaction mechanism of these reactions in detail.69,70 As a result, in situ characterization and theoretical studies should be combined to provide more understanding of the mechanism.
Fifth, we believe that, in the near future, industrial electroreduction of small molecules should be realized, and some problems should be solved including energy harvesting devices, efficient adsorption of substrates such as CO2, advanced flow electrolyzers, coupled reactions, and product separation.
Conflicts of interest
The authors declare no conflict of interest.
Acknowledgements
This work was supported by the National Natural Science Foundation of China (22002172 and 22121002), the Beijing Natural Science Foundation (J210020) and the Photon Science Center for Carbon Neutrality.
References
- M. He, Y. Sun and B. Han, Green carbon science: Scientific basis for integrating carbon resource processing, utilization, and recycling, Angew. Chem., Int. Ed., 2013, 52, 9620–9633 CrossRef CAS PubMed.
- M. He, Y. Sun and B. Han, Green carbon science: Efficient carbon resource processing, utilization, and recycling towards carbon neutrality, Angew. Chem., Int. Ed., 2022, 61, e202112835 CAS.
- P. Gao, L. Zhong, B. Han, M. He and Y. Sun, Green carbon science: Keeping the pace in practice, Angew. Chem., Int. Ed., 2022, 61, e202210095 CAS.
- S. Jia, X. Ma, X. Sun and B. Han, Electrochemical transformation of CO2 to value-added chemicals and fuels, CCS Chem., 2022, 4, 3213–3229 CrossRef CAS.
- X. Song, S. Jia, L. Xu, J. Feng, L. He, X. Sun and B. Han, Towards sustainable CO2 electrochemical transformation via coupling design strategy, Mater. Today Sustain., 2022, 19, 100179 CrossRef.
- L. Wu, W. Guo, X. Sun and B. Han, Rational design of nanocatalysts for ambient ammonia electrosynthesis, Pure Appl. Chem., 2021, 93, 777–797 CrossRef CAS.
- Y. Kawamata and P. S. Baran, Electrosynthesis: Sustainability is not enough, Joule, 2020, 4, 701–704 CrossRef.
- X. Tan, X. Sun and B. Han, Ionic liquid-based electrolytes for CO2 electroreduction and CO2 electroorganic transformation, Natl. Sci. Rev., 2021, 9, nwab022 CrossRef PubMed.
- X. Song, W. Guo, X. Ma, L. Xu, X. Tan, L. Wu, S. Jia, T. Wu, J. Ma, F. Zhang, J. Jia, X. Sun and B. Han, Boosting CO2 electroreduction over Co nanoparticles supported on N,B-co-doped graphitic carbon, Green Chem., 2022, 24, 1488–1493 RSC.
- X. Tan, W. Guo, S. Liu, S. Jia, L. Xu, J. Feng, X. Yan, C. Chen, Q. Zhu, X. Sun and B. Han, A Sn-stabilized Cuδ+ electrocatalyst toward highly selective CO2-to-CO in a wide potential range, Chem. Sci., 2022, 13, 11918 RSC.
- Y. Xu, H. Yang, X. Chang and B. Xu, Introduction to electrocatalytic kinetics, Acta Phys.-Chim. Sin., 2022, 39, 2210025 Search PubMed.
- J. Shi, On the synergetic catalytic effect in heterogeneous nanocomposite catalysts, Chem. Rev., 2013, 113, 2139–2181 CrossRef CAS PubMed.
- L. Zhou and R. Lv, Rational catalyst design and interface engineering for electrochemical CO2 reduction to high-valued alcohols, J. Energy Chem., 2022, 70, 310–331 CrossRef CAS.
- Y. Zhai, P. Han, Q. Yun, Y. Ge, X. Zhang, Y. Chen and H. Zhang, Phase engineering of metal nanocatalysts for electrochemical CO2 reduction, eScience, 2022, 2, 467–485 CrossRef.
- D. D. Zhu, J. L. Liu and S. Z. Qiao, Recent Advances in Inorganic Heterogeneous Electrocatalysts for Reduction of Carbon Dioxide, Adv. Mater., 2016, 28, 3423–3452 CrossRef CAS PubMed.
- S. Jin, Z. Hao, K. Zhang, Z. Yan and J. Chen, Advances and challenges for the electrochemical reduction of CO2 to CO: From fundamentals to industrialization, Angew. Chem., Int. Ed., 2021, 60, 20627–20648 CrossRef CAS PubMed.
- H. Shin, K. U. Hansen and F. Jiao, Techno-economic assessment of low-temperature carbon dioxide electrolysis, Nat. Sustain., 2021, 4, 911–919 CrossRef.
- F. Chang, G. Zhan, Z. Wu, Y. Duan, S. Shi, S. Zeng, X. Zhang and S. Zhang, Technoeconomic Analysis and Process Design for CO2 Electroreduction to CO in Ionic Liquid Electrolyte, ACS Sustainable Chem. Eng., 2021, 9, 9045–9052 CrossRef CAS.
- M. Jouny, W. Luc and F. Jiao, General Techno-Economic Analysis of CO2 Electrolysis Systems, Ind. Eng. Chem. Res., 2018, 57, 2165–2177 CrossRef CAS.
- M. G. Kibria, J. P. Edwards, C. M. Gabardo, C.-T. Dinh, A. Seifitokaldani, D. Sinton and E. H. Sargent, Electrochemical CO2 Reduction into Chemical Feedstocks: From Mechanistic Electrocatalysis Models to System Design, Adv. Mater., 2019, 31, 1807166 CrossRef PubMed.
- T. Xu, B. Ma, J. Liang, L. Yue, Q. Liu, T. Li, H. Zhao, Y. Luo, S. Lu and X. Sun, Recent Progress in Metal-Free Electrocatalysts toward Ambient N2 Reduction Reaction, Acta Phys.-Chim. Sin., 2021, 37, 2009043 Search PubMed.
- Y. Wan, J. Xu and R. Lv, Heterogeneous electrocatalysts design for nitrogen reduction reaction under ambient conditions, Mater. Today, 2019, 27, 69–90 CrossRef CAS.
- Y. Wu, Z. Jiang, Z. Lin, Y. Liang and H. Wang, Direct electrosynthesis of methylamine from carbon dioxide and nitrate, Nat. Sustain., 2021, 4, 725–730 CrossRef.
- D. Wang, C. Chen and S. Wang, Defect engineering for advanced electrocatalytic conversion of nitrogen-containing molecules, Sci. China: Chem., 2022 DOI:10.1007/s11426-11022-11419-y.
- H. Wan, A. Bagger and J. Rossmeisl, Electrochemical Nitric Oxide Reduction on Metal Surfaces, Angew. Chem., Int. Ed., 2021, 60, 21966–21972 CrossRef CAS PubMed.
- H. Zhang, Y. Li, C. Cheng, J. Zhou, P. Yin, H. Wu, Z. Liang, J. Zhang, Q. Yun, A.-L. Wang, L. Zhu, B. Zhang, W. Cao, X. Meng, J. Xia, Y. Yu and Q. Lu, Isolated electron-rich ruthenium atoms in intermetallic compounds for boosting electrochemical nitric oxide reduction to ammonia, Angew. Chem., Int. Ed., 2022 DOI:10.1002/anie.202213351.
- Y. Zhang, Y. Wang, L. Han, S. Wang, T. Cui, Y. Yan, M. Xu, H. Duan, Y. Kuang and X. Sun, Nitrite electroreduction to ammonia promoted by molecular carbon dioxide with near-unity faradaic efficiency, Angew. Chem., Int. Ed., 2022 DOI:10.1002/anie.202213711.
- S. E. Braley, J. Xie, Y. Losovyj and J. M. Smith, Graphite conjugation of a macrocyclic cobalt complex enhances nitrite electroreduction to ammonia, J. Am. Chem. Soc., 2021, 143, 7203–7208 CrossRef CAS PubMed.
- P. Wang, H. Yang, C. Tang, Y. Wu, Y. Zheng, T. Cheng, K. Davey, X. Huang and S.-Z. Qiao, Boosting electrocatalytic CO2–to–ethanol production via asymmetric C–C coupling, Nat. Commun., 2022, 13, 3754 CrossRef CAS PubMed.
- Y. Li, F. Liu, Z. Chen, L. Shi, Z. Zhang, Y. Gong, Y. Zhang, X. Tian, Y. Zhang, X. Qiu, X. Ding, X. Bai, H. Jiang, Y. Zhu and J. Zhu, Perovskite-socketed sub-3 nm copper for enhanced CO2 electroreduction to C2+, Adv. Mater., 2022, 34, 2206002 CrossRef CAS PubMed.
- P. Li, J. Bi, J. Liu, Q. Zhu, C. Chen, X. Sun, J. Zhang and B. Han, In situ dual doping for constructing efficient CO2-to-methanol electrocatalysts, Nat. Commun., 2022, 13, 1965 CrossRef CAS PubMed.
- F.-Y. Chen, Z.-Y. Wu, S. Gupta, D. J. Rivera, S. V. Lambeets, S. Pecaut, J. Y. T. Kim, P. Zhu, Y. Z. Finfrock, D. M. Meira, G. King, G. Gao, W. Xu, D. A. Cullen, H. Zhou, Y. Han, D. E. Perea, C. L. Muhich and H. Wang, Efficient conversion of low-concentration nitrate sources into ammonia on a Ru-dispersed Cu nanowire electrocatalyst, Nat. Nanotechnol., 2022, 17, 759–767 CrossRef CAS PubMed.
- C. Lv, C. Yan, G. Chen, Y. Ding, J. Sun, Y. Zhou and G. Yu, An amorphous noble-metal-free electrocatalyst that enables nitrogen fixation under ambient conditions, Angew. Chem., Int. Ed., 2018, 57, 6073–6076 CrossRef CAS PubMed.
- C. Yang, B. Huang, S. Bai, Y. Feng, Q. Shao and X. Huang, A generalized surface chalcogenation strategy for boosting the electrochemical N2 fixation of metal nanocrystals, Adv. Mater., 2020, 32, 2001267 CrossRef CAS PubMed.
- W. Wang, Z. Wang, R. Yang, J. Duan, Y. Liu, A. Nie, H. Li, B. Y. Xia and T. Zhai, In situ phase separation into coupled interfaces for promoting CO2 electroreduction to formate over a wide potential window, Angew. Chem., Int. Ed., 2021, 60, 22940–22947 CrossRef CAS PubMed.
- J. Duan, T. Liu, Y. Zhao, R. Yang, Y. Zhao, W. Wang, Y. Liu, H. Li, Y. Li and T. Zhai, Active and conductive layer stacked superlattices for highly selective CO2 electroreduction, Nat. Commun., 2022, 13, 2039 CrossRef CAS PubMed.
- K. Sun, K. Yu, J. Fang, Z. Zhuang, X. Tan, Y. Wu, L. Zeng, Z. Zhuang, Y. Pan and C. Chen, Nature-inspired design of molybdenum–selenium dual-single-atom electrocatalysts for CO2 reduction, Adv. Mater., 2022, 34, 2206478 CrossRef CAS PubMed.
- Y. Zhou, Y. Yao, R. Zhao, X. Wang, Z. Fu, D. Wang, H. Wang, L. Zhao, W. Ni, Z. Yang and Y.-M. Yan, Stabilization of Cu+ via strong electronic interaction for selective and stable CO2 electroreduction, Angew. Chem., Int. Ed., 2022, 61, e202205832 CAS.
- Z.-H. Zhu, Z.-L. Liang, Z.-H. Jiao, X.-L. Jiang, Y. Xie, H. Xu and B. Zhao, A facile strategy to obtain low-cost and high-performance gold-based catalysts from artificial electronic waste by [Zr48Ni6] nano-cages in MOFs for CO2 electroreduction to CO, Angew. Chem., Int. Ed., 2022, 61, e202214243 CAS.
- L. Xu, X. Ma, L. Wu, X. Tan, X. Song, Q. Zhu, C. Chen, Q. Qian, Z. Liu, X. Sun, S. Liu and B. Han, In situ periodic regeneration of catalyst during CO2 electroreduction to C2+ products, Angew. Chem., Int. Ed., 2022, 61, e202210375 CAS.
- X. Sun, Q. Zhu, X. Kang, H. Liu, Q. Qian, Z. Zhang and B. Han, Molybdenum–bismuth bimetallic chalcogenide nanosheets for highly efficient electrocatalytic reduction of carbon dioxide to methanol, Angew. Chem., Int. Ed., 2016, 55, 6771–6775 CrossRef CAS PubMed.
- R. Wang, X. Wang, W. Weng, Y. Yao, P. Kidkhunthod, C. Wang, Y. Hou and J. Guo, Proton/electron donors enhancing electrocatalytic activity of supported conjugated microporous polymers for CO2 reduction, Angew. Chem., Int. Ed., 2022, 61, e202115503 CAS.
- M. Luo, Z. Wang, Y. C. Li, J. Li, F. Li, Y. Lum, D.-H. Nam, B. Chen, J. Wicks, A. Xu, T. Zhuang, W. R. Leow, X. Wang, C.-T. Dinh, Y. Wang, Y. Wang, D. Sinton and E. H. Sargent, Hydroxide promotes carbon dioxide electroreduction to ethanol on copper via tuning of adsorbed hydrogen, Nat. Commun., 2019, 10, 5814 CrossRef CAS PubMed.
- G. Fan, W. Xu, J. Li, J.-L. Chen, M. Yu, Y. Ni, S. Zhu, X.-C. Su and F. Cheng, Nanoporous NiSb to enhance nitrogen electroreduction via tailoring competitive adsorption sites, Adv. Mater., 2021, 33, 2101126 CrossRef CAS PubMed.
- W. Guo, S. Liu, X. Tan, R. Wu, X. Yan, C. Chen, Q. Zhu, L. Zheng, J. Ma, J. Zhang, Y. Huang, X. Sun and B. Han, Highly efficient CO2 electroreduction to methanol through atomically dispersed Sn coupled with defective CuO catalysts, Angew. Chem., Int. Ed., 2021, 60, 21979–21987 CrossRef CAS PubMed.
- Z. Zhang, G. Wen, D. Luo, B. Ren, Y. Zhu, R. Gao, H. Dou, G. Sun, M. Feng, Z. Bai, A. Yu and Z. Chen, “Two ships in a bottle” design for Zn–Ag–O catalyst enabling selective and long-lasting CO2 electroreduction, J. Am. Chem. Soc., 2021, 143, 6855–6864 CrossRef CAS PubMed.
- K.-Y. Wang, Z. Yang, J. Zhang, S. Banerjee, E. A. Joseph, Y.-C. Hsu, S. Yuan, L. Feng and H.-C. Zhou, Creating hierarchical pores in metal–organic frameworks via postsynthetic reactions, Nat. Protoc., 2022 DOI:10.1038/s41596-41022-00759-41597.
- W. Lin, H. Chen, G. Lin, S. Yao, Z. Zhang, J. Qi, M. Jing, W. Song, J. Li, X. Liu, J. Fu and S. Dai, Creating frustrated Lewis pairs in defective boron carbon nitride for electrocatalytic nitrogen reduction to ammonia, Angew. Chem., Int. Ed., 2022, 61, e202207807 CAS.
- Y. Kong, Y. Li, X. Sang, B. Yang, Z. Li, S. Zheng, Q. Zhang, S. Yao, X. Yang, L. Lei, S. Zhou, G. Wu and Y. Hou, Atomically dispersed Zinc(I) active sites to accelerate nitrogen reduction kinetics for ammonia electrosynthesis, Adv. Mater., 2022, 34, 2103548 CrossRef CAS PubMed.
- Y. Wang, W. Zhou, R. Jia, Y. Yu and B. Zhang, Unveiling the Activity Origin of a Copper-based Electrocatalyst for Selective Nitrate Reduction to Ammonia, Angew. Chem., Int. Ed., 2020, 59, 5350 CrossRef CAS PubMed.
- Y. Ma, J. Yu, M. Sun, B. Chen, X. Zhou, C. Ye, Z. Guan, W. Guo, G. Wang, S. Lu, D. Xia, Y. Wang, Z. He, L. Zheng, Q. Yun, L. Wang, J. Zhou, P. Lu, J. Yin, Y. Zhao, Z. Luo, L. Zhai, L. Liao, Z. Zhu, R. Ye, Y. Chen, Y. Lu, S. Xi, B. Huang, C.-S. Lee and Z. Fan, Confined growth of silver-copper Janus nanostructures with {100} facets for highly selective tandem electrocatalytic carbon dioxide reduction, Adv. Mater., 2022, 34, 2110607 CrossRef CAS PubMed.
- D. Chen, L.-H. Zhang, J. Du, H. Wang, J. Guo, J. Zhan, F. Li and F. Yu, A tandem strategy
for enhancing electrochemical CO2 reduction activity of single-atom Cu-S1N3 catalysts via integration with Cu nanoclusters, Angew. Chem., Int. Ed., 2021, 60, 24022–24027 CrossRef CAS PubMed.
- L. Lin, T. Liu, J. Xiao, H. Li, P. Wei, D. Gao, B. Nan, R. Si, G. Wang and X. Bao, Enhancing CO2 Electroreduction to Methane with a Cobalt Phthalocyanine and Zinc–Nitrogen–Carbon Tandem Catalyst, Angew. Chem. Int. Ed., 2020, 50, 22408–22413 CrossRef PubMed.
- T. Zhang, J. C. Bui, Z. Li, A. T. Bell, A. Z. Weber and J. Wu, Highly selective and productive reduction of carbon dioxide to multicarbon products via in situ CO management using segmented tandem electrodes, Nat. Catal., 2022, 5, 202–211 CrossRef CAS.
- W. He, J. Zhang, S. Dieckhöfer, S. Varhade, A. C. Brix, A. Lielpetere, S. Seisel, J. R. C. Junqueira and W. Schuhmann, Splicing the active phases of copper/cobalt-based catalysts achieves high-rate tandem electroreduction of nitrate to ammonia, Nat. Commun., 2022, 13, 1129 CrossRef CAS PubMed.
- C. Chen, X. Yan, Y. Wu, S. Liu, X. Zhang, X. Sun, Q. Zhu, H. Wu and B. Han, Boosting the productivity of electrochemical CO2 reduction to multi-carbon products by enhancing CO2 diffusion through a porous organic cage, Angew. Chem., Int. Ed., 2022, 61, e202202607 CAS.
- H. K. Lee, C. S. L. Koh, Y. H. Lee, C. Liu, I. Y. Phang, X. Han, C.-K. Tsung and X. Y. Ling, Favoring the unfavored: Selective electrochemical nitrogen fixation using a reticular chemistry approach, Sci. Adv., 2018, 4, eaar3208 CrossRef PubMed.
- W.-J. Sun, H.-Q. Ji, L.-X. Li, H.-Y. Zhang, Z.-K. Wang, J.-H. He and J.-M. Lu, Built-in electric field triggered interfacial accumulation effect for efficient nitrate removal at ultra-low concentration and electroreduction to ammonia, Angew. Chem., Int. Ed., 2021, 60, 22933–22939 CrossRef CAS PubMed.
- W. Qiu, X. Chen, Y. Liu, D. Xiao, P. Wang, R. Li, K. Liu, Z. Jin and P. Li, Confining intermediates within a catalytic nanoreactor facilitates nitrate-to-ammonia electrosynthesis, Appl. Catal., B, 2022, 315, 121548 CrossRef CAS.
- D. Yang, Q. Zhu and B. Han, Electroreduction of CO2 in ionic liquid-based electrolytes, The Innovation, 2020, 1, 100016 CrossRef PubMed.
- Y. Zhong, H. Xiong, J. Low, R. Long and Y. Xiong, Recent progress in electrochemical C–N coupling reactions, eScience, 2022 DOI:10.1016/j.esci.2022.1011.1002.
- R. Wang, S. Jia, L. Wu, X. Sun and B. Han, CO2–involved electrochemical C–N coupling into value-added chemicals, Chem. J. Chin. Univ., 2022, 43, 20220395 Search PubMed.
- S. Wang, T. Feng, Y. Wang and Y. Qiu, Recent Advances in Electrocarboxylation with CO2, Chem. – Asian J., 2022, 17, e202200543 CAS.
- Y. Wang, Z. Zhao, D. Pan, S. Wang, K. Jia, D. Ma, G. Yang, X.-S. Xue and Y. Qiu, Metal-free electrochemical carboxylation of organic halides in the presence of catalytic amounts of an organomediator, Angew. Chem., Int. Ed., 2022, 61, e202210201 CAS.
- X. Sun, Q. Zhu, J. Hu, X. Kang, J. Ma, H. Liu and B. Han, N,N–Dimethylation of nitrobenzenes with CO2 and water by electrocatalysis, Chem. Sci., 2017, 8, 5669–5674 RSC.
- Z.-H. Lyu, J. Fu, T. Tang, J. Zhang and J.-S. Hu, Design of ammonia oxidation electrocatalysts
for efficient direct ammonia fuel cells, EnergyChem, 2022 DOI:10.1016/j.enchem.2022.100093.
- D. Li, N. Xu, Y. Zhao, C. Zhou, L.-P. Zhang, L.-Z. Wu and T. Zhang, A Reliable and Precise Protocol for Urea Quantification in Photo/Electrocatalysis, Small Methods, 2022, 6, 2200561 CrossRef CAS PubMed.
- Y. Huang, Y. Wang, Y. Wu, Y. Yu and B. Zhang, Electrocatalytic construction of the C–N bond from the derivates of CO2 and N2, Sci. China: Chem., 2022, 65, 204–206 CrossRef CAS.
- X. Song, L. Xu, X. Sun and B. Han, In situ/operando characterization techniques for electrochemical CO2 reduction, Sci. China: Chem., 2022 DOI:10.1007/s11426-021-1463-6.
- Y. Zou and S. Wang, An Investigation of Active Sites for electrochemical CO2 Reduction Reactions: From In Situ Characterization to Rational Design, Adv. Sci., 2021, 8, 2003579 CrossRef CAS PubMed.
|
This journal is © Institute of Process Engineering of CAS 2023 |