Supercritical CO2-induced room-temperature ferromagnetism in two-dimensional MoO3−x†
Received
21st September 2022
, Accepted 25th November 2022
First published on 26th November 2022
Abstract
Two-dimensional (2D) magnetic semiconductors are crucial in spin-based information-processing technologies due to the combination of the strong 2D quantum effects, surface effects and the control of spin states. However, most experimental approaches for tuning 2D magnets achieve pure ferromagnetism at low temperature. Herein, a defect engineering strategy using supercritical CO2 is introduced to achieve nanostructure with abundant defects for 2D MoO3−x, and room-temperature ferromagnetism can be obtained and tuned by introduction of the Mo5+ ion depending on the change of supercritical pressure. In defective regions, the presence of the pentacoordinated [Mo5+O5] centers can achieve ferromagnetic ordering resulting in room-temperature ferromagnetism. With increasing supercritical pressure, it is easier for the supercritical CO2 to break the Mo–O bonds, achieving enhancement of the ferromagnetic performance with desired Curie temperature (>380 K). The magnetic responses in the MoO3−x system provide a step closer to the expansion of spin electronics.
Keywords: Supercritical CO2; Room-temperature ferromagnetism; Two-dimensional; MoO3−x.
1 Introduction
Room-temperature two-dimensional ferromagnets are at the forefront of research owing to the unusual physical and chemical properties that are useful for various applications, especially spintronics.1–3 In recent years, vigorous scientific inquiry has been undertaken on magnetic semiconductors, which possess both semiconductor and magnetic properties.4–7 2D transition-metal dichalcogenides (TMDs) and oxides (TMOs) with strong spin–orbit coupling are foreseen as promising candidates, such as MoS2, WS2, VSe2, VS2, ZnO and Ti2O3.8–13 However, because of the weak magnetic coupling or a lack of unpaired electrons, most of the pristine TMDs and TMOs are intrinsically nonmagnetic. Hence, exploring experimental approaches to modulate the magnetism are being pursued.
To date, systematic structural modulation methods to induce the signature of magnetic ordering are mainly classified as substitutional doping, phase transition, formation of surface dangling bonds, strain engineering and introduction of vacancies or defects.14–16 Among various 2D TMOs, because of tunable electrical properties, MoO3 has become attractive for achieving modulation of magnetic behavior. For example, room-temperature ferromagnetism of MoO3 was achieved by manipulating nanostructures such as nanofibers and hierarchical branches.17,18 And transition metal elements and non-metal elements doping can help the fabrication of ferromagnetism in Co-doped MoO3 films, and Ni- and Ni–Co doped MoO3 films, Te-doped MoO3 nanoflakes and hydrogen-doped MoO3 nanosheets.19–22 Due to the absence of unpaired electron spins, stoichiometric MoO3 and MoO2 are reported to be paramagnetic semiconductors.23 To expand the multifunctionality of spintronics devices where charge and spin manipulation could be combined, such as the novel magnetic tunnel junction, semiconductors with desirable magnetic nature have become more important; desirable properties include high saturation magnetization (Ms) under lower magnetic field, low coercivity field (Hc) and high Curie temperature (Tc).24 In fact, ferromagnetism can be manipulated by controlling the defects in 2D nanostructure, such as different magnetic properties depending on various defects in graphitic carbon.25–27
In supercritical fluid technology, as a green industrial solvent system, supercritical CO2 (SC CO2) can exfoliate various 2D layered materials, and in the interlayer confined space, CO2 can prompt diffusive atomic disordering to control the formation of defects because the generated local stress acts on the 2D surface.28,29 Defects strategy is beneficial for the introduction of unpaired electrons into the system, which is expected as an effective technical means to obtain 2D materials with ferromagnetic properties. Recently, non-van der Waals-like layered VO2 and BaTiO3 materials have exhibited superior ferromagnetism because of the induced symmetry breaking after high pressure treatment with SC CO2.30,31 Herein, for the first time, we report the successful fabrication of ferromagnetic 2D MoO3−x nanosheets with the assistance of SC CO2. And the magnetic moments in the defect regions result in ferromagnetic responses and the saturation magnetization reaches 0.01 emu g−1 under ∼2000 Oe magnetic field at room temperature, and the Curie temperature can reach to over 380 K.
2 Results and discussion
To verify the atomic arrangement of MoO3−x, transmission electron microscopy (TEM) is applied to observe the surface morphology. From the TEM image in Fig. 1a, the 2D sheet-like structure with irregular contour can be clearly visualized. The high resolution transmission electron microscopy (HRTEM) image (Fig. 1b) taken of the MoO3−x nanosheet shows an imperfect atomic arrangement with a (110) lattice distance of 0.38 nm. The ordered crystal lattices are separated by disordered regions. The fast Fourier transform (FFT) pattern also exhibits well-defined orthorhombic rings spots, which is consistent with layered α-MoO3−x. Moreover, according to the atomic force microscopy (AFM) analysis in Fig. 1c, the exfoliated MoO3−x nanosheets have a thickness of ∼4.5 nm (5–6 layers).32,33 The corresponding energy dispersive X-ray (EDX) elemental mapping images (Fig. 1d and e) further suggest the uniform distribution of Mo and O elements on the sheets. Thus, SC CO2 can destroy the Mo–O bonds on the surface, forming large amounts of defects and vacancies (Fig. 1f).
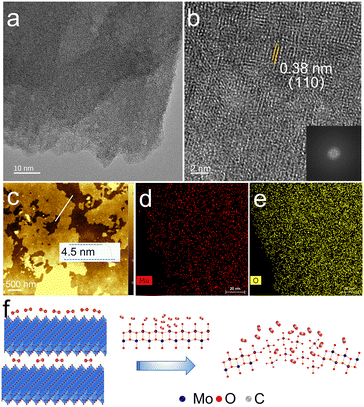 |
| Fig. 1 (a) TEM image of the MoO3−x nanosheets; (b) HRTEM image of the MoO3−x nanosheets, inset: the corresponding fast Fourier transform (FFT) pattern; (c) AFM image of the MoO3−x nanosheets; (d and e) elemental mapping of the MoO3−x nanosheets for Mo and O; (f) schematic side views showing the formation of MoO3−x using SC CO2. Mo, O, and C atoms are represented by balls in blue, red, and grey, respectively. | |
The MoO3−x shows abundant defects, which can be proven by the X-ray diffraction (XRD) patterns (Fig. 2a). Its X-ray powder diffraction pattern has a broad peak curve in the range of 20 to 30°, suggesting the existence of a disordered structure. Raman measurements at a low laser power were carried out (excitation wavelength: 532 nm). As can be seen in Fig. 2b, strong peaks occur at 818 and 991 cm−1 for the sample. The 818 cm−1 peak is assigned to the doubly coordinated oxygen (Mo2–O) stretching mode, and the peak at 991 cm−1 is attributable to the terminal oxygen (Mo
O) stretching mode. Other peaks at 334 and 374 cm−1 can be assigned to Mo3–O and Mo
O bending modes. And the broad trends of these peaks show the existence of lattice defects or the local disorder in MoO3−x.34,35 To elucidate the elemental composition and oxidation state of the MoO3−x, X-ray photoelectron spectroscopy (XPS) measurements were performed. For the XPS spectrum of Mo 3d, peaks correspond to Mo6+ (236.0 and 232.8 eV) and Mo5+ (234.8 and 231.6 eV), respectively (Fig. 2c).36,37 The proportion of Mo5+ can reach as high as 14.9%. The coexistence of Mo6+ and Mo5+ verifies the existence of oxygen vacancies. Fig. 2d shows the O 1s spectrum, the peaks at 530.5 eV and 532.4 eV correspond to oxygen in MoO6 and surface adsorbed species, respectively.38,39 And the energy level (531.6 eV) also suggests the existence of neighbouring oxygen vacancies.40 From the absorption spectrum of MoO3−x, the absorption in the visible–near infrared regions consists of three peaks at 685, 815 and 1050 nm, corresponding to 1.8, 1.5 and 1.2 eV, respectively. The three peaks originate from the intervalence charge transfer, the d–d transitions of Mo5+ and the surface plasmon resonance (SPR) of the MoO3−x nanosheets (Fig. 2e).41 It is concluded that the surface of MoO3−x possesses enough unsaturated atoms, resulting from oxygen vacancies.42,43 In order to investigate the oxygen vacancies and coordinately unsaturated Mo5+ atoms in Mo–O tetragonal pyramids of the MoO3−x nanosheets, electron paramagnetic resonance (EPR) spectrum measurements were carried out. Fig. 2f shows the hyperfine structure of Mo5+ with sharp peaks of parallel (g∥ = 1.898) and perpendicular (g⊥ = 1.937) bands and oxygen vacancies (g = 2.003), while these weakened peaks in crystalline MoO3 account for few unsaturated atoms.33
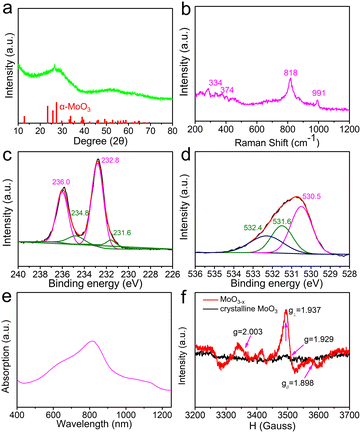 |
| Fig. 2 (a) XRD patterns of the MoO3−x nanosheets; (b) Raman scattering characteristics of the MoO3−x nanosheets; (c and d) XPS spectrum details of MoO3−x for Mo 3d and O 1s binding energy regions; (e) absorption spectrum of MoO3−x (concentration: 1 mg mL−1); (f) EPR spectrum of the MoO3−x nanosheets. | |
The photoluminescence (PL) spectrum at 300 K of the MoO3−x nanosheets is shown in Fig. 3a. Compared with crystalline MoO3, the PL spectrum of MoO3−x exhibits obvious emission and a blue-green emission peak centred at about 510 nm, which originates from the presence of the singly charged oxygen vacancy (Vo+).17 Vo+ defects with unpaired electrons can be the source of ferromagnetism, because they limit delocalization of unpaired electrons potentially giving rise to ferromagnetic double exchange. Owing to the inherent properties of layered MoO3−x, the net magnetic moment can be localized on the d orbital of the Mo atom.17 Moreover, the asymmetric nature of the photoluminescence spectrum at higher wavelengths can be ascribed to the intraband transitions occurring at the pentacoordinated [Mo5+O5] centers.19
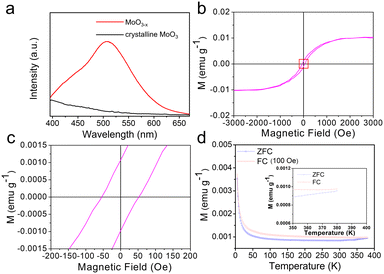 |
| Fig. 3 (a) PL emission spectra at 300 K of the MoO3−x nanosheets and crystalline MoO3 excited at 365 nm; (b) magnetization hysteresis loop of the MoO3−x nanosheets at 300 K; (c) the magnified curves near H = 0; (d) M–T curves of the MoO3−x nanosheets measured under ZFC and FC (H = 100 Oe) modes. | |
The magnetic properties of the MoO3−x nanosheets were studied by measuring the magnetization as a function of applied magnetic field (M–H) at 300 K. From Fig. 3b and c, the magnetic response of the sample with S-shaped and linear forms of the hysteresis loop shows ferromagnetic responses. Particularly, the ferromagnetic behavior is observed for low values of the magnetic excitation up to ∼2000 Oe. And the Ms is about 0.01 emu g−1. The magnified curves near H = 0 show that Hc and the remnant magnetization (Mr) are about 52 Oe and 0.001 emu g−1. As can be confirmed from Fig. 3d, the magnetization vs. temperature (M–T) curves further substantiate room-temperature ferromagnetism in MoO3−x nanosheets, and the zero-field-cooled (ZFC) and field-cooled (FC) curves show obvious splitting over the whole measurement temperature range, indicating the Curie temperature (TC) is >380 K.19 To compare with the reported oxide series (MoO3–MoO3−x–MoO2) and their doped compounds, the experimental magnetic parameters at room temperature are given in Table S1 (see ESI†). The Ms, Mr and Tc of the as-prepared MoO3−x are outstanding, meanwhile, Hc can maintain a low value. Importantly, the yield is around 10%, and the preparation process is expected to recycle CO2 for the large-scale production of ferromagnetic MoO3−x, which has potential for practical applications.
To further identify the magnetic source, it is necessary to study the magnetic properties of SC CO2-treated samples at different pressures. The XRD patterns (Fig. 4a) show that the crystal structure of the nanosheets is more disordered with an increase in SC CO2 pressure except for that of 16 MPa, owing to a phase transition into the hexagonal phase for MoO3−x (h-MoO3−x).44 Moreover, from their corresponding HRTEM images (Fig. 4b–d), it can be found that the atomic arrangements are all more perfect than that of the MoO3−x obtained at 14 MPa. Among them, the lowest degree of defects can be found for MoO3−x obtained at 10 MPa, which indicates the SC CO2 destroys the atomic arrangement under high enough pressure conditions. Additionally, h-MoO3−x with a (300) lattice distance of 0.30 nm for the 16 MPa sample is observed clearly, which is agreeing with the XRD results. In addition, the variation of atomic structures is also analyzed by XPS. From the XPS spectrum analysis (Fig. 5), compared to that of samples obtained at 14 MPa (14.9%), the proportions of Mo5+ for MoO3−x obtained at 10, 12 and 16 MPa are 5.2%, 9.2% and 14.6% respectively, which demonstrates the introduction of more Mo5+ with increase in SC CO2 pressure. It is known that the Mo5+ ion has an unpaired electron spin (i.e., Mo5+: [Kr]4d1), so the presence of the pentacoordinated [Mo5+O5] centers can also provide net magnetic moments in addition to the introduction of the oxygen vacancies in MoO3.19
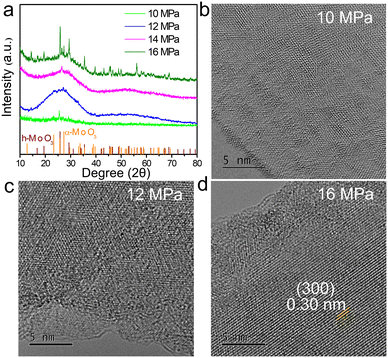 |
| Fig. 4 (a) XRD patterns comparison of the MoO3−x obtained at different pressures, and XRD patterns at the bottom show α-MoO3 (JCPDS No. 05-0508) and h-MoO3 (JCPDS No. 21-0569); (b–d) HRTEM images of the MoO3−x sample obtained at 10 MPa, 12 MPa, 16 MPa. | |
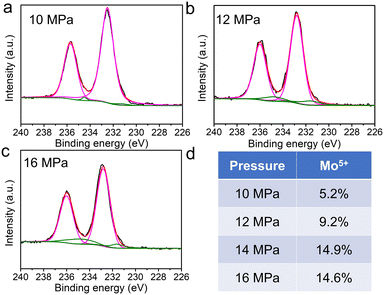 |
| Fig. 5 (a–c) XPS spectrum details of the Mo 3d binding energy regions and (d) the varied percent of Mo5+ for the MoO3−x sample obtained at 10 MPa, 12 MPa, 16 MPa. | |
In addition, the variation trend of the Mo5+ component is also partly reflected in their EPR spectra (Fig. 6a). It can be observed that the unpaired electron spin signal strength of Mo5+ in the samples becomes strong with the increase in pressure, and 14 MPa is the strongest when compared. And there are no obvious signal responses of oxygen vacancies for these samples obtained at 10, 12 and 16 MPa, which could be disadvantageous to ferromagnetic performance. Further, their magnetic behaviors are also confirmed by M–H loop measurements (Fig. 6b), and it shows a paramagnetic response for crystalline MoO3, differing from the ferromagnetism of the MoO3−x obtained at 12, 14 and 16 MPa.
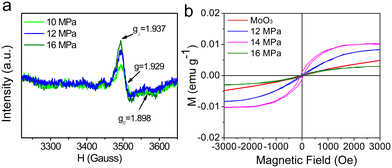 |
| Fig. 6 (a) EPR spectra comparison of MoO3−x obtained at different pressures; (b) magnetization hysteresis loop comparison of the MoO3−x sample obtained at 12 MPa, 14 MPa, 16 MPa and crystalline MoO3 at 300 K. | |
It is concluded that both Mo5+ and oxygen vacancies contribute to the achievement of ferromagnetism, which can be tuned by SC CO2 pressure, because the bound magnetic polarons could be formed via Mo5+ coupling with the charged oxygen vacancies.18 Meanwhile, the Ms and Mr reduce remarkably for the sample at 16 MPa (Fig. S1†), which shows the necessity of 2D structure. Moreover, there is less Mo5+ and a weak ferromagnetic response for the MoO3−x nanosheets obtained by sonication treatment without SC CO2 (Fig. S2†). Therefore, through defect engineering by SC CO2, the Mo5+ can induce polarized spins, which is critical to the magnetic properties for 2D molybdenum oxide materials.
3 Conclusions
In summary, a defect engineering strategy using SC CO2 is presented to endow nonmagnetic 2D materials with ferromagnetic properties. The 2D MoO3−x nanosheets are selected as a model, in which the introduction of moderate Mo5+ provides the MoO3−x nanosheets with ferromagnetic behavior at room temperature, resulting from magnetic polarons in defective regions. And the MoO3−x nanosheets exhibit a large saturation magnetization (0.01 emu g−1) and TC (>380 K). Moreover, SC CO2 can tune the magnetic properties achieving continuous enhancement of ferromagnetic performance with increasing supercritical CO2 pressure below the phase transformation pressure, and 14 MPa is the optimum pressure. Undoubtedly, the strategy is feasible to stimulate the ferromagnetic characteristics of other kinds of 2D materials for more capabilities and higher performances of spintronics devices in complex magnetic environments.
4 Experimental section
4.1 Materials and methods
4.1.1 Materials.
MoS2 powder was purchased from Sigma-Aldrich (Fluka, product number 69860). Analytical grade ethanol was provided by Sinopharm Chemical Reagent Co., Ltd. All were directly used owing to their analytical grade. CO2 with a purity of 99.99% was purchased from the Zhengzhou Shuangyang Gas Co. Deionized water was prepared with double-distilled water.
4.1.2 Preparation of MoO3−x nanosheets.
MoS2 powder (100 mg) was first annealed at 623 K for 90 min in the air. The obtained MoO3 was dispersed in a 45% ethanol/water mixture (10 mL) and subsequently was sonicated for 1 h in a water bath (equipment power: 200 W), forming a uniform black dispersion. Then the dispersion was quickly transferred into the supercritical CO2 apparatus composed mainly of a stainless-steel autoclave with a heating jacket and a temperature controller. The autoclave was heated to 353 K, and then CO2 was charged into reactor to the set pressure (e.g. 10, 12, 14 and 16 MPa). The autoclave was maintained at 353 K for 3 h and then cooled to room temperature. The dispersion was centrifuged at 6000 rpm for 15 min to remove aggregates, and the supernatant was collected. Then the solution was dried in an oven at a constant temperature of 333 K.
4.1.3 Characterization.
TEM images were obtained on a JEOL JEM 2100F transmission electron microscope at an acceleration voltage of 200 kV. The XRD patterns were examined using a Bruker D8 Advance diffractometer using germanium monochromatic CuKα radiation (40 kV and 40 mA). The thickness of the nanosheets was measured using AFM (NanoManVS). Raman spectra of the samples were measured using a LabRAM HR Evolution with 532 nm laser excitation. XPS measurements were performed on a Thermo ESCALAB 250XI platform. Magnetic properties were characterized using a superconducting quantum interference device (SQUID)-magnetic property measurement system (MPMS)-3. UV–vis–NIR spectroscopy was carried out with a Shimadzu UV-240/PC. The PL spectra were recorded at room temperature on a Hitachi F-4500 spectrophotometer.
Conflicts of interest
There are no conflicts to declare.
Acknowledgements
We are grateful to the National Natural Science Foundation of China (No. 21773216, 51173170), the Henan-Provincial and the China-National Natural Science United Foundation (Project No. U2004208).
References
- K. S. Burch, D. Mandrus and J. G. Park, Magnetism in two-dimensional van der Waals materials, Nature, 2018, 563, 47–52 CrossRef CAS PubMed.
- Y. Liu, J. Guo, A. Yu, Y. Zhang, J. Kou, K. Zhang, R. Wen, Y. Zhang, J. Zhai and Z. L. Wang, Magnetic-induced-piezopotential gated MoS2 field-effect transistor at room temperature, Adv. Mater., 2018, 30, 1704524 CrossRef PubMed.
- W. Wang, M. W. Daniels, Z. Liao, Y. Zhao, J. Wang, G. Koster, G. Rijnders, C.-Z. Chang, D. Xiao and W. Wu, Spin chirality fluctuation in two-dimensional ferromagnets with perpendicular magnetic anisotropy, Nat. Mater., 2019, 18, 1054–1059 CrossRef CAS PubMed.
- M. Gibertini, M. Koperski, A. F. Morpurgo and K. S. Novoselov, Magnetic 2D materials and heterostructures, Nat. Nanotechnol., 2019, 14, 408–419 CrossRef CAS PubMed.
- T. Song, Z. Fei, M. Yankowitz, Z. Lin, Q. Jiang, K. Hwangbo, Q. Zhang, B. Sun, T. Taniguchi, K. Watanabe, M. A. McGuire, D. Graf, T. Cao, J.-H. Chu, D. H. Cobden, C. R. Dean, D. Xiao and X. Xu, Switching 2D magnetic states via pressure tuning of layer stacking, Nat. Mater., 2019, 18, 1298–1302 CrossRef CAS PubMed.
- N. Sivadas, S. Okamoto, X. Xu, C. J. Fennie and D. Xiao, Stacking-dependent magnetism in bilayer CrI3, Nano Lett., 2018, 18, 7658–7664 CrossRef CAS PubMed.
- M. Bonilla, S. Kolekar, Y. Ma, H. C. Diaz, V. Kalappattil, R. Das, T. Eggers, H. R. Gutierrez, M.-H. Phan and M. Batzill, Strong room-temperature ferromagnetism in VSe2 monolayers on van der Waals substrates, Nat. Nanotechnol., 2018, 13, 289–293 CrossRef CAS.
- W. Yu, J. Li, T. S. Herng, Z. Wang, X. Zhao, X. Chi, W. Fu, I. Abdelwahab, J. Zhou, J. Dan, Z. Chen, Z. Chen, Z. Li, J. Lu, S. J. Pennycook, Y. P. Feng, J. Ding and K. P. Loh, Chemically exfoliated VSe2 monolayers with room-temperature ferromagnetism, Adv. Mater., 2019, 31, 1903779 CrossRef CAS PubMed.
- Y. Li, Y. Weng, X. Yin, X. Yu, S. R. S. Kumar, N. Wehbe, H. Wu, H. N. Alshareef, S. J. Pennycook, M. B. H. Breese, J. Chen, S. Dong and T. Wu, Orthorhombic Ti2O3: a polymorph-dependent narrow-bandgap ferromagnetic oxide, Adv. Funct. Mater., 2018, 28, 1705657 CrossRef.
- H. Tan, C. Wang, W. Hu, H. Duan, P. Guo, N. Li, G. Li, L. Cai, Z. Sun, F. Hu and W. Yan, Reversible tuning of the ferromagnetic behavior in Mn-doped MoS2 nanosheets via interface charge transfer, ACS Appl. Mater. Interfaces, 2018, 10, 31648–31654 CrossRef CAS.
- Y. Guo, H. Deng, X. Sun, X. Li, J. Zhao, J. Wu, W. Chu, S. Zhang, H. Pan, X. Zheng, X. Wu, C. Jin, C. Wu and Y. Xie, Modulation of metal and insulator states in 2D ferromagnetic VS2 by van der Waals interaction engineering, Adv. Mater., 2017, 29, 1700715 CrossRef PubMed.
- T. Taniguchi, K. Yamaguchi, A. Shigeta, Y. Matsuda, S. Hayami, T. Shimizu, T. Matsui, T. Yamazaki, A. Funatstu, Y. Makinose, N. Matsushita, M. Koinuma and Y. Matsumoto, Enhanced and engineered d0 ferromagnetism in molecularly-thin zinc oxide nanosheets, Adv. Funct. Mater., 2013, 23, 3140–3145 CrossRef CAS.
- J. Luxa, O. Jankovsky, D. Sedmidubsky, R. Medlin, M. Marysko, M. Pumera and Z. Sofer, Origin of exotic ferromagnetic behavior in exfoliated layered transition metal dichalcogenides MoS2 and WS2, Nanoscale, 2016, 8, 1960–1967 RSC.
- R. Sanikop and C. Sudakar, Tailoring magnetically active defect sites in MoS2 nanosheets for spintronics applications, ACS Appl. Nano Mater., 2020, 3, 576–587 CrossRef CAS.
- S. Yan, W. Qiao, X. He, X. Guo, L. Xi, W. Zhong and Y. Du, Enhancement of magnetism by structural phase transition in MoS2, Appl. Phys. Lett., 2015, 106, 012408 CrossRef.
- Z. Zhang, X. Zou, V. H. Crespi and B. I. Yakobson, Intrinsic magnetism of grain boundaries in two-dimensional metal dichalcogenides, ACS Nano, 2013, 7, 10475–10481 CrossRef CAS PubMed.
- S. K. S. Patel, K. Dewangan and N. S. Gajbhiye, Synthesis and room temperature d0 ferromagnetic properties of α-MoO3 nanofibers, J. Mater. Sci. Technol., 2015, 31, 453–457 CrossRef CAS.
- Y. Mao, W. Li, X. Sun, Y. Ma, J. Xia, Y. Zhao, X. Lu, J. Gan, Z. Liu, J. Chen, P. Liu and Y. Tong, Room-temperature ferromagnetism in hierarchically branched MoO3 nanostructures, CrystEngComm, 2012, 14, 1419–1424 RSC.
- D. J. Lee, Y. Lee, Y. H. Kwon, S. H. Choi, W. Yang, D. Y. Kim and S. Lee, Room-temperature ferromagnetic ultrathin α-MoO3:Te nanoflakes, ACS Nano, 2019, 13, 8717–8724 CrossRef CAS PubMed.
- O. Kamoun, A. Boukhachem, S. Alleg, B. Jeyadevan and M. Amlouk, Physical study of nano-structured MoO3 films codoped with cobalt and nickel in which there is a ferro-diamagnetic transition, J. Alloys Compd., 2018, 741, 847–854 CrossRef CAS.
- J. Zhang, J. Fu, F. Shi, Y. Peng, M. Si, L. Cavallo and Z. Cao, Hydrogen atom induced magnetic behaviors in two-dimensional materials: insight on origination in the model of α-MoO3, Nanoscale, 2018, 10, 14100–14106 RSC.
- A. Boukhachem, M. Mokhtari, N. Benameur, A. Ziouche, M. Martínez, P. Petkova, M. Ghamnia, A. Cobo, M. Zergoug and M. Amlouk, Structural optical magnetic properties of Co doped α-MoO3 sprayed thin films, Sens. Actuators, A, 2017, 253, 198–209 CrossRef CAS.
- M. A. Khilla, H. Mikhail, A. A.-E. Soud and Z. M. Hanafi, Magnetic susceptibility of molybdenum trioxide, dioxide and some suboxides, Czech. J. Phys. B, 1980, 30, 1039–1045 CrossRef.
- C. Gong and X. Zhang, Two-dimensional magnetic crystals and emergent heterostructure devices, Science, 2019, 363, eaav4450 CrossRef CAS PubMed.
- S.-M. Jung, J. Park, D. Shin, H. Y. Jeong, D. Lee, I.-Y. Jeon, H. Cho, N. Park, J.-W. Yoo and J.-B. Baek, Paramagnetic carbon nanosheets with random hole defects and oxygenated functional groups, Angew. Chem., Int. Ed., 2019, 58, 11670–11675 CrossRef CAS PubMed.
- R. R. Nair, M. Sepioni, I.
L. Tsai, O. Lehtinen, J. Keinonen, A. V. Krasheninnikov, T. Thomson, A. K. Geim and I. V. Grigorieva, Spin-half paramagnetism in graphene induced by point defects, Nat. Phys., 2012, 8, 199–202 Search PubMed.
- J. Červenka, M. I. Katsnelson and C. F. J. Flipse, Room-temperature ferromagnetism in graphite driven by two-dimensional networks of point defects, Nat. Phys., 2009, 5, 840–844 Search PubMed.
- W. Liu, Q. Xu, W. Cui, C. Zhu and Y. Qi, CO2-assisted fabrication of two-dimensional amorphous molybdenum oxide nanosheets for enhanced plasmon resonances, Angew. Chem., Int. Ed., 2017, 56, 1600–1604 CrossRef CAS PubMed.
- Y. Ren, C. Li, Q. Xu, J. Yan, Y. Li, P. Yuan, H. Xia, C. Niu, X. Yang and Y. Jia, Two-dimensional amorphous heterostructures of Ag/a-WO3-x for high-efficiency photocatalytic performance, Appl. Catal., B, 2019, 245, 648–655 CrossRef CAS.
- Y. Zhou, P. Yan, S. Zhang, C. Ma, T. Ge, X. Zheng, L. Zhang, J. Jiang, Y. Shen, J. Chen and Q. Xu, Conversion of non-van der Waals VO2 solid to 2D ferromagnet by CO2-induced phase engineering, Nano Today, 2021, 40, 101272 CrossRef CAS.
- B. Gao, S. Xu and Q. Xu, CO2-induced exposure of the intrinsic magnetic surface of BaTiO3 to give room-temperature ferromagnetism, Angew. Chem., 2022, 61, e202117084 CAS.
- I. A. de Castro, R. S. Datta, J. Z. Ou, A. Castellanos-Gomez, S. Sriram, T. Daeneke and K. Kalantar-Zadeh, Molybdenum oxides – from fundamentals to functionality, Adv. Mater., 2017, 29, 1701619 CrossRef PubMed.
- W. Liu, C. Li, Q. Xu, P. Yan, C. Niu, Y. Shen, P. Yuan and Y. Jia, Anderson localization in 2D amorphous MoO3-x monolayers for electrochemical ammonia synthesis, ChemCatChem, 2019, 11, 5412–5416 CrossRef CAS.
- K. Ajito, L. A. Nagahara, D. A. Tryk, K. Hashimoto and A. Fujishima, Study of the photochromic properties of amorphous MoO3 films using Raman microscopy, J. Phys. Chem., 1995, 99, 16383–16388 CrossRef CAS.
- S. Balendhran, J. Deng, J. Z. Ou, S. Walia, J. Scott, J. Tang, K. L. Wang, M. R. Field, S. Russo, S. Zhuiykov, M. S. Strano, N. Medhekar, S. Sriram, M. Bhaskaran and K. Kalantar-zadeh, Enhanced charge carrier mobility in two-dimensional high dielectric molybdenum oxide, Adv. Mater., 2013, 25, 109–114 CrossRef CAS PubMed.
- J. Li, Y. Ye, L. Ye, F. Su, Z. Ma, J. Huang, H. Xie, D. E. Doronkin, A. Zimina, J.-D. Grunwaldt and Y. Zhou, Sunlight induced photo-thermal synergistic catalytic CO2 conversion via localized surface plasmon resonance of MoO3−x, J. Mater. Chem. A, 2019, 7, 2821–2830 RSC.
- W. Liu, Q. Xu, P. Yan, J. Chen, Y. Du, S. Chu and J. Wang, Fabrication of a single-atom platinum catalyst for the hydrogen evolution reaction: a new protocol by utilization of HxMoO3−x with plasmon resonance, ChemCatChem, 2018, 10, 946–950 CrossRef CAS.
- Y. Li, X. Chen, M. Zhang, Y. Zhu, W. Ren, Z. Mei, M. Gu and F. Pan, Oxygen vacancy-rich MoO3−x nanobelts for photocatalytic N2 reduction to NH3 in pure water, Catal. Sci. Technol., 2019, 9, 803–810 RSC.
- A. R. Head, C. Gattinoni, L. Trotochaud, Y. Yu, O. Karslıoğlu, S. Pletincx, B. Eichhorn and H. Bluhm, Water (non-)interaction with MoO3, J. Phys. Chem. C, 2019, 123, 16836–16842 CrossRef CAS PubMed.
- Z. Luo, R. Miao, T. D. Huan, I. M. Mosa, A. S. Poyraz, W. Zhong, J. E. Cloud, D. A. Kriz, S. Thanneeru, J. He, Y. Zhang, R. Ramprasad and S. L. Suib, Mesoporous MoO3-x material as an efficient electrocatalyst for hydrogen evolution reactions, Adv. Energy Mater., 2016, 6, 1600528 CrossRef.
- T. Ge, Z. Wei, X. Zheng, P. Yan and Q. Xu, Atomic rearrangement and amorphization induced by carbon dioxide in two-dimensional MoO3–x nanomaterials, J. Phys. Chem. Lett., 2021, 12, 6543–6550 CrossRef CAS PubMed.
- H. Cheng, X. Qian, Y. Kuwahara, K. Mori and H. Yamashita, A plasmonic molybdenum oxide hybrid with reversible tunability for visible-light-enhanced catalytic reactions, Adv. Mater., 2015, 27, 4616–4621 CrossRef CAS PubMed.
- H. Cheng, M. Wen, X. Ma, Y. Kuwahara, K. Mori, Y. Dai, B. Huang and H. Yamashita, Hydrogen doped metal oxide semiconductors with exceptional and tunable localized surface plasmon resonances, J. Am. Chem. Soc., 2016, 138, 9316–9324 CrossRef CAS PubMed.
- W. Liu and Q. Xu, Fabrication of Ag/h-MoO3 with surface plasmon resonances for enhanced photoelectrochemical performance, Sol. RRL, 2019, 3, 1900242 CrossRef CAS.
|
This journal is © Institute of Process Engineering of CAS 2023 |