DOI:
10.1039/D3GC00649B
(Paper)
Green Chem., 2023,
25, 4319-4325
Mechanochemical Simmons–Smith cyclopropanation via ball-milling-enabled activation of zinc(0)†
Received
23rd February 2023
, Accepted 26th April 2023
First published on 15th May 2023
Abstract
The bulk solvent-free synthesis of cyclopropanes via a ball-milling-enabled Simmons–Smith reaction is herein described. Harnessing the use of mechanochemical techniques to activate zinc(0), this, all under air and operationally simple protocol, allows the synthesis of a wide range of cyclopropane structures and scale up to enable gram-scale.
Introduction
The transformation of organohalide species into reactive organometallic nucleophiles – and resulting reactivity – is one of the most common disconnections in organic chemistry.1 Within this context, organomagnesium, organolithium, and organozinc species dominate the literature precedent due to their well-documented efficiency. Despite this, there can remain issues with the generation and use of these valuable nucleophiles (Scheme 1A).2 Notably capricious activation of zero-valent magnesium or zinc3 can result in a range of reagent concentrations in solution, and there often remains a necessity for inert gas protection.4 Furthermore, these organometallic species are almost in their entirety synthesised and stored as solutions in appropriate solvents leading to increased usage (ergo waste) of solvents (n-hexane, Et2O, THF).5
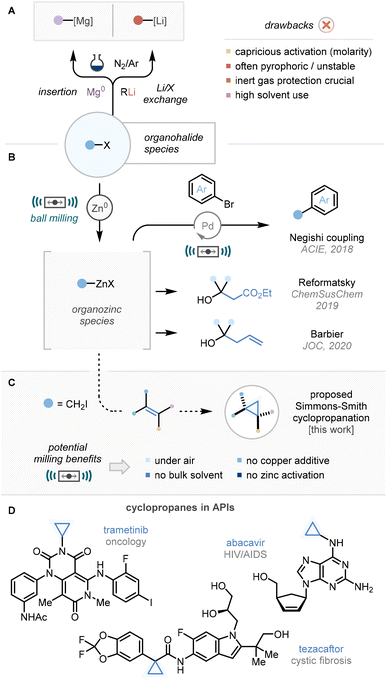 |
| Scheme 1 Mechanochemical activation of zinc(0) for synthesis of organozinc species and application in organic synthesis. (A) solution-based/traditional approaches; (B) mechanochemical approach; (C) this work; (D) cyclopropanes in APIs. | |
One technique which has enabled the reduction of solvent use is the mechanochemical manipulation of materials in a solvent-free or highly solvent-minimised environment via ball milling technology.6 Moreover, in contrast to solution-phase counterparts, mechanochemical protocols can often be carried out without recourse to air/moisture-sensitive set-ups.7 Within the context of organometallic generation, recent work has established that analogous organozinc species can be formed via milling a suitable organohalide with zero-valent zinc metal.
These procedures can be carried out without need of exogenous activation procedures,8 bypassing pyrophoric intermediates such as finely ground magnesium,9 all under air atmospheres, and in the absence of bulk solvent. Using this technology, our group has leveraged protocols for the ball-milling-enabled Negishi,10 Reformatsky,11 and Barbier12 reactions (Scheme 1B). Also notable are recent endeavours in the mechanochemical generation and utility of organomagnesium, organocalcium and organomanganese species.6,13
In a continuation of our programme towards sustainable formation and manipulation of organozinc species, a mechanochemical Simmons–Smith cyclopropanation method was targeted (Scheme 1C).14 New methods for the generation of highly strained ring systems are important, for example cyclopropane moieties are surging in prevalence in the development of active pharmaceuticals such as trametinib (oncology, Scheme 1D), Abacavir (HIV/AIDS), and tezacaftor (CF).15 This is primarily due to favourable metabolic stability and opportunities for c
log
P modulation.16 To this end, an operationally simple, bulk-solvent-free cyclopropanation protocol without requirement for air/moisture-sensitive reaction set-ups, undesired use of pyrophoric dialkyl zincs, or milling of diazo compounds17 would be of interest to the sustainable chemistry community. Herein we wish to report our findings.
Results and discussion
Optimization studies
Our studies into a mechanochemical protocol for the cyclopropanation of alkenes commenced by identifying trans-cinnamyl alcohol (1a) as a model substrate. Preliminary scouting studies (see ESI† for full details) revealed that milling 1a with zinc(0) (granular 20–30 mesh), diiodomethane (2a, as the carbenoid precursor) and copper(0) (0.5 eq.) using a mixer mill at 30 Hz for 1 hour gave promising yields of the cyclopropanated product 3a (Scheme 2, entry 1, 59%). Our previous work9 on mechanochemical organozinc formation has demonstrated that the addition of N,N′-dimethylacetamide (DMA) as a liquid assisted grinding additive proved crucial to high reaction efficiency. In this case, whilst a pleasing increase was observed on the addition of DMA (entry 2, 76%, 2.5 equivalents of DMA), further improvement was observed on employing more environment-acceptable alternatives 2-MeTHF (entry 4, 83%), and EtOAc (entry 5, 81%). Interestingly, and conversely to our studies in the absence of LAG (see ESI†) we found that removing the copper additive led to little detrimental effect to the reaction efficiency, affording 3a in 77% yield (entry 6).18 Furthermore, reducing quantities of 2-MeTHF to near equimolar concentrations led to optimal conditions, delivering the cyclopropane product in 94% isolated yield (entry 7). Further studies revealed reducing the quantities of CH2I2 or Zn(0) to 4 equivalents each proved damaging to reaction efficiency (entry 8).
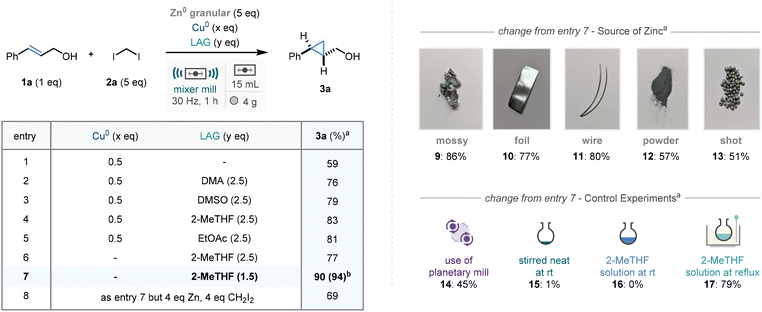 |
| Scheme 2 Optimization of the reaction conditions for the mechanochemical Simmons–Smith reaction and control experiments: aCalculated via1H NMR analysis of the crude reaction mixture against 1,3,5-trimethoxybenzene as an internal standard. bIsolated yield after silica gel column chromatography. | |
Our prior reports have demonstrated that mechanochemical techniques to activate raw-form zinc(0) is largely form-independent. To validate this observation in this methodology, five further zinc(0) forms were employed in the reaction protocol: mossy, foil, powder, wire, and shot. All five of these metal inputs were reliable in this ball-milling manifold, affording 3a in excellent yields in three cases (mossy, foil, wire – entries 9–11, 77–86%) and moderate yields in the other two (powder, shot – entries 12 and 13, 51–57%)
Following this, translation of the methodology to other reaction vessels, serving as control experiments, was explored. Use of a planetary mill (using zirconia jars and ball, see ESI† for more details), demonstrated reasonable reaction efficiency (entry 14, 45%), which not only offers reactivity in other mechanochemical set-ups but can be used to demonstrate that reactivity is maintained in a “stainless steel-free” environment, highlighting that the stainless-steel material used to fabricate the mixer mill jars is not necessarily mechanistically relevant.19
Subsequently a subset of reactions were conducted in standard round-bottomed flask glassware. Notably, stirring the reaction mixture neat or in 2-MeTHF solution led to no conversion to the cyclopropane product 3a (entries 15 and 16).
Despite this, remarkably, without employment any zinc(0) activation methods a large quantity of the product was formed when heating at reflux in 2-MeTHF solution (entry 13, 79% yield). With this in mind, temperature monitoring studies of our milled reaction demonstrate a maximum temperature of 31 °C, suggesting that the zinc(0) activation in our protocol is mechanical rather than thermal (see ESI† for temperature–time data).
Reaction scope
With optimal conditions in hand for the mechanochemical solvent-minimised alkene cyclopropanation methodology, the scope and limitations of the reaction were explored.20 The cyclopropanation method proceeded with exquisite diastereoselectivity with the concerted pathway leading to cyclopropane products mimicking the stereochemical orientation of the alkene starting material (e.g. (E)-alkenes lead to trans diastereomers).
Initially a trisubstituted version of our model substrate was studied, affording the cyclopropane in high yields (Scheme 3A, 3b, 81%). The presence of the alcoholic OH to direct the cyclopropanation was shown not to be critical to reactivity, with alkyl and silyl ethers demonstrating excellent reaction efficiency (3c–3e, 85–92%), although with reduction in yields for THP-protected alcohol (3f, 28%), where a complex mixture of products was observed in conjunction with the product. Further arene and benzyl substituted allylic alcohols participated well in the reaction methodology (3g–i) with modest yields observed with secondary alcohol 3i. Following this, carbocyclic rings bearing endo- and exo-cyclic alkenes were excellent substrates for cyclopropanation (3j–m, 60–94%), and unsurprisingly proceeded with exquisite diastereoselectivity cis to the oxygen-centred appendage.
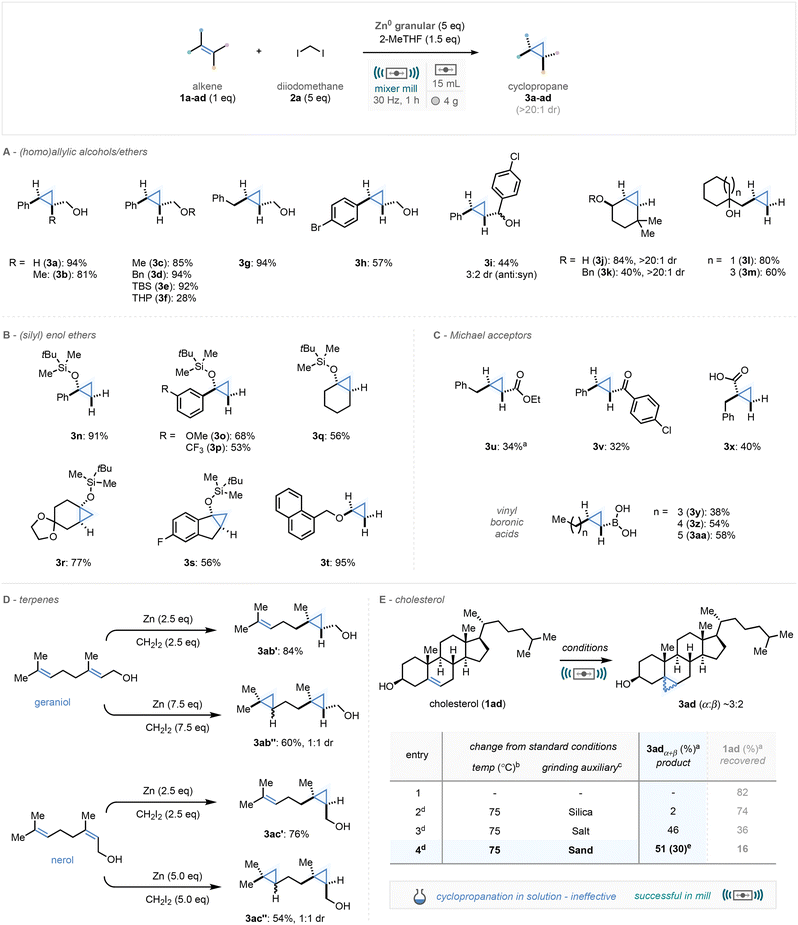 |
| Scheme 3 Scope of the mechanochemical Simmons–Smith reaction. Isolated yields after purification are reported unless otherwise stated. aYields based on 1H NMR analysis of the crude reaction mixture vs. 1,3,5-trimethoxybenzene as an internal standard. bUsing a PID-controlled programmable jar heater, see ESI† for details. c1 mass equivalent of the sum of all reaction components was added. d30 mL jar and a 9 g ball was used. eIsolated yield after silica gel column chromatography and epoxidation work-up to remove remaining alkene starting material. | |
Cyclopropanation of a range of silyl enol ethers proceeded efficiently on both acyclic and cyclic structures (Scheme 3B, 3n–s, 53–91%), in the latter cases affording fused bicyclic architectures in good yields (3q–s). Furthermore, a naphthyl-substituted alkyl enol ether was very effective in this methodology, affording the cyclopropyl ether in 95% yield (3t).
A selection of α,β-unsaturated carbonyl species (Michael acceptors, Scheme 3C) were surveyed and gave cyclopropane products in modest yields (3u–x, 34–40%), and subsequently a small selection of vinyl boronic acids proceeded in good yields under our reaction conditions (3y–3aa, 38–58%) affording the cyclopropyl boronic acid species which could be further used in cross coupling methodology.
Two terpene natural products – bearing two alkene moieties – geraniol and nerol were chosen to explore chemoselective mono- and di-cyclopropanation (Scheme 3D). Impressively through control of stoichiometry we could access both mono-cyclopropanated and di-cyclopropanated species (3ab–ac) with the allylic alcohol reaction proceeding first. Notably, in the case of the two double cyclopropanation systems, both ring formations were shown to take place in just 1 hour of milling time.
Finally, we turned our attention towards the Simmons–Smith reaction of cholesterol, a reaction unknown in solution, and unsuccessful in our own hands in solution using conditions from Scheme 2 (entry 17). Under our standard conditions, no product was formed which was perceived to be due to unfavourable reaction morphology (Scheme 3E). To that end, we carried out a mini-optimization on this specific substrate with values in the table Scheme 3E sourced from 1H NMR analysis of the crude reaction mixture against a relevant standard. From these studies, we found that increasing the effective milling temperature – using our in-house designed and prototyped PID-controlled jar heater21 – to 75 °C and adding sand as a grinding auxiliary (1 mass equivalents), enabled the promising formation of the cyclopropanated cholesterol analogue. After epoxidation of the remaining alkene and separation via silica gel column chromatography, the cyclopropane structure was isolated in 30% yield. Whilst this yield remains modest, it serves to highlight the opportunities mechanochemistry can offer in unlocking materials which perform poorly in solution-phase conditions.
Aware that the starting material for cyclopropane 3l was prepared via a Barbier-type reaction, which has previously seen success in a similar mechanochemical methodology,12 we sought to conduct a one-jar Barbier/Simmons–Smith cyclopropanation protocol, whereby mechanochemical activation of zinc(0) facilitated both transformations (Scheme 4A). Milling cyclohexanone, diiodomethane, and allyl bromide in the presence of zinc(0) (7 eq.), and 2-MeTHF (1.5 eq.) for 1 h led to the installation of the methylcyclopropane group in a promising 44% yield (3l).
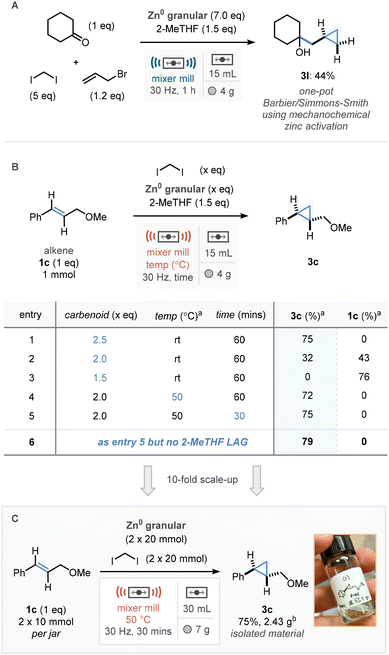 |
| Scheme 4 Tandem organozinc reactions via ball milling and scale up; (A) Tandem mechanochemical Barbier/Simmons-Smith process; (B) Optimization for scale up on model substrate; (C) scale-up of the mechanochemical Simmons–Smith reaction; aNMR yield calculated via 1H NMR analysis of the crude reaction mixture vs. 1,3,5-trimethoxy-benzene as an internal standard. bSum of two reaction jars. | |
Scale-up
Following this the scalability of the Simmons–Smith protocol was investigated. Initially, to maximise the scale in the available jars and to reduce waste, we sought to reduce the quantity of the Simmons–Smith reagent required. Pleasingly, whilst reducing the quantities did lead to appreciable drop in reaction efficiency (Scheme 4B, entries 1–3), supplementary heating to 50 °C reinvigorated reactivity, leading to cyclopropane 1c in 72% yield (entry 4). In addition to this, reducing the reaction time led to slightly increased formation of product (entry 5, 75%). At this point it was also found that after reduction of the amount of Simmons–Smith reagent and with supplementary heat that the 2-MeTHF LAG was no longer required (entry 6). Using these small-scale NMR studies of the crude reaction mixtures, in combination with larger 30 mL jars the reaction was scaled ten-fold to 20 mmol (10 mmol in each jar) in a single mixer mill run, affording 2.43 g of cyclopropanated product in good yield (75%, Scheme 4C). This was all carried out in the absence of reaction solvent, and without the need for any air/moisture-sensitive reaction set-ups.
Sustainability metrics
When studying our reaction protocols from a sustainability perspective, analysing the process mass intensity (PMI) of a given reaction set-up can give information on the waste streams produced using eqn (1) (Fig. 1A).22
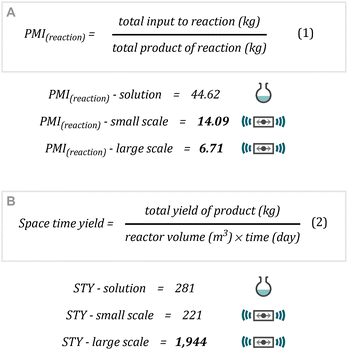 |
| Fig. 1 Metrics calculations for the cyclopropanation methodology.(A) Process Mass Intensity; (B) Space-Time Yield. | |
The PMI of a given reaction is made up of two components PMI(reaction) and PMI(work-up). Within this context comparing un-optimized work-up procedures has been reported to be routinely misused and non-productive.23 On the other hand, when calculating the PMI(reaction) of our systems revealed PMI(reaction) – small scale of 14.09 and PMI(reaction) – large scale of 6.71. These figures are an improvement on an archetypal solution-phase reaction such as in Scheme 2, entry 17 of 44.62, highlighting the possibilities that mechanochemistry can provide for minimization of waste when designing synthetic protocols. It must be noted that the current work-up/purification procedure is non-optimal from a sustainability perspective. Whilst this was not the focus of this report, it must also be considered when moving forward in the development of mechanochemistry protocols.
Further to this, the analysis of space–time yield provides information about process/reactor efficiency and is calculated via eqn (2) (Fig. 1B).24 Whilst on small scale there isn't much appreciable difference between reactor efficiency (comparing a 10 mL round-bottomed flask with a 15 mL milling jar), our large-scale operation using the 30 mL jars enabling the processing of 10 mmol per jar provides an increase in space–time yield to 1944 kg m−3 day−1.
Conclusions
In conclusion, a mechanochemical Simmons–Smith reaction has been designed and developed. Owing to ball-milling-enabled activation of raw form zinc, we are able to access a system to enable the cyclopropanation of a range of akenes including (homo)allylic alcohols, (silyl) enol ethers, and Michael acceptors, as well as terpene and cholesterol structures. We further demonstrated tandem Barbier/Simmons–Smith reactivity in a “one-jar” protocol and through use of a PID-controlled jar heater enabled the solvent-free gram-scale cyclopropanation of an allylic ether in 30 minutes. Work is ongoing to continue exploration and exploitation of mechanochemical zero-valent metal activation.
Author contributions
JAL and DLB conceptualised, designed, and managed the project. LP conducted the experiments. JAL, LP, and DLB prepared the manuscript.
Conflicts of interest
There are no conflicts to declare.
Acknowledgements
The authors thank Università degli Studi di Perugia for a doctoral scholarship and mobility programme grant (L.P.) and the Leverhulme Trust for a Research Project Grant (RPG-2019-260, D.L.B., J.A.L).
References
-
(a) U. Tilstam and H. Weinmann, Org. Process Res. Dev., 2002, 6, 906–910 CrossRef CAS;
(b) A. Hafner, P. Filipponi, L. Piccioni, M. Meisenbach, B. Schenkel, F. Venturoni and J. Sedelmeier, Org. Process Res. Dev., 2016, 20, 1833–1837 CrossRef CAS.
-
(a) T. L. Rathman and J. A. Schwindeman, Org. Process Res. Dev., 2014, 18, 1192–1210 CrossRef CAS;
(b) H. Kryk, G. Hessel, W. Schmitt and N. Tefera, Chem. Eng. Sci., 2007, 62, 5198–5200 CrossRef CAS.
- A. C. Jones, J. A. Leitch, S. E. Raby-Buck and D. L. Browne, Nat. Synth., 2022, 1, 763–775 CrossRef.
-
(a) P. Knochel and R. D. Singer, Chem. Rev., 1993, 93, 2117–2188 CrossRef CAS;
(b) D. Seyferth, Organometallics, 2001, 20, 2940–2955 CrossRef CAS;
(c) S. M. Manolikakes, M. Ellwart, C. I. Stathakis and P. Knochel, Chem. – Eur. J., 2014, 20, 12289–12297 CrossRef CAS PubMed.
- F. P. Byrne, S. Jin, G. Paggiola, T. H. M. Petchey, J. H. Clark, T. J. Farmer, A. J. Hunt, C. R. McElroy and J. Sherwood, Sustainable Chem. Processes, 2016, 4, 7 CrossRef.
-
(a) L. Takacs, Chem. Soc. Rev., 2013, 42, 7649–7659 RSC;
(b) E. Boldyreva, Chem. Soc. Rev., 2013, 42, 7719–7738 RSC;
(c) A. Stolle, T. Szuppa, S. E. S. Leonhardt and B. Ondruschka, Chem. Soc. Rev., 2011, 40, 2317–2329 RSC;
(d) J. L. Howard, Q. Cao and D. L. Browne, Chem. Sci., 2018, 9, 3080–3094 RSC;
(e) J. Andersen and J. Mack, Green Chem., 2018, 20, 1435–1443 RSC;
(f) I. N. Egorov, S. Santra, D. S. Kopchuk, I. S. Kovalev, G. V. Zyranov, A. Majee, B. C. Ranu, V. L. Rusinov and O. N. Chupakhin, Green Chem., 2020, 22, 302–315 RSC;
(g) T. Friščić, C. Mottillo and H. M. Titi, Angew. Chem., Int. Ed., 2020, 59, 1018–1029 CrossRef PubMed;
(h) K. Kubota and H. Ito, Trends Chem., 2020, 2, 1066–1081 CrossRef CAS;
(i) J. A. Leitch and D. L. Browne, Chem. – Eur. J., 2021, 27, 9721–9726 CrossRef CAS PubMed;
(j) J.-L. Do and T. Friščić, ACS Cent. Sci., 2017, 3, 13–19 CrossRef CAS;
(k)
A. Krusenbaum, S. Grätz, G. T. Tigineh, L. Borchardt and J. G. Kim, Chem. Soc. Rev., 2022512873–2905 Search PubMed;
(l) A. Beillard, X. Bantreil, T.-X. Métro, J. Martinez and F. Lamaty, Chem. Rev., 2019, 119, 7529–7609 CrossRef CAS;
(m) O. Bento, F. Luttringer, T. M. E. Dine, N. Pétry, X. Bantreil and F. Lamaty, Eur. J. Org. Chem., 2022, e202101516 CAS;
(n) F. Cuccu, L. De Luca, F. Delogu, E. Colacino, N. Solin, R. Mocci and A. Porcheddu, ChemSusChem, 2022, e202200362 CAS;
(o) A. Porcheddu, E. Colacino, L. De Luca and F. Delogu, ACS Catal., 2020, 10, 8344–8394 CrossRef CAS;
(p) A. A. L. Michalchuk, E. V. Boldyreva, A. M. Belenguer, F. Emmerling and V. V. Boldyrev, Front. Chem., 2021, 9, 685789 CrossRef CAS PubMed;
(q) S. L. James, C. J. Adams, C. Bolm, D. Braga, P. Collier, T. Friščić, F. Grepioni, K. D. M. Harris, G. Hyett, W. Jones, A. Krebs, J. Mack, L. Maini, A. G. Orpen, I. P. Parkin, W. C. Shearouse, J. W. Steed and D. C. Waddell, Chem. Soc. Rev., 2012, 41, 413–447 RSC;
(r) P. Ying, J. Yu and W. Su, Adv. Synth. Catal., 2021, 363, 1246–1271 CrossRef CAS.
-
(a) K. Kubota, R. Takahashi and H. Ito, Chem. Sci., 2019, 10, 5837–5842 RSC;
(b) K. Kubota, R. Takahashi, M. Uesugi and H. Ito, ACS Sustainable Chem. Eng., 2020, 8, 16577–16582 CrossRef CAS.
- A. C. Jones, J. A. Leitch, S. E. Raby-Buck and D. L. Browne, Nat. Synth., 2022, 1, 763–775 CrossRef and references therein.
- For activation of magnesium metal by ball milling see:
(a) J. M. Harrowfield, R. J. Hart and C. R. Whitaker, Aust. J. Chem., 2001, 54, 423–425 CrossRef CAS;
(b) V. Birke, C. Schütt, H. Burmeier and W. K. L. Ruck, Fresenius Environ. Bull., 2011, 20, 1794–2805 Search PubMed;
(c) I. R. Speight and T. P. Hanusa, Molecules, 2020, 25, 570 CrossRef CAS PubMed;
(d) V. S. Pfennig, R. C. Villella, J. Nikodemus and C. Bolm, Angew. Chem., Int. Ed., 2022, 61, e20116514 CrossRef PubMed;
(e) R. Takahashi, A. Hu, P. Gao, Y. Gao, Y. Pang, T. Seo, J. Jiang, S. Maeda, H. Takaya, K. Kubota and H. Ito, Nat. Commun., 2021, 12, 6691 CrossRef CAS PubMed For associated safety warning, see:
(f) T. Vilaivan, ACS Chem. Health Saf., 2022, 29, 132–134 CrossRef CAS.
- Q. Cao, J. L. Howard, E. Wheatley and D. L. Browne, Angew. Chem., Int. Ed., 2018, 57, 11339–11343 CrossRef CAS PubMed.
- Q. Cao, R. T. Stark, I. A. Fallis and D. L. Browne, ChemSusChem, 2019, 12, 2554–2557 CrossRef CAS PubMed.
- J.-X. Yin, R. T. Stark, I. A. Fallis and D. L. Browne, J. Org. Chem., 2020, 85, 2347–2354 CrossRef CAS PubMed.
- For very recent explorations into alternative organometallic nucleophiles such as organocalcium and organomanganese, see:
(a) P. Gao, J. Jiang, S. Maeda, K. Kubota and H. Ito, Angew. Chem., Int. Ed., 2022, e202207118 CAS;
(b) R. Takahashi, P. Gao, K. Kubota and H. Ito, Chem. Sci., 2023, 14, 499–505 RSC.
-
(a) H. E. Simmons and R. D. Smith, J. Am. Chem. Soc., 1959, 81, 4256–4264 CrossRef CAS;
(b) M. Nakamura, A. Hirai and E. Nakamura, J. Am. Chem. Soc., 2003, 125, 2341–2350 CrossRef CAS PubMed;
(c) G. Bartoli, G. Bencivenni and R. Dalpozzo, Synthesis, 2014, 979–1029 Search PubMed;
(d) R. S. Shank and H. Shechter, J. Org. Chem., 1959, 24, 1825–1826 CrossRef CAS;
(e) A. B. Charette and A. Beauchemin, Org. React., 2001, 58, 1–415 CAS.
-
(a) E. J. Corey and M. Chaykovsky, J. Am. Chem. Soc., 1965, 87, 1353–1364 CrossRef CAS;
(b) H. Lebel, J.-F. Marcoux, C. Molinaro and A. B. Charette, Chem. Rev., 2003, 103, 977–1050 CrossRef CAS PubMed;
(c) D. Y.-K. Chen, R. H. Pouwer and J.-A. Richard, Chem. Soc. Rev., 2012, 41, 4631–4642 RSC;
(d) W. Wu, Z. Lim and H. Jiang, Org. Biomol. Chem., 2018, 16, 7315–7329 RSC.
- M. R. Bauer, P. Di Fruscia, S. C. C. Lucas, I. V. Michaelides, J. E. Nelson, R. I. Storer and B. C. Whitehurst, RSC Med. Chem., 2021, 12, 448–471 RSC.
- For a mechanochemical cyclopropanation methodology using diazo compounds, see:
(a) L. Chen, M. O. Bovee, B. E. Lemma, K. S. M. Keithley, S. L. Pilson, M. G. Coleman and J. Mack, Angew. Chem., Int. Ed., 2015, 54, 11084–11087 CrossRef CAS PubMed;
(b) L. Chen, D. Leslie, M. G. Coleman and J. Mack, Chem. Sci., 2018, 9, 4650–4661 RSC.
- This copper-free activation of zinc and subsequent SSR is consistent with the ultrasounds-based protocol reported by Repič and Vogt, see: O. Repič and S. Vogt, Tetrahedron Lett., 1982, 23, 2729–2732 CrossRef.
- Y. Sawama, N. Yasukawa, K. Ban, R. Goto, M. Niikawa, Y. Monguchi, M. Itoh and H. Sajiki, Org. Lett., 2018, 20, 2892–2896 CrossRef CAS PubMed.
- For unsuccessful and low yielding substrates see ESI.†.
- For further details, see: R. R. A. Bolt, S. E. Raby-Buck, K. Ingram, J. A. Leitch and D. L. Browne, Angew. Chem., Int. Ed., 2022, 61, e202210508 CrossRef CAS PubMed.
- D. P. Kjell, I. A. Watson, C. N. Wolfe and J. T. Spilter, Org. Process Res. Dev., 2013, 17, 169–174 CrossRef CAS.
- C. Jimenez-Gonzalez, C. S. Ponder, Q. B. Broxterman and J. B. Manley, Org. Process Res. Dev., 2011, 15, 912–917 CrossRef CAS; E. R. Monteith, P. Mampuys, L. Summerton, J. H. Clark, B. U. W. Maes and C. R. McElroy, Green Chem., 2020, 22, 123–135 RSC.
-
(a) G. J. Hanz and S. C. Wait, J. Chem. Phys., 1955, 23, 1550 Search PubMed;
(b) R. R. A. Bolt, J. A. Leitch, A. C. Jones, W. I. Nicholson and D. L. Browne, Chem. Soc. Rev., 2022, 51, 4243–4260 RSC.
|
This journal is © The Royal Society of Chemistry 2023 |
Click here to see how this site uses Cookies. View our privacy policy here.