DOI:
10.1039/D2GC04548F
(Paper)
Green Chem., 2023,
25, 1442-1452
Sodium ethoxide as an environmentally benign and cost-effective catalyst for chemical depolymerization of post-consumer PET waste†
Received
29th November 2022
, Accepted 16th January 2023
First published on 19th January 2023
Abstract
Polyethylene terephthalate (PET) waste is mounting up in the environment due to its poor biodegradability and low recycling rate. Glycolysis is a promising chemical recycling technique to convert PET into its monomer bis(2-hydroxyethyl)terephthalate (BHET). Here, we present our work on the glycolytic depolymerization of post-consumer PET waste using sodium ethoxide (EtONa) as a low-cost catalyst. In order to optimize the reaction in terms of PET conversion and BHET yield, response surface methodology (RSM) based on the Box–Behnken design was applied for the reaction temperature (160–190 °C), the molar ratio of PET
:
EtONa (50–150), the molar ratio of ethylene glycol to PET (EG
:
PET) (3–7), the reaction time (2–6 h) and the PET particle size (0.25–1 mm). Based on the experimental results, regression models as a function of significant process parameters were obtained and evaluated by analysis of variance (ANOVA) to predict the depolymerization performance of EtONa. By further optimization and expanding the parameter space beyond the initial upper limits, high PET conversion (98%) and an isolated yield of BHET (76%) were achieved. Under similar conditions, its depolymerization performance was compared to other widely studied catalysts, such as zinc acetate (PET conversion 97%, BHET yield 75%) and cobalt acetate (PET conversion 93%, BHET yield 70%). BHET precipitation without water is also demonstrated and it was found that EG and catalyst recycling is possible at least for 5 recycled runs with persistent conversion. Hence, EtONa is a very promising low-cost catalyst for PET depolymerization which has potential feasibility for a large-scale process.
Introduction
Durability and resistance to degradation make plastics versatile in innumerable applications in almost every aspect of daily life. The global production of plastics in 2020 was 367 million tons.1 The largest groups of plastics include polyethylene, polypropylene, polyvinyl chloride, polyethylene terephthalate (PET), polyurethane, and polystyrene. Polyesters, most of which are PET, make up 70% of plastic fiber production.2 The global production of PET was 41.56 million tons in 2014 and reached 73.39 million tons in 2020.3 The best-known applications are within the packaging sector, where global PET packaging consumption grew at an annual average rate of 4.0% to 21.8 million tons during 2015–19 and is forecast to grow at an annual average rate of 3.7% to 27.1 million tons during 2020–25.4 Owing to its light weight, transparency, and virtually indestructible properties, PET has a huge stake in the bottle marketplace that resulted in the production of 583.3 billion PET bottles in 2021.5 This huge production of plastic bottles will result in the generation of billions of disposable drinking bottles as post-consumer waste. The green chemistry framework developed by Anastas and Warner focuses on preventing waste generation for the betterment of humans and the environment.6,7 Furthermore, as PET is a petroleum-derived product and natural resources are finite, the production of PET contributes to the reduction of fossil reserves. Therefore, exploring effective recycling methods for PET waste is a universally urgent demand.8
Tertiary recycling is a chemical recycling process that transforms a polymer backbone into its respective monomers, if needed in the presence of a suitable solvent. Chemical recycling is gaining significance because the quality of the recycled products is identical to that of the virgin products and it can be conducted also on a mechanically recycled polymer that has already passed through several life cycles. Therefore, it has the potential to be the ultimate sustainable method for plastic recycling.9
Depending upon the nature of the solvent employed, the chemical recycling methods of PET waste are divided into glycolysis,10,11 hydrolysis,12,13 and methanolysis.14–16 Glycolysis is the most promising method17 owing to the low cost, comparatively mild operating conditions, low volatility of solvents, and the resulting product bis(2-hydroxyethyl)terephthalate (BHET) that can easily be integrated into the existing standard production lines of PET, mixed with virgin raw materials.
The glycolysis of post-consumer PET waste is carried out in the presence of a transesterification catalyst. In the literature, various catalysts have been reported that include metal acetates (cobalt, lead, zinc, and manganese),18–20 less efficient sodium carbonate and sodium bicarbonate,21 titanium phosphate,22 deep eutectic solvents,23 organic catalysts,24 heterogeneous catalysts,25–28 and ionic liquids of zinc and copper acetate.29 The most active glycolysis catalyst is zinc acetate, but as a heavy metal, zinc is toxic to the environment. Furthermore, the expensive nature of these reported catalysts is a hurdle to implement them in large-scale applications. For these reasons, there is a need for a catalyst that allows the economic processing of large volumes of PET waste with acceptable performance in terms of PET conversion and BHET yield under mild and environmentally benign conditions.
Herein, we describe our study on glycolysis with sodium ethoxide, a basic metal alkoxide, as a low-cost depolymerization catalyst to reduce post-consumer PET waste, which is achieved by chemical recycling. To determine the optimum operating conditions, a statistical approach for response surface methodology was followed to simultaneously study five operating parameters. To ensure maximum utilization of resources, BHET precipitation without water as well as the reusability of EG and catalyst solution have also been demonstrated. To the best of our knowledge, sodium ethoxide has not been reported so far as a catalyst for PET glycolytic depolymerization.
Reaction mechanism
The transesterification mechanism catalyzed by a metal alkoxide is illustrated in Fig. 1.30 In step 1, EG is deprotonated due to the base strength of sodium ethoxide. The deprotonated EG in step 2 then gets added (nucleophilic substitution) to the electron-deficient carbon atom of the carbonyl group of the PET polymer chain. The leaving group of the polymer chain is then eventually protonated in step 3.
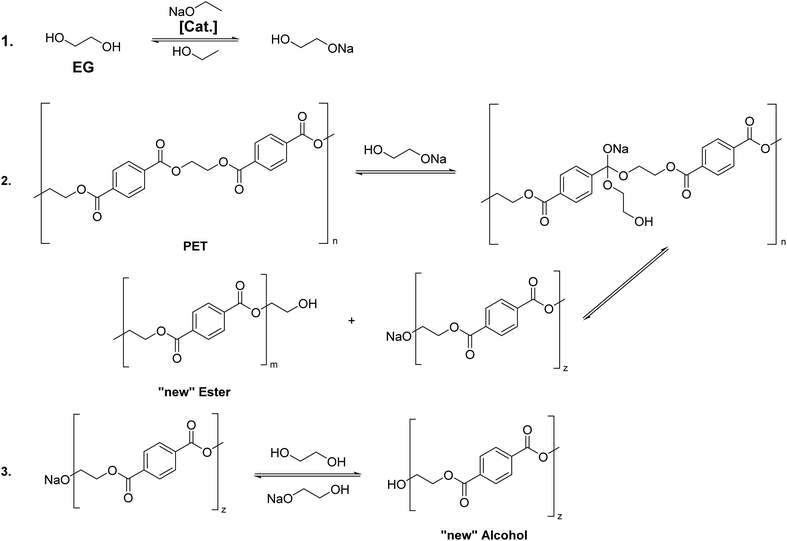 |
| Fig. 1 Schematic reaction mechanism of PET degradation using sodium ethoxide (EtONa) in ethylene glycol n = m + z. | |
Materials and methods
Materials
Ethylene glycol (>99.5%) was purchased from Carl Roth, Germany. Sodium ethoxide (EtONa) was purchased from Sigma Aldrich. Zinc acetate Zn(OAc)2 (>99.9%) was purchased from Alfa Aesar, Germany. Cobalt acetate Co(OAc)2 (>99.9%) was purchased from Sigma Aldrich. HPLC-grade and technical-grade methanol were purchased from VWR Germany. Isopropanol was also purchased from VWR Germany and was used as a rinsing solvent. Deuterated methanol for NMR analysis was purchased from Deutero, Germany. Glass microfiber filters of type GF/C and grade MN GF-1 were purchased from VWR, Germany, and used to filter the PET residue and glycolysis product, respectively. Post-consumer soft drink soda bottles with an estimated number average molecular weight (Mn) of 23
640 g mol−1 (see the ESI† for details) were used as polyethylene terephthalate (PET) sources and they were washed, cleaned, and crushed for use as a substrate in glycolysis reaction, as represented in Fig. 1S (ESI†).
Depolymerization process
To use post-consumer PET bottles as a substrate, the labels and caps were taken off, and the bottles were manually cut into smaller pieces and then washed with water and soap. After drying (overnight, 60 °C), the PET was shredded into small particles using a kitchen grinder. The crushed PET particles were placed into a sieving tower and sieved into fractions of various particle sizes. They were rinsed with isopropanol and stored after drying (overnight, 60 °C). Glycolysis experiments were carried out with a known quantity of PET with the desired particle size that was put into a glass reactor. EG and the catalyst were added in specific ratios. The glass reactor containing PET, EG, and the catalyst was then put onto a heating block with a temperature controller for the desired amount of time under constant stirring. At the end of the reaction time, the heating was stopped and the glycolytic products were filtered with 10 ml of hot (90 °C) water. With this, EtONa will be hydrolyzed and deactivated but this is the standard reported procedure to compare the performance. However, a green approach for extracting BHET without water is also included. (For recycling filtrate residue no water was added.) The reaction mixture was filtered using a microfiber filter type GF/C under vacuum. After this, the reaction mixture was separated into two different fractions, (i) the solid residual fraction was primarily made up of unconverted PET, named A, and (ii) the liquid filtrate fraction consisting mainly of BHET, EG, and water, named B. The residue fraction (A) was kept and dried overnight in an oven at a temperature of 60 °C to determine the conversion with the help of the following formula: |  | (1) |
where mPET,0 is the initial mass of PET in grams and mPET,t is the mass of incompletely depolymerized PET in grams after a certain reaction time t.
The filtrate fraction (B) was stored overnight in a refrigerator at 4 °C to crystallize the product. White crystalline BHET was filtered using the glass microfiber filter grade MN GF-1 under vacuum. The solid crystals, named fraction C, were then dried overnight in an oven at a temperature of 60 °C to determine the BHET yield. As a precaution, the remaining filtrate was stored again in the refrigerator named fraction D. When enough crystals had grown, fraction D was filtered again to extract the remaining crystals to add up to the total BHET yield. If not, the filtrate was discarded. The stored BHET was further analyzed to confirm the product. The BHET yield (isolated) was calculated using the following formula (eqn (2)):
|  | (2) |
where
mPET,0 is the initial weight of PET in grams,
mBHET is the weight of BHET crystals collected in grams,
MBHET is the molecular weight of BHET (254 g mol
−1) and
MPRU is the molecular weight of the
![[P with combining low line]](https://www.rsc.org/images/entities/char_0050_0332.gif)
ET-
![[R with combining low line]](https://www.rsc.org/images/entities/char_0052_0332.gif)
epeating-
![[U with combining low line]](https://www.rsc.org/images/entities/char_0055_0332.gif)
nit (PRU) (192 g mol
−1). The schematic diagram for the depolymerization process is given in
Fig. 2 and the experimental setup is shown in Fig. 2S (ESI
†). The turnover number (TON) and turnover frequency (TOF) of the catalysts were calculated using
eqn (3) and (4), respectively. It should be noted that the TOF was calculated for 20% conversion and is therefore described as the TOF
20.
| 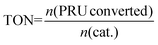 | (3) |
|  | (4) |
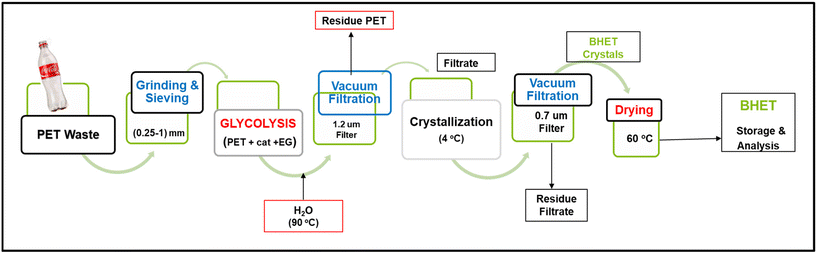 |
| Fig. 2 Schematic diagram of PET glycolysis. | |
Substrate and product characterization
The BHET product was characterized by gas chromatography–mass spectrometry (GC-MS), nuclear magnetic resonance spectroscopy (NMR), differential scanning calorimetry (DSC) and melting point. The glass transition temperature (Tg) was measured through DSC analysis. Afterwards, the Tg was used to determine the approximate Mn of the PET substrate by applying the FOX and FLORY relation. Inductively coupled plasma atomic emission spectroscopy (ICP-EOS) was performed to observe the presence of sodium metals in the filtered residue. The corresponding details and methods are given in the ESI.†
Results and discussion
Influence of parameters
The influence of various operating conditions on EtONa-catalyzed PET glycolysis was investigated in terms of PET conversion and the BHET yield and the results are presented in Fig. 3. As expected, both PET conversion and the BHET yield benefit from a higher reaction temperature (Fig. 3a). The effect of catalyst loading in terms of the substrate (PET) to catalyst (EtONa) ratio was investigated at two different temperatures (Fig. 3b). A ratio of PET
:
EtONa 50 (mol/mol) means there is one mole of catalyst per 50 moles of repeating units of PET waste. It was found that (i) the addition of more catalysts results in higher PET conversion and BHET yields, (ii) the reaction is faster at higher temperatures, and (iii) the effect of the catalyst is obvious at both studied temperatures. The uncatalyzed PET glycolysis experiment was also conducted for reference, where depolymerization was not possible and the PET substrate remained in the mixture without the formation of BHET (see the ESI†). The effect of EG on glycolysis performance was studied by varying the EG
:
PET (mol/mol) ratio (Fig. 3c). Excess EG is required for good catalytic performance since EG acts as a solvent and its higher concentration will shift the equilibrium towards the product side, the monomer. The effect of the reaction time was also studied (Fig. 3d). As anticipated, a longer reaction time led to higher PET conversion and BHET yields.
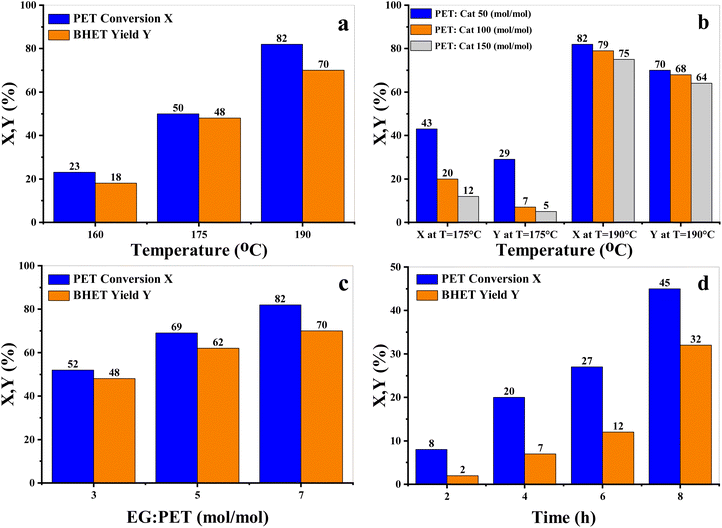 |
| Fig. 3 Influence of operating parameters. Reaction conditions: (a) T = 160–190 °C, PET : Cat = 50 (mol/mol), EG : PET = 7 (mol/mol), time = 6 h, PS = 0.25 mm, rpm = 500. (b) at 175 °C: EG : PET = 5 (mol/mol), time = 4 h, PS = 0.25 mm, rpm = 500, at 190 °C: EG : PET = 7 (mol/mol), time = 6 h, PS = 0.25 mm, rpm = 500. (c) T = 190 °C, PET : Cat = 50 (mol/mol), EG : PET = 3–7 (mol/mol), time = 6 h, rpm = 500, PS = 0.25 mm. (d) T = 175 °C, EG : PET = 5 (mol/mol), PET : Cat = 100 (mol/mol), PS = 0.625 mm, rpm = 500. | |
From the above findings, it can be concluded that (i) a higher operating temperature, higher reaction time, higher EG
:
PET ratio, and lower PET
:
EtONa ratio (higher catalyst loading) enhance glycolysis performance, and (ii) it was also observed that there exist some synergistic effects of parameters on glycolysis performance. Based on these conclusions, a full study of the combined effects of all the operating parameters is desirable to further optimize the catalytic performance of EtONa in the depolymerization of post-consumer PET waste. To accomplish this, an experimental layout was made using the design of experiment methodology using the software “Design Expert”. With this approach, not only can all the parameters be studied together but a predictive mathematical model for glycolysis performance will also be derived.
Design of experiments (DoE)
To study the combined effect of all parameters, the experimental plan was carried out through a response surface methodology (RSM) approach based on the Box–Behnken design (BBD) using the software Design Expert. In comparison with conventional factorial design techniques, BBD can significantly reduce the number of experimental sets without affecting the accuracy of optimization.31,32 For the Box–Behnken design, only three levels are needed for each factor, which is a practical advantage. This fact is frequently significant in industrial experimentation. Another choice is the uniform shell design, which according to Doehlert and Klee typically requires three or five levels for each factor in the experiment (though up to nine levels may be needed).33 But there is a trade-off between design effectiveness and design size, which is why BBD was chosen for this study.34 Five independent variables (reaction temperature, reaction time, PET
:
EtONa (molar ratio), EG
:
PET (molar ratio), and particle size) were investigated with the help of 46 experiments. PET conversion X (%) and BHET yield Y (%) were the observed responses. The coded and uncoded values of independent factors along with their ranges are given in Table 1.
Table 1 Coded and uncoded values of independent variables
Independent variable |
Symbol |
Coded levels |
Actual (Vi) |
Coded (Ci) |
−1 (L) |
0 (M) |
+1 (H) |
Reaction temperature (°C) |
A
|
C
1
|
160 |
175 |
190 |
Reaction time (h) |
B
|
C
2
|
2 |
4 |
6 |
PET : EtONa (mol/mol) |
C
|
C
3
|
50 |
100 |
150 |
EG : PET (mol/mol) |
D
|
C
4
|
3 |
5 |
7 |
Particle size (mm) |
E
|
C
5
|
0.25 |
0.625 |
1 |
The experimental BBD matrix with the observed responses from 46 experimental runs is presented in Table 3S (ESI†) and was further used to find the regression model after inserting the observed responses. Analysis of variance (ANOVA) was performed to find the significant parameters with the help of a probability p-value test. The coefficient of determination (R2), adjusted R2, adequate precision, and test for Lack of Fit were determined to check the correctness of the established model. A second-order model (eqn (5)) was selected to describe the observed responses (PET conversion X and BHET yield Y.):
|  | (5) |
In eqn (5), X is the predicted response for PET conversion, Y is the predicted response for the BHET yield, bo is the intercept term and bi and bii are linear and second-order polynomial coefficients, respectively. Interaction terms are given by bij, whereas xi and xj are the coded independent variables. The relationship between the coded factors (Ci) and the actual values (Vi) is given by the following generalized expression (eqn (6));
|  | (6) |
where Mi is the medium and Hi is the highest level of independent factors.
Development of regression model
Experimental data for PET conversion and the BHET yield were fitted with a quadratic model. The suitability of the models was tested by the “coefficient of determination” (R2) which is a measure of the fitting degree. Good fitting models were obtained for PET conversion and the BHET yield, where the R2 values were greater than 92% in both cases. Table 2 shows the important fit statistics for both models.
Table 2 Statistical parameters for regression models
Response |
PET conversion (X) |
BHET yield (Y) |
R
2 (%) |
92.31 |
92.48 |
Adjusted R2 |
0.8615 |
0.8646 |
Predicted R2 |
0.7108 |
0.7255 |
Adequate precision |
14.78 |
15.31 |
Lack of Fit (p-value) |
0.1668 |
0.3265 |
Significant terms |
A, B, C, A2, C2, D2 |
A, B, C, A2, D2 |
The higher values of R2 and the adjusted R2 show that regression models are significant. ANOVA results for both the models are given in Tables 4S and 5S (ESI†). “Adeq Precision” measures the signal-to-noise ratio. A ratio greater than 4 is desirable.35 For both developed models, the signal-to-noise ratio is greater than 4, which indicates an adequate signal. Therefore, both the models can be used to navigate the design space. If the p-value is lower than 0.0500, it indicates that model and model terms are significant and the values greater than 0.1000 indicate that the terms are not significant (Tables 4S and 5S†). The significant terms for both responses are given in Table 2. The Lack of Fit (p-value) for both the models implies that the Lack of Fit is not significant as the values are greater than 0.0500. Non-significant Lack of Fit is good for model fitting. The expressions for quadratic models in terms of significant terms for PET conversion and the BHET yield are given in eqn (7) and (8), respectively. However, the complete quadratic models including insignificant terms are also given in the ESI (SEq. 2 & SEq. 3†)
|  | (7) |
| 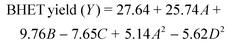 | (8) |
The “Model Summary Statistics” for both responses are also provided in Tables 6S and 7S (ESI†) which give a comparison of all the available models for each response. The selection of the model was based on maximizing the “Adjusted R-Squared” and the “Predicted R-Squared”.
A comparison of predicted versus actual values of both measured responses (PET conversion and BHET yield) is presented in Fig. 4. It can be seen that in most experimental runs, values predicted by both models are very close to the actual experimental values, which is in strong agreement with a higher value of R2 (greater than 92%) in both cases. Therefore, both the models can be used to navigate the design space as they are appropriate to explain the relationship between the independent variables and the observed responses. The maximum PET conversion achieved within the design space using the sodium ethoxide catalyst to depolymerize waste PET was recorded at 79% and the highest BHET yield was recorded at 68%.
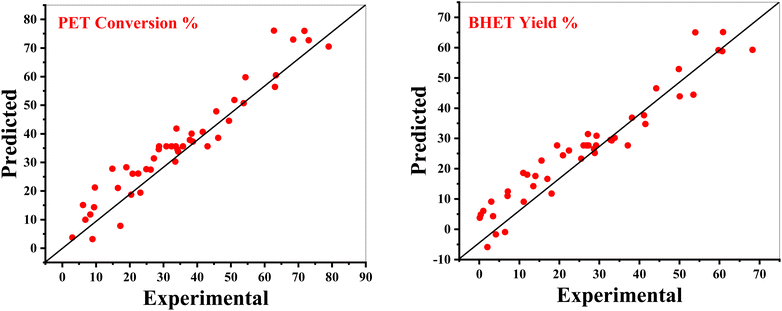 |
| Fig. 4 Predicted vs. actual response for PET conversion (left) and BHET yield (right). | |
Interactive effects
The response surface plot showing the interaction effect of various process parameters on PET conversion is shown in Fig. 9S(a–d) (ESI†). All remaining variables in Fig. 9S† were fixed at the median operating level.
The key observations are (for a more detailed discussion, see the ESI†):
1. Although the increase in the temperature and reaction time is showing a significant increase in PET conversion, the glycolysis temperature has a more pronounced effect. The substantial influence of the operating temperature is also depicted in Table 4S (ESI†) for the ANOVA response for PET conversion, in which the mean square value of the temperature (factor A) is 8 times larger than the reaction time (factor B).
2. Increasing the excess of EG alone is not sufficient to efficiently depolymerize PET. A combined increase in the temperature and amount of EG is needed to achieve a higher depolymerization rate.
3. The effect of catalyst loading is less pronounced than that of the reaction temperature and time. This is evidenced by the mean square value of factor C given in the ANOVA (Table 4S, ESI†), which is the third largest, after operating temperature and reaction time.
4. It is surprising here that the effect of PET particle size is a less significant factor as compared to other operating parameters such as temperature and time as depicted in Table 4S (ESI†). This is because the rate of mass transfer and dissolution of PET is significant for a short reaction time at the early stages of depolymerization when the reaction system is heterogeneous. However, at higher reaction times and higher reaction temperatures, the system tends to be homogeneous, which results in the insignificant impact of particle size as compared to other operating parameters. Furthermore, the results shown in Tables 3 and 4 demonstrate that at a particular reaction temperature and time, the highest conversion is possible with the smallest particle size.
Table 3 Model validation and optimization of EtONa glycolysis
Entry no. |
Reaction conditions |
PET conversion X [%] |
BHET yield Y [%] |
Temp (°C) |
Time (h) |
PET : Cat (mol/mol) |
EG : PET (mol/mol) |
PS (mm) |
Predicted |
Experimental |
Predicted |
Experimental |
1 |
190 |
4.5 |
50 |
5.7 |
0.25 |
79 |
76 |
68 |
67 |
2 |
6 |
103 |
6.5 |
72 |
71 |
62 |
61 |
3 |
5 |
64 |
5.3 |
81 |
77 |
70 |
68 |
Table 4 Performance comparison of EtONa glycolysis with the literature-reported catalysts
Catalyst |
EG : PET : Cat [molar] |
Time [h] |
PS [mm] |
T [°C] |
PET conversion [%] |
BHET yield [%] |
Ref. |
Zn(Oac)2 |
13.75 : 1 : 0.04 |
1.5 |
0.1275 |
197 |
99 |
— |
38
|
15.48 : 1 : 0.01 |
2.5 |
1.00 |
196 |
— |
85.6 |
39
|
13.75 : 1 : 0.04 |
1.5 |
0.1275 |
197 |
97 |
75 |
This work
|
Co(Oac)2 |
13.75 : 1 : 0.04 |
1.5 |
0.1275 |
197 |
99 |
— |
38
|
13.75 : 1 : 0.04 |
1.5 |
0.1275 |
197 |
93 |
70 |
This work
|
Na2CO3 |
7.6 : 1 : 0.01 |
2 |
0.25 |
196 |
— |
66 |
21
|
NaHCO3 |
18.85 : 1 : 0.02 |
3.02 |
— |
192 |
— |
75.7 |
8
|
EtONa |
7.0 : 1 : 0.0156 |
6 |
0.25 |
190 |
82 |
70 |
This work
|
5.3 : 1 : 0.02 |
5 |
0.25 |
190 |
77 |
68 |
13.75 : 1 : 0.04 |
1.5 |
0.1275 |
197 |
98 |
76 |
5. These observations allow us to sort the effects of significant parameters on measured responses in the order of temperature > reaction time > catalyst loading > excess of EG.
The response surface and contour plot of the operating temperature with the reaction time for PET conversion and the BHET yield are given in Fig. 5 with the remaining operating variables fixed at higher levels. From the response surface and contour plot, it is predicted that maximum conversion and yields are attainable at the highest levels of independent variables. For instance, an increase in the temperature will enhance the performance and this prediction was validated when the performance of sodium ethoxide was compared with that of zinc and cobalt acetate (Fig. 6) under the conditions reported in the literature. As experiments were conducted under atmospheric conditions in standard glass equipment, the boiling point of EG (197–198 °C)36 is a limiting factor for the further increase in the temperature.
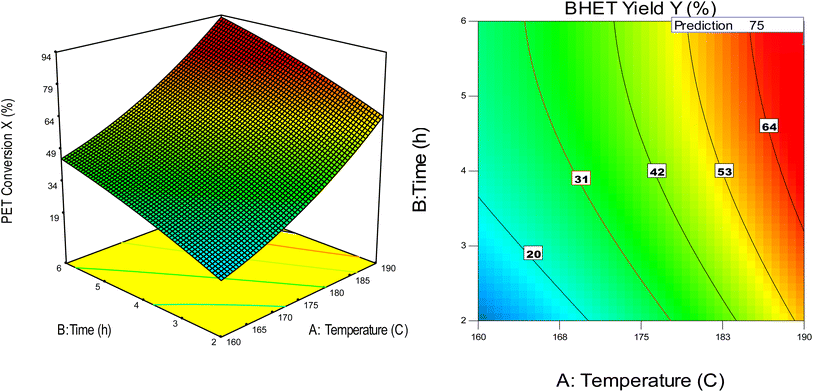 |
| Fig. 5 Response surface and contour plot at higher coded values with temperature vs. time (left) PET conversion (right), BHET yield (PET : Cat = 50 (mol/mol), EG : PET = 7 (mol/mol), PS = 0.25 mm. | |
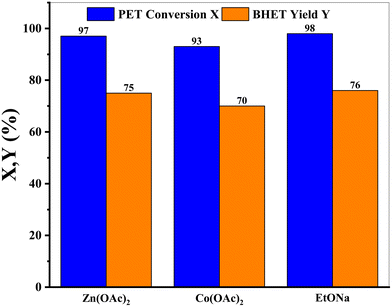 |
| Fig. 6 Performance comparison of EtONa with different catalysts. Reaction conditions: T = 197 °C, time = 1.5 h, PET : Cat = 26 (mol/mol), EG : PET = 13.74 (mol/mol), PS = 0.125 mm, rpm = 500. | |
Model validity and optimization
Based on the obtained experimental results so far (Table 3S†), various experiments were selected by tuning the parameters of the design expert to validate the regression models. For this purpose, the temperature and particle size were fixed at 190 °C and 0.25 mm, respectively. A total of two validation experiments (Table 3, entries 1 and 2) were selected and performed 3 times. An average of the measured responses is given in Table 3. The difference between theoretical and experimental responses was measured in percentage error, which is less than 5% in both validation experiments. This means that both models are suitable for predicting the responses as supported by the high values for the coefficient of determination (R2).
The next step is the optimization of all the independent parameters to achieve the maximum PET conversion and BHET yield. All the input variables were taken in the desirable range to maximize both outputs.37 The optimization experiment (Table 3, entry 3) was also performed 3 times and an average of the measured responses is given. As a result of optimization, the regression model predicted 81% PET conversion and 70% BHET yield (isolated) at 190 °C, a reaction time of 5 hours, PET
:
EtONa = 64, EG
:
PET = 5.3, and a particle size of 0.25 mm.
The experimental average of PET conversion (77%) and BHET yield (68%) were found with a slight deviation from the predicted responses. Hence, the regression model is adequate in predicting the response in the investigated design space.
Performance comparison for sodium ethoxide
The glycolysis performance of sodium ethoxide was compared to the widely studied performance of zinc acetate and cobalt acetate at 197 °C, as reported in the literature,38,39 which is also beyond the initial upper limits of the present study. From Fig. 6, it is noticeable that EtONa has comparable depolymerization efficiency (PET conversion of 98% and BHET isolated yield of 76%) as compared to zinc acetate (PET conversion 97% and BHET isolated yield 75%) and cobalt acetate (PET conversion 93% and BHET isolated yield 70%).
As discussed earlier, the effect of the operating temperature is the highest among all the operating parameters, and it is also reflected when the operating temperature increases from 190 °C to 197 °C and PET conversion increases to 98%. It is significant that under the specified operating conditions, sodium ethoxide can completely depolymerize post-consumer PET waste.
Table 4 gives the data for the comparison of EtONa with various literature-reported catalysts. From those data, it is evident that EtONa compares very well and provides excellent glycolysis performance. The fact that the reaction time can likely be further reduced at higher temperatures and catalyst loading will be very favorable when a scaleup of this depolymerization process is considered. The isolated BHET yields are lower than the PET conversion in all cases owing to the material loss in the course of the filtration/extraction procedure.
The TON is slightly higher for sodium ethoxide (42) as compared to those for zinc acetate (40) and cobalt acetate (40), indicating the better utilization of sodium ethoxide for one glycolysis cycle under the given conditions (Table 5). However, the TOF20 is higher for zinc acetate (9 times) and cobalt acetate (1.5 times) as compared to sodium ethoxide, signifying their superior intrinsic activity. On the other hand, sodium ethoxide is comparatively inexpensive (Table 5) and more environmentally benign as compared to zinc acetate and cobalt acetate, which certainly makes sodium ethoxide a more feasible option for a large-scale PET depolymerization process.
Table 5 Performance comparison based on the TON and TOF
Catalyst |
PET conversion [%] |
TON [mol/mol] |
TOF20a [h−1] |
Cost |
Reaction conditions: T = 190 °C, time = 0.5–1.5 h, PET : Cat = 50 (mol/mol), EG : PET = 7 (mol/mol), PS = 0.25 mm. TOF calculated for 20% PET conversion. |
Zn(OAc)2 |
86 |
40 |
314 |
$25 per kg (ref. 40) |
$2640 per t (ref. 41) |
Co(OAc)2 |
82 |
40 |
54 |
$20 per kg (ref. 42) |
EtONa |
76 |
42 |
36 |
$10 per kg (ref. 43) |
Green glycolysis
Most of the literature reported on PET glycolysis (homogeneously catalyzed) indicates the use of water as a precipitating agent to separate BHET (the main product of depolymerization via glycolysis). Water, on the one hand, serves as a precipitating agent (anti-solvent), while it also dilutes the glycolysed mixture, which makes it easier to let the reaction mixture flow out of the reaction vessel. However, this added water needs to be evaporated in later steps. Furthermore, water destroys sodium ethoxide, which is the glycolysis catalyst. From an industrial point of view, if no water is added to EG, this solvent can be reused for depolymerization and the catalyst will also be saved from water destruction, which leads to the maximum utility of all resources.44 This will make the process more economical and environmentally friendly. It is anticipated that the precipitation of BHET directly from the EG solution will be achieved without adding water.
Therefore, an attempt was made to precipitate BHET without antisolvent (green glycolysis) at the expense of a high EG amount as shown in Fig. 7. Excess EG not only promotes the depolymerization process, on the other hand, it also makes the filtration of the glycolysis reaction mixture more convenient. ICP measurements indicated (Table 2S†) the presence of the sodium metal in the filtrate containing EG. To validate the catalytic activity, the main glycolysis filtrate was recycled (without EG and catalyst makeup) and it was found that PET conversion is almost similar to the main reaction (Fig. 7). The upscaling and recycling of green glycolysis were also performed by increasing the PET substrate 2 times (2 g of PET) and the results are shown in Fig. 8. It was found that PET conversion remains similar up to 5 glycolysis runs, justifying the use of a high EG volume, i.e. 55 g of EG for 10 g of PET recycling in a total of 5 glycolysis runs. Furthermore, the isolated BHET yield is more than 85% for all glycolysis cycles and some crystal loss occurs in the course of the filteration/extraction procedure. To summarize, BHET precipitation without water is possible; however, the optimization of parameters (such as high EG loading) for green glycolysis still needs to be performed and research work related to this is in progress.
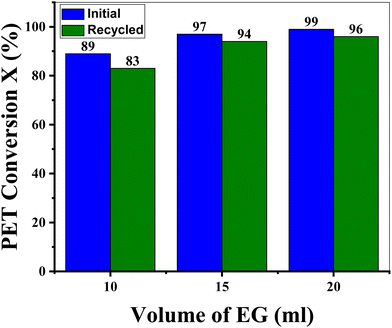 |
| Fig. 7 Effect of the EG volume on PET conversion. Reaction conditions: T = 190 °C, time = 4 h, PET : Cat = 50 (mol/mol), PS = 0.25 mm, PET = 1 g, EG = 11–22 g. | |
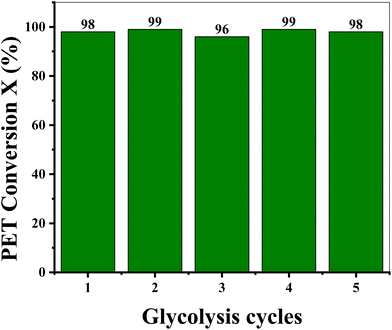 |
| Fig. 8 Recycling of EG and the catalyst. T = 190 °C, time = 4 h, PET : Cat = 50 (mol/mol), EG = 50 ml, EG : PET = 86(mol/mol), PS = 0.25 mm, PET = 2 g, EG = 55 g. | |
Conclusions
PET can be depolymerized into BHET in the presence of sodium ethoxide, utilizing ethylene glycol as a reagent and solvent. A single-factor study of operating parameters shows that PET conversion and BHET yields are dependent on various factors, including the reaction temperature and time, the substrate-to-catalyst ratio, the solvent-to-substrate ratio, and the size of PET particles. Therefore, response surface methodology, based on the Box–Behnken model, was used to study these five independent factors. Analysis of variance was performed and based on fit statistics, and the developed regression models are adequate to predict the PET conversion and BHET yield. The regression models and the interactive study of parameters reveal that the order of significance of the parameters is as follows: reaction temperature > reaction time > PET
:
EtONa > EG
:
PET. It was also found that excess ethylene glycol greatly enhances depolymerization performance. The optimum conditions were found to be 190 °C, 5 h, PET
:
EtONa = 64 (mol/mol), EG
:
PET = 5.28 mol/mol, and particle size 0.25 mm. Confirmation experiments were performed and it was found that under the optimum conditions, PET conversion is 77% and BHET yield is 68%, with a difference of less than 5% from the predicted values. The catalytic depolymerization efficiency of sodium ethoxide was also compared to that of zinc and cobalt acetate. All compared catalysts gave virtually complete conversion and a high yield of BHET. The huge advantage of sodium ethoxide is that it is more environmentally benign than the heavy metal catalysts. Recycling of filtrate residue is feasible for at least five recycle runs with a stable conversion of up to 98%. This certainly makes it a very interesting candidate for a large-scale PET depolymerization process.
Abbreviations
BHET | Bis(2-hydroxyethyl)terephthalate |
Co(OAc)2 | Cobalt acetate (anhydrous) |
DoE | Design of experiments |
EG | Ethylene glycol |
GCMS | Gas chromatography–mass spectroscopy |
MW | Molecular weight |
NMR | Nuclear magnetic resonance |
EtONa | Sodium ethoxide |
PET | Polyethylene terephthalate |
RSM | Response surface methodology |
TON | Turnover number |
TOF | Turnover frequency |
Zn(OAc)2 | Zinc acetate (anhydrous) |
Conflicts of interest
There are no conflicts to declare.
Acknowledgements
Saqib Javed would like to acknowledge the financial assistance from the Higher Education Commission, Pakistan, and Deutscher Akademischer Austauschdienst (DAAD), Germany.
References
-
Plastics Europe, Plastics – the Facts 2021·Plastics Europe. Available at: https://plasticseurope.org/knowledge-hub/plastics-the-facts-2021/, 2022 [accessed 10.02.2022].
- J. Geyer, Law. Production, use, and fate of all plastics ever made, Sci. Adv., 2017, 3(7), e1700782 CrossRef PubMed
.
-
Statista, PET global production 2020 | Statista. Available at: https://www.statista.com/statistics/650191/global-polyethylene-terephthalate-production-outlook/, 2021 [accessed 29.07.2021].
-
Smithers, The Future of Pet Packaging to 2025 | Market Reports and Data | Smithers. Available at: https://www.smithers.com/services/market-reports/packaging/the-future-of-pet-packaging-to-2025, 2021 [accessed 29.07.2021].
-
Statista, PET global bottle production 2021 | Statista. Available at: https://www.statista.com/statistics/723191/production-of-polyethylene-terephthalate-bottles-worldwide/, 2021 [accessed 29.07.2021].
-
American Chemical Society, 12 Principles of Green Chemistry – American Chemical Society. Available at: https://www.acs.org/content/acs/en/greenchemistry/principles/12-principles-of-green-chemistry.html, 2022, 2022.000Z [accessed 02.11.2022.617Z].
- A. Ivanković, Review of 12 principles of green chemistry in practice, Int. J. Sustainable Green Energy, 2017, 6(3), 39 CrossRef
.
- D.-T. Van-Pham, Q.-H. Le, T.-N. Lam, C.-N. Nguyen and W. Sakai, Four-factor optimization for PET glycolysis with consideration of the effect of sodium bicarbonate catalyst using response surface methodology, Polym. Degrad. Stab., 2020, 179(1), 109257 CrossRef CAS
.
- A. B. Raheem, Z. Z. Noor, A. Hassan, M. K. A. Hamid, S. A. Samsudin and A. H. Sabeen, Current developments in chemical recycling of post-consumer polyethylene terephthalate wastes for new materials production: A review, J. Cleaner Prod., 2019, 225, 1052–1064 CrossRef CAS
.
- A. M. Al-Sabagh, F. Z. Yehia, G. Eshaq, A. M. Rabie and A. E. ElMetwally, Greener routes for recycling of polyethylene terephthalate, Egypt. J. Pet., 2016, 25(1), 53–64 CrossRef
.
- A. M. Al-Sabagh, F. Z. Yehia, A.-M. M. F. Eissa, M. E. Moustafa, G. Eshaq and A.-R. M. Rabie,
et al., Glycolysis of poly(ethylene terephthalate) catalyzed by the Lewis base ionic liquid [Bmim][OAc], Ind. Eng. Chem. Res., 2014, 53(48), 18443–18451 CrossRef CAS
.
- G. P. Karayannidis, A. P. Chatziavgoustis and D. S. Achilias, Poly(ethylene terephthalate) recycling and recovery of pure terephthalic acid by alkaline hydrolysis: 12, Adv. Polym. Technol., 2002, 21(4), 250–259 CrossRef CAS
.
- V. Sinha, M. R. Patel and J. V. Patel, PET waste management by chemical recycling: A review, J. Polym. Environ., 2010, 18(1), 8–25 CrossRef CAS
.
- H. Kurokawa, M.-a. Ohshima, K. Sugiyama and H. Miura, Methanolysis of polyethylene terephthalate (PET) in the presence of aluminium tiisopropoxide catalyst to form dimethyl terephthalate and ethylene glycol, Polym. Degrad. Stab., 2003, 79(3), 529–533 CrossRef CAS
.
- M. Goto,
et al., Depolymerization of polyethylene terephthalate in supercritical methanol, J. Phys.: Condens. Matter, 2002, 11427–11430 CrossRef CAS
.
- M. Genta, T. Iwaya, M. Sasaki, M. Goto and T. Hirose, Depolymerization mechanism of poly(ethylene terephthalate) in supercritical methanol, Ind. Eng. Chem. Res., 2005, 44(11), 3894–3900 CrossRef CAS
.
- A. Aguado, L. Martínez, L. Becerra, M. Arieta-araunabeña, S. Arnaiz and A. Asueta,
et al., Chemical depolymerisation of PET complex waste: Hydrolysis vs. glycolysis, J. Mater. Cycles Waste Manage., 2014, 16(2), 201–210 CrossRef CAS
.
- C.-H. Chen, C.-Y. Chen, Y.-W. Lo, C.-F. Mao and W.-T. Liao, Studies of glycolysis of poly(ethylene terephthalate) recycled from postconsumer soft-drink bottles. I. Influences of glycolysis conditions, J. Appl. Polym. Sci., 2000, 80, 943–948 CrossRef
.
- S. R. Shukla and K. S. Kulkarni, Depolymerization of poly(ethylene terephthalate) waste, J. Appl. Polym. Sci., 2002, 85, 1765–1770 CrossRef CAS
.
- M. Ghaemy and K. Mossaddegh, Depolymerisation of poly(ethylene terephthalate) fibre wastes using ethylene glycol, Polym. Degrad. Stab., 2005, 90(3), 570–576 CrossRef CAS
.
- R. López-Fonseca, I. Duque-Ingunza, B. de Rivas, S. Arnaiz and J. I. Gutiérrez-Ortiz, Chemical recycling of post-consumer PET wastes by glycolysis in the presence of metal salts, Polym. Degrad. Stab., 2010, 95(6), 1022–1028 CrossRef
.
- K. Troev, G. Grancharov, R. Tsevi and I. Gitsov, A novel catalyst for the glycolysis of poly(ethylene terephthalate), J. Appl. Polym. Sci., 2003, 90, 1148–1152 CAS
.
- E. Sert, E. Yılmaz and F. S. Atalay, Chemical recycling of polyethlylene terephthalate by glycolysis using deep eutectic solvents, J. Polym. Environ., 2019, 27(12), 2956–2962 CrossRef CAS
.
- Z. Fehér, J. Kiss, P. Kisszékelyi, J. Molnár, P. Huszthy and L. Kárpáti,
et al., Optimisation of PET glycolysis by applying recyclable heterogeneous organocatalysts, Green Chem., 2022, 3, e1700782 Search PubMed
.
- R.-X. Yang, Y.-T. Bieh, C. H. Chen, C.-Y. Hsu, Y. Kato and H. Yamamoto,
et al., Heterogeneous metal azolate framework-6 (MAF-6) catalysts with high zinc density for enhanced polyethylene terephthalate (PET) conversion, ACS Sustainable Chem. Eng., 2021, 9(19), 6541–6550 CrossRef CAS
.
- Y. Kim, M. Kim, J. Hwang, E. Im and G. D. Moon, Optimizing PET glycolysis with an oyster shell-derived catalyst using response surface methodology, Polymers, 2022, 14, 656 CrossRef CAS PubMed
.
- L.-X. Yun, H. Wu, Z.-G. Shen, J.-W. Fu and J.-X. Wang, Ultrasmall CeO2 nanoparticles with rich oxygen defects as novel catalysts for efficient glycolysis of polyethylene terephthalate, ACS Sustainable Chem. Eng., 2022, 10(16), 5278–5287 CrossRef CAS
.
- Q. Suo, J. Zi, Z. Bai and S. Qi, The glycolysis of poly(ethylene terephthalate) promoted by metal organic framework (MOF) catalysts, Catal. Lett., 2017, 147(1), 240–252 CrossRef CAS
.
- A. M. Al-Sabagh, F. Z. Yehia, A. M. F. Eissa, M. E. Moustafa, G. Eshaq and A. M. Rabie,
et al., Cu- and Zn-acetate-containing ionic liquids as catalysts for the glycolysis of poly(ethylene terephthalate), Polym. Degrad. Stab., 2014, 110, 364–377 CrossRef CAS
.
- N. A. M. Aziz, H. A. Hamid, R. Yunus, Z. Abbas, R. Omar and U. Rashid,
et al., Kinetics and thermodynamics of synthesis of palm oil-based trimethylolpropane triester using microwave irradiation, J. Saudi Chem. Soc., 2020, 24(8), 552–566 CrossRef CAS
.
- S. Javed and I. H. Aljundi, Tuning the RO membranes using the spin-assisted layer by layer assembly of polyelectrolytes, Desalin. Water Treat., 2021, 209, 24–36 CrossRef CAS
.
- P. Qiu, M. Cui, K. Kang, B. Park, Y. Son and E. Khim,
et al. Application of Box–Behnken design with response surface methodology for modeling and optimizing ultrasonic oxidation of arsenite with H2O2, Open Chem., 2014, 12(2), 164–172 CrossRef
.
- D. H. Doehlert and V. L. Klee, Experimental designs through level reduction of the d-dimensional cuboctahedron, Discrete Math., 1972, 2(4), 309–334 CrossRef
.
- J. M. Lucas, Which response surface design is best: A performance comparison of several types of quadratic response surface designs in symmetric regions, Technometrics, 1976, 18(4), 411 CrossRef
.
- G. Singh, N. Ahuja, P. Sharma and N. Capalash, Response surface methodology for the optimized production of an alkalophilic laccase from gamma-proteobacterium JB, BioResources, 2009, 4(2), 544–553 CAS
.
-
ChemicalBook, Ethylene glycol | 107-21-1. Available at: https://www.chemicalbook.com/ChemicalProductProperty_EN_CB7852707.htm, 2022, 2022.000Z [accessed 30.10.2022.932Z].
- A. Aguirre-Fierro, H. A. Ruiz, M. A. Cerqueira, R. Ramos-González, R. M. Rodríguez-Jasso and S. Marques,
et al., Sustainable approach of high-pressure agave bagasse pretreatment for ethanol production, Renewable Energy, 2020, 155(1), 1347–1354 CrossRef CAS
.
- A. S. Goje and S. Mishra, Chemical kinetics, simulation, and thermodynamics of glycolytic depolymerization of poly(ethylene terephthalate) waste with catalyst optimization for recycling of value added monomeric products: 29, Macromol. Mater. Eng., 2003, 288, 326–336 CrossRef CAS
.
- G. Xi, M. Lu and C. Sun, Study on depolymerization of waste polyethylene terephthalate into monomer of bis(2-hydroxyethyl terephthalate), Polym. Degrad. Stab., 2005, 87(1), 117–120 CrossRef CAS
.
- Zinc acetate – Chemical Information Search. Available at: https://www.chemicalbook.com/ProductList_En.aspx?kwd=zinc%20acetate, 2022 [accessed 10.08.2022].
- Zinc Acetate-Zinc Acetate Manufacturers, Suppliers and Exporters on Alibaba.comOrganic Salt. Available at: https://www.alibaba.com/trade/search?fsb=y&IndexArea=product_en&CatId=&tab=all&SearchText=zinc+acetate, 2022 [accessed 10.08.2022].
- Cobalt acetate – Chemical Information Search. Available at: https://www.chemicalbook.com/ProductList_En.aspx?kwd=cobalt%20acetate, 2022 [accessed 10.08.2022].
- Sodium ethoxide – Chemical Information Search. Available at: https://www.chemicalbook.com/ProductList_En.aspx?kwd=Sodium%20ethoxide&gclid=Cj0KCQjwrs2XBhDjARIsAHVymmStoPtStoA9HQ0i-tDw_cM5KWxYQivzaUjqkF88C0kJwcoQc7Z0FpoaAmHIEALw_wcB, 2022 [accessed 10.08.2022].
- A. D. Kreuder, T. House-Knight, J. Whitford, E. Ponnusamy, P. Miller, N. Jesse, R. Rodenborn, S. Sayag, M. Gebel, I. Aped, I. Sharfstein, E. Manaster, I. Ergaz, A. Harris and L. N. Grice, A method for assessing greener alternatives between chemical products following the 12 principles of green chemistry, ACS Sustainable Chem. Eng., 2017, 5(4), 2927–2935 CrossRef
.
|
This journal is © The Royal Society of Chemistry 2023 |