DOI:
10.1039/D2GC03534K
(Paper)
Green Chem., 2023,
25, 431-438
Endowing cells with unnatural photocatalytic ability for sustainable chemicals production by bionic minerals-triggering†
Received
20th September 2022
, Accepted 24th November 2022
First published on 25th November 2022
Abstract
Photobiological chemical production is of great importance for solar energy storage and clean fuel generation. One rising strategy for solar fuel production is to enhance the original catalytic efficiency of non-photosynthetic cells by photosensitization of light-harvesting semiconducting nanomaterials. However, endowing cells with a new photocatalytic property to meet the ever-growing demands for environmentally-friendly processes to synthesize multifarious desirable chemicals is still a huge challenge. Herein, we report that a fungus, Saccharomyces cerevisiae, with neither photosynthetic properties nor hydrogen production abilities is endowed with efficient and sustainable light-driven hydrogen production (1063.51 μmol g−1CdS h−1) via the synergistic action of in situ biosynthesized intracellular CdS nanodots (nCdS) and ethanol dehydrogenases (ADH) from yeast. The catalytic mechanism indicates that the photoelectrons from nCdS were transferred to the activated water molecules located in the ADH combined with nCdS, realizing high-efficiency photocatalytic hydrogen production. This finding provides prospective guidance for endowing cells with sustainable and efficient conversion of solar energy by inducing in situ biosynthesized bionic minerals.
Introduction
Although traditional semiconductor photocatalysts have achieved significant breakthroughs and shown efficient energy harvesting and high conversion efficiency, the harsh synthetic conditions and increasing consumption of energy and resources from unsustainable fuels in the chemical synthetic processes of the semiconductor materials remain a great concern.1 Based on the principles and metrics of sustainable development, whole-cell biohybrid systems as bio-catalysts for sustainable solar-to-chemicals production are being developed in a green and eco-friendly way.2–6 Notably, whole-cell photocatalytic biohybrid systems with self-replicating and self-repairing properties exhibit high performance and operational stability under the protection of a cell environment.7–11 For example, whole-cell-based photosynthetic biohybrid systems have been constructed by combining nitrogenases or hydrogenases in cells with implanted quantum dots (InP/ZnSe, CdS, CdSe, etc.) to enhance solar-to-chemicals production.12,13 In addition, a CdS nanophotosensitizer precipitated on the surface of S. oneidensis MR-1 can also promote the solar-to-hydrogen production of hydrogenases inside bacteria.14
Despite the whole-cell biohybrid systems showing impressive photocatalytic activities for the sustainable production of chemicals, most studies focus on known non-photosynthetic microorganisms but with the original biological and chemical production functions.15–21 If a cell with neither nonphotosynthetic properties nor inherent chemical bioproduction ability can be endowed with a new capacity for sustainable photobiological chemical production, a substantial number of microorganisms can move beyond the limits of natural evolution to meet chemists’ extensive needs for sustainable chemical production.
In recent years, some efforts have been made to explore the specific properties of nanomaterials in regulating the original biological activity of enzymes to discover their different catalytic functions from those of native ones.22–27 Therefore, we wondered whether a cell could be endowed with a new catalytic function under the mediation of intracellular bionic semiconducting nanoparticles. Here, bionic nanominerals can not only capture light and produce photoelectrons, but can also induce enzymes to catalyse new reactions. The green and in situ biomimetic synthetic strategy for the modification of the living cell can eliminate the use of harsh conditions (high temperature, high pressure and hazardous solvent) or the generation of adverse substances in the design and application of chemical products. Notably, this attempt is undoubtedly helpful in extending next-generation natural whole-cell photocatalysts for sustainable solar-to-chemical production.
Herein, we chose Saccharomyces cerevisiae with neither original hydrogen production abilities nor photosynthetic properties as a cell model,28,29 and CdS with an appropriate bandgap as a photosensitizer. A biohybrid system consisting of Saccharomyces cerevisiae and in situ biosynthesized intracellular CdS nanodots (nCdS@Yeasts) was developed for sustainable solar-to-hydrogen production. Saccharomyces cerevisiae contains abundant dehydrogenases (ADHs) with the natural function of selectively catalysing the transformation of ethanol to acetaldehyde.30,31 The active-site zinc ion in ADH is bound in a distorted tetrahedral coordination by the sulfhydryl groups of Cys-43, Cys-153 and the imidazole of His-66, with the fourth coordination position being accessible to water and other ligands from solution.32,33 Universally, H2O as the fourth ligand is a critical component of the catalytically active zinc sites, and the O–H could be activated by polarization.34,35 Therefore, these could open up the possibility of designing intracellular bionic photosensitizers inducing ADH-catalyzed H2O-reducing reactions for hydrogen production inside yeast. This green and in situ synthetic strategy has broken through the bottleneck caused by photosensitization of nonphotosynthetic bacteria currently using foreign light-harvesting semiconducting nanomaterials only to enhance their original catalytic efficiency. More generally, a similar material-based in situ modification strategy offers a tenable method for diverse products and may be extended to other microorganisms to induce a designed functional transformation.
Results and discussion
Formation of nCdS@Yeasts biohybrid systems
The hybrid systems are synthesized in situ via facile incubation with the exogenous Cd2+ and S2− in turn, and exhibit efficient hydrogen production (1063.51 μmol g−1CdS h−1) under visible light compared to the case of native Saccharomyces cerevisiae without hydrogen production ability and nCdS synthesized in vivo (Scheme 1). We attributed the H2 production of the hybrid systems to integration between the nCdS and the fungus. Mechanistic studies indicate that the ADHs derived from the yeasts can combine with nCdS and contribute to photocatalytic hydrogen production. Density functional theory (DFT) further indicates that the fracturing of O–H in H2O activated by ADH needs lower energy, which is beneficial for activated H2O accepting photoelectrons from nCdS to produce H2. Therefore, the work reveals intracellular bionic minerals triggering enzyme-catalyzed reactions to endow a nonphotocatalytic microorganism with different biocatalytic functions from native ones.
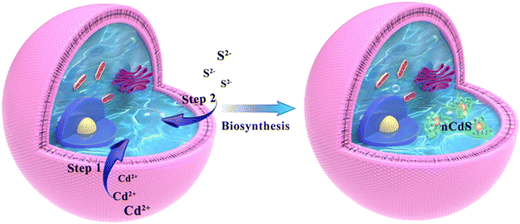 |
| Scheme 1 The formation mechanism of nCdS@Yeasts. | |
Characterization of the nCdS@Yeasts biohybrid systems
Fig. 1 reveals that the biosynthesized nCdS are present inside the cell rather than on its surface. The scanning electron micrograph (SEM) image of the nCdS@Yeasts shows that the surfaces of nCdS@Yeasts are as smooth as the native cells (Fig. 1a and Fig. S1†), preliminarily indicating that there are no nCdS on the surface of the yeasts. Furthermore, it can be clearly observed that the nCdS are well dispersed in the cytoplasm of yeasts from the transmission electron microscope (TEM) images of fine slices of nCdS@Yeasts (Fig. 1b). The confocal laser scanning microscopy (CLSM) images of the nCdS@Yeasts show that there is obvious green emission from the nCdS inside the hybrid system (Fig. 1c), and their 3D visualization further confirms the distribution of nCdS (Fig. 1d). In comparison, no fluorescence signals can be observed in the native yeasts (Fig. S2†). High-resolution TEM (HRTEM) analysis indicates the isolated CdS nanodots from the nCdS@Yeasts have a uniform diameter of about 6.01 nm (Fig. 1e and f) and the lattice spacing (ca. d = 1.76 Å) of individual intracellular nanodots agrees with the distance facets (311) of CdS (inset in Fig. 1e). A typical Tyndall effect and the high value of the zeta potential (about −20.1 mV) of the isolated nCdS aqueous solution in pH 7.5 further indicate their good dispersibility and small size, probably resulting from the regulation of intracellular proteins (inset of Fig. 1f and Fig. S3†).36,37 The amount of nCdS in nCdS@Yeasts was determined to be 7.9% by inductively coupled plasma mass spectrometry (ICP-MS). The crystal structure and composition of the nanodots were determined from the X-ray diffraction (XRD) patterns and X-ray photoelectron spectroscopy (XPS) of the isolated nCdS from nCdS@Yeasts. The XRD result indicates that broad diffraction peaks around 27.4°, 43.9° and 52.6° could be indexed to the (111), (220) and (311) reflections for cubic CdS (JCPDS card 65-2887), respectively (Fig. 1g). Moreover, the XPS analyses also show the typical values for isolated nCdS, including the abundant carbon, oxygen and nitrogen derived from the intracellular proteins (Fig. S4†).38,39 These characterizations clearly confirm that nCdS, with excellent uniformity and stability, are biosynthesized in situ inside the cells under the regulation of intracellular proteins.
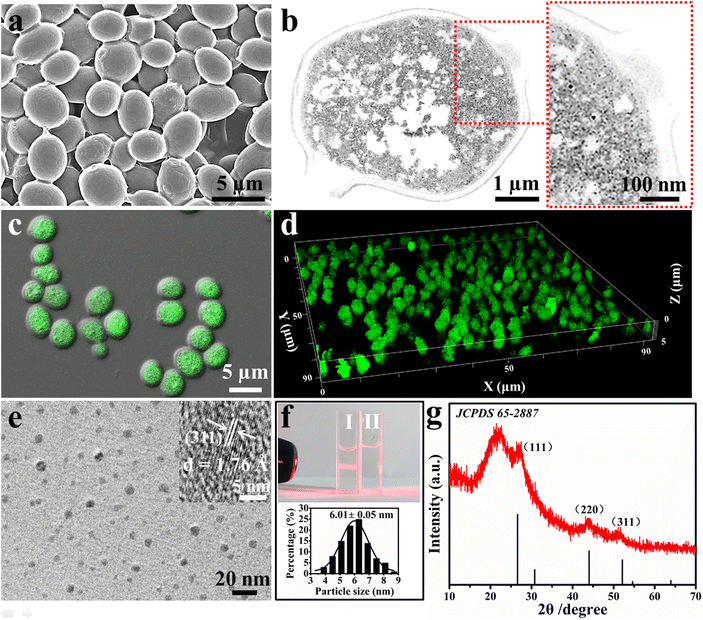 |
| Fig. 1 The characterizations of nCdS@Yeasts biohybrid systems with intracellular nCdS. (a) SEM image of nCdS@Yeasts. (b) TEM image of fine slices of nCdS@Yeasts and the corresponding magnified image of the outlined red rectangle. (c and d) CLSM image and 3D visualization of nCdS@Yeasts under 405 nm laser excitation. (e) HRTEM image of nCdS separated from nCdS@Yeasts (the insert shows the lattice spacing of the nCdS). (f) Photograph of nCdS in aqueous solution (I) and the aqueous solution (II). The nCdS micelle shows a Tyndall effect. The following image shows the nCdS size distribution. (g) XRD spectrum of the isolated nCdS from nCdS@Yeasts. | |
The effect of intracellular nCdS on bioactivity
To explore the effect of intracellular nCdS on the biological properties of the microorganism, we tested the integrity and reproductivity of nCdS@Yeasts biohybrid systems. The trypan blue exclusion assay shows that the membranes of nCdS@Yeasts are still intact (Fig. S5†). Fig. 2a and b are the images of a live/dead assay for native yeast cells and nCdS@Yeasts, respectively. Based on the green color of living cells in the two images, this indicates that the nCdS@Yeasts biohybrid systems are alive, similar to native cells. The optical density measurements of the nCdS@Yeasts (OD600) in the media show that the proliferation of hybrid systems is initially in a dormant state before entering a rapid cell proliferation state up to 20 h of incubation (Fig. 2c). The above results demonstrate that the synthetic process is mild, and the formation of intracellular nanodots does not prevent cells from multiplying, which could benefit the sustained use of the catalysts.
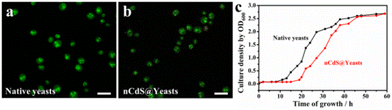 |
| Fig. 2 The bioactivity of nCdS@Yeasts. Live/dead assay for native yeast cells (a) and nCdS@Yeasts (b). (Green–live cells: Ex: 488 nm, Em: 525 nm; red–dead cells: Ex: 535 nm, Em: 615 nm. Scale bar: 20 μm.) (c) Growth curve of the nCdS@Yeasts (red curve) and the control native yeast cells (black curve). | |
The effect of regulation of cells on the physicochemical properties of the nCdS
Additionally, the optical properties of the nCdS in nCdS@Yeasts biohybrid systems can be tuned by the proteins.40–43 As a control model, the CdS nanoparticles (CdS NPs) with a similar size to those of intracellular nCdS were synthesized in vitro by adding a morphology regulator (bovine serum albumin BSA), in the absence of cells (Fig. S6†). The UV-vis diffuse reflectance spectra demonstrate that the nCdS isolated from nCdS@Yeasts have a wider region of visible light absorption than that of CdS NPs (Fig. 3a). The Tauc plots show the larger measured bandgap of isolated nCdS (2.40 eV) relative to that of CdS NPs (2.37 eV), which could prevent the recombination of electrons and holes (inset in Fig. 3a).44,45 The separation and transfer of photogenerated charge carriers of the hybrid system was investigated with steady-state photoluminescence (PL) spectra. As shown in Fig. S7a,† the PL emission intensity of the isolated nCdS from nCdS@Yeasts was lower than that of the pure CdS NPs, indicating more efficient electron–hole separation on the as-prepared hybrid. Moreover, EIS Nyquist plots of nCdS isolated from nCdS@Yeasts obtained under light irradiation exhibit a smaller arc radius compared with CdS NPs, confirming the higher electronic conductivity of nCdS due to effective electron transfer (Fig. S7b†). In addition, the XPS valence spectrum reveals an upshift in the valence band (VB) edge, from 1.52 eV for CdS NPs to 1.43 eV for isolated nCdS (Fig. S8†). On the basis of the optical bandgap, the calculation results show that the conduction band (CB) edge (−0.97 eV) of the isolated nCdS upshifts by ≈0.12 eV relative to that (−0.85 eV) of CdS NPs (Fig. 3b). The upshift of the CB position of the isolated nCdS indicated that the intracellular nCdS has better reduction potential of photogenerated electrons.46 The photogenerated electrons of nCdS were further confirmed by the transient photocurrent response. As a photocathode, the photocurrent density of the nCdS hybrid is consistently higher than that of CdS NPs (Fig. S9†), indicating that nCdS has a higher photogenerated charge carrier density under visible light.
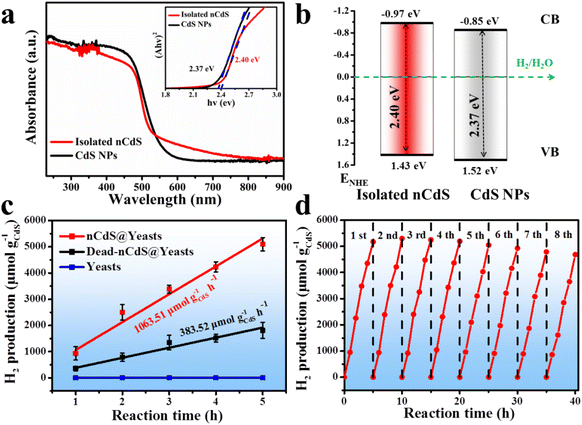 |
| Fig. 3 The optical properties of the nanodots and photocatalytic H2 production reaction. (a) UV-vis diffuse reflectance spectra (DRS); the inset shows corresponding plots of (Ahν)2versus hν. (b) Schematic of energy bandgap of isolated nCdS from nCdS@Yeasts and CdS NPs synthesized in vitro, respectively. (c) Hydrogen production versus reaction time data of nCdS@Yeasts, dead-nCdS@Yeasts and native yeasts under visible light irradiation. Hydrogen production is normalized by nCdS weight for comparative purposes. (d) Cycling experiments of nCdS@Yeasts, 5 h for each cycle. | |
Photocatalytic H2 production reaction
The photocatalytic ability of biohybrid systems is evaluated by photocatalytic hydrogen production under visible light irradiation (λ > 420 nm). As shown in Fig. 3c, the nCdS@Yeasts biohybrid systems show the highest H2-production abilities (up to 5095.47 μmol g–1 CdS) and the rate of photocatalytic H2 production reaches 1063.51 μmol g−1CdS h−1, which are very competitive with similar previously reported biological based catalysts (Table S1 in the ESI†). By comparison, the photocatalytic H2-production of native yeast cells is not detected. And the optimal amount of nCdS@Yeasts is 0.12 g, relating to nCdS@Yeasts population dispersion in the photoreactor system (Fig. S10†).47 The long-term stability of nCdS@Yeasts for photocatalytic H2 production is studied by eight successive recycling operations and each cycle comprises 5 h of visible light irradiation under the same conditions. As can be seen from Fig. 3d, the nCdS@Yeasts biohybrid systems still exhibit persistent high photoactivity and photostability. Further investigations show that the morphologies and crystalline structures of intracellular nCdS remain unchanged and the nCdS@Yeasts retain some reproductive capacity after visible light irradiation for 40 h (Fig. S11 and S12†). Based on the above results, when CdS nanodots are biosynthesized in situ inside the cell, the biohybrid microorganisms without a hydrogen production nature and photocatalytic properties are endowed with photocatalytic hydrogen production, which induces unnatural catalytic activity in the yeast cells and further expands the species of whole cells in photocatalytic fields.
Mechanistic insights of nCdS triggered for H2 production
To investigate the living yeast's contribution to the total hydrogen production of the hybrid system, the dead nCdS@Yeasts obtained by heat-treatment show lower H2 production (383.52 μmol g−1CdS h−1) compared with the live nCdS@Yeasts (1063.51 μmol g−1CdS h−1), indicating that the proteins in the living biohybrid system might play a role in hydrogen production (Fig. 3c). Hence, we explored the proteins that might be responsible via proteomics techniques. Sodium dodecyl sulfate-polyacrylamide gel electrophoresis (SDS-PAGE) was used to detect the proteins combined with nCdS, and the matrix assisted laser desorption ionization-time of flight mass spectrometry (MALDI-TOF-MS) detection results show that the binding proteins are ADH, glyceraldehyde-3-phosphate dehydrogenase (GAPD) and elongation factor 1-alpha (EF1A) in Fig. 4a. Moreover, the FT-IR spectra show that the relevant absorption peaks of nCdS-proteins have obvious changes compared with the natural yeast extract, further demonstrating the hybridization of intracellular proteins with the nCdS (Fig. S13†).
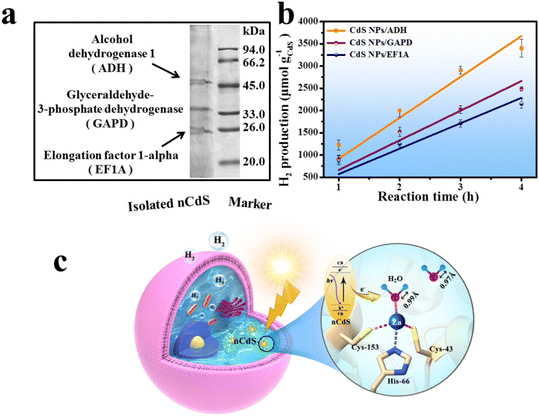 |
| Fig. 4 The tentative mechanism of photocatalytic reaction. (a) SDS-PAGE electrophoretogram and MALDI-TOF-MS analysis of isolated nCdS. (b) Hydrogen production of CdS NPs hybridized with ADH, GAPD and EF1A under visible light irradiation. (c) Schematic diagram for charge carrier separation on nCdS@Yeasts and the photocatalytic mechanism. | |
In order to further identify the roles of the detected proteins among photocatalytic hydrogen generation of nCdS@Yeasts, the CdS nanoparticles were synthesized in vitro under the mediation of ADH, GAPD and EF1A, respectively, and the hybrids were named CdS NPs/ADH, CdS NPs/GAPD and CdS NPs/EF1A. Then, the catalytic performances of the three hybrids were determined under the same test conditions as those for nCdS@Yeasts, respectively. In contrast, the catalytic properties of three pure enzymes and the CdS NPs were also determined. As shown in Fig. 4b, among the CdS-hybrid systems synthesized in vitro, the CdS NPs/ADH possess the highest hydrogen production ability (3407.51 μmol g–1 CdS), and their hydrogen production rate is 919.40 μmol g−1CdS h−1. However, the CdS NPs/GAPD and CdS NPs/EF1A hybrids have lower hydrogen production (2154.49 μmol g–1 CdS and 2486.94 μmol g–1 CdS), similar to that of CdS NPs (2368.37 μmol g–1 CdS) (Fig. S14†). The results reveal that GADP and EF1A have no obvious effect on photocatalytic hydrogen production unlike ADH. In addition, none of the three pure enzymes and BSA have a photocatalytic property (Fig. S14†). The simulation studies of the intracellular microenvironment show that only ADH plays an important role in photocatalytic H2-production among the hybrid systems of enzymes with CdS nanoparticles.
Based on the above research, the underlying mechanism of nCdS@Yeasts biohybrid systems for solar-to-hydrogen production was proposed in Fig. 4c. The photocatalytic hydrogen production of hybrid systems may mainly be attributed to the synergistic effect between intracellular nCdS nanodots and ADH. Otherwise, the density functional theory (DFT) calculations show that the H2O bound to ADH can be activated, namely, the bond lengths of H–O (0.99 Å) are longer than that of free H2O (0.97 Å), resulting in the activated H2O being more likely to gain electrons to be reduced. It is noteworthy that the photoexcited electrons from the CdS combined with ADH may be transferred directly to the activated H2O for H2 production owing to spatial co-localization.14 Therefore, the synergistic effect between nCdS and ADH can endow a microorganism with unnatural photocatalysis capability.
Conclusions
In summary, compared to native yeast cells without original hydrogen production and photosynthetic properties, the microorganisms are endowed with sustainable photocatalyzed hydrogen production by intracellular bionic nCdS inducing enzyme-catalyzed H2O-reducing reactions. Theoretical calculations based on experimental results suggest that the photoelectrons from nCdS can be transferred to the activated water molecules coordinated with the catalytically active zinc sites of ADH. On the premise that the enzyme interacts with the substrate, an accessible pathway via in situ material-based modification will endow microorganisms with newly designed photocatalysis functions, not merely for photosynthetic plant cells, but also for non-photosynthetic microorganism cells. This finding provides a feasible pathway for the sustainable production of light-driven chemicals to develop green energy alternatives by using a rationally designed whole-cell biohybrid system based on a non-photosynthetic cell without original chemical production abilities.
Experimental section
Materials and methods
All chemicals were of analytical grade and used without further purification. Cadmium chloride (CdCl2·2.5H2O) was purchased from Sinopharm Chemical Reagent Co., Ltd and sodium sulfide nonahydrate (Na2S·9H2O) was purchased from Tianjin Deen Reagent Company. The yeast purchased from ANGEL YEAST CO., Ltd was assigned to Angel yeast, a kind of hexose-fermenting Saccharomyces cerevisiae. For all the experiments, solutions were prepared using ultrapure water.
Characterization
The morphologies of nCdS@Yeasts and native yeast cells were characterized with a scanning electron microscope (SU8010). The nCdS were suspended in ultrapure water, and then dried on a piece of ultrathin carbon-coated copper grid for high-resolution TEM (HRTEM) analysis. The composite phases of the nCdS were identified with a Bruker D8 Advance X-ray powder diffractometer with graphite monochromatized Cu Kα (λ = 0.15406 nm). Fluorescent images were recorded using an Olympus Fluoview1000 confocal laser scanning microscope. Fourier transform infrared (FT-IR) spectra were obtained with a PerkinElmer Spectrum 100 spectrometer (USA) using KBr pellets (a 3
:
100 “sample to KBr” ratio).
Preparation of nCdS@Yeasts
The CdCl2 (0.5 M) and Na2S (0.5 M) aqueous solutions were freshly prepared. 300 mg of yeast powder was put into 50 mL of yeast extract peptone dextrose solution (YPD), containing 5 mM CdCl2 solution. The mixed solution was kept under moderate shaking (30 °C, 48 h) to let Cd2+ ions enter effectively inside the yeast cells. Then, the products were centrifuged to remove the excess Cd2+ ions that did not enter the cells, and yeast cells with intracellular Cd2+ ions could be obtained. Subsequently, the yeast cells with intracellular Cd2+ ions were suspended in 49.5 mL of HEPES (50 mM, pH = 8.5) aqueous solution, and 0.5 mL of 0.5 M Na2S aqueous solution was dropped in slowly. During the above process, a little dilute hydrochloric acid was used to make sure the pH was below 8.5. The above mixed solution was kept under moderate shaking for overnight incubation at 30 °C. During this process, the aging process began, during which the Cd2+ and S2− reacted to produce CdS nanodots (nCdS) inside the cells. The products were centrifuged to remove excess S2− and Cd2+ ions.
Extraction and isolation of the nCdS
The nCdS@Yeasts were crushed with an Ultrasonic cell crusher. The crushed sample was washed with double ultrapure water (5000 rpm, 5 min) to remove a mass of organics, and the supernatant was mixed with isopropanol (1
:
1) and then centrifuged for 10 min at 15
000 rpm.
Bio-TEM observation
The nCdS@Yeasts were fixed by glutaraldehyde at room temperature, and dehydrated through a graded ethanol series. Then, the fixed samples were embedded in EPOM812 and polymerized in an oven at 60 °C for 48 h. Sections of approximately 60 nm in thickness were cut with a Leica UC6 ultramicrotome. The ultrathin sections were stained with uranyl acetate and lead citrate. Finally, the samples were transferred to the copper grid for Bio-TEM observation.
Preparation of CdS NPs
5 mM CdCl2 aqueous solution was mixed with 5 mg mL−1 BSA solution, and moderately stirred for 8 h for the chelation of Cd2+ with BSA. Then, 5 mM Na2S aqueous solution was injected into the Cd2+-BSA solution. The mixed solutions were allowed to react for 24 h at 30 °C. Finally, the samples (CdS NPs) were collected (100 kDa MW, 10
000 rpm, 10 min) and dried under vacuum. Accordingly, the corresponding proteins were added during the chelation process to obtain CdS NPs/ADH, CdS NPs/GAPD and CdS NPs/EF1A hybrids.
Viability test
1 mL of 0.4% trypan blue solution and 9 mL of nCdS@Yeasts suspension were mixed. After 3 min, the stained products were observed with a light microscope. The cell viability of nCdS@Yeasts was further investigated with a Calcein-AM/PI staining assay. As a fluorescent dead-cell indicator, PI produces a bright red signal and Calcein-AM triggers green fluorescence for a live-cell indicator. 500 μL of buffer solution was mixed with 5 × 105 nCdS@Yeasts. 5 μL of Calcein-AM and 5 μL of PI were added to the above mixed liquor, and the suspension was incubated for 15 min at room temperature away from the light while shaking, and tested by confocal microscopy.
Cell-division tests
The nCdS@Yeasts and control yeast cells were cultured in YPD medium (30 °C, 150 rpm), respectively. The culture densities of the nCdS@Yeasts and native yeast cells were measured by turbidity (OD600) and then observed with a light microscope.
Photocatalytic H2 production
The photocatalyst was dispersed with constant stirring (400 rpm) in an 80 mL 1× PBS (pH = 7.5) mixed with 5 mM glucose solution (energy source) by using 150 mM L-cysteine as the hole sacrificial reagent. Prior to irradiation, the system was degassed under vacuum for 1 h in a 200 mL batch photocatalytic quartz reactor (Beijing Perfectlight). A xenon lamp (Microsolar 300, Beijing Perfectlight) with a 420 nm cut-off filter was used as a visible light source to induce the photocatalytic reaction. During the reaction, the intensity of the xenon lamp was adjusted to 2000 W m−2 and the reaction was maintained at 37 °C using a circulating water bath. A gas sample was analysed for hydrogen content with a gas chromatograph (GC9790II, FULI, China) equipped with a thermal conductivity detector. The amount of CdS in the samples remained consistent during the catalytic experiments. In addition, the catalytic performances of the three hybrids (CdS NPs/ADH, CdS NPs/GAPD and CdS NPs/EF1A hybrids) were determined under the same test conditions as those for nCdS@Yeasts.
The performance calculation procedure
The CdS content of nCdS@Yeasts was found to be 7.9 wt% by ICP-MS;
Amount of CdS used in 0.12 g nCdS@Yeasts = 7.9 wt% × 0.12 g = 0.09 g;
n
H2(mol) = VH2(L)/22.4 (L mol−1);
The performance of hydrogen (μmol g−1CdS h−1) = nH2 (μmol)/gCdS (g);
The H2-production rates were calculated via linear fits of reaction data averaged from three duplicate tests.
Cycling experiments of nCdS@Yeasts for photocatalytic H2 production
After one complete photocatalytic reaction, the nCdS@Yeasts were centrifuged (8000 rpm, 4 min), washed 5 times with ultrapure water, and the obtained nCdS@Yeasts were cultured overnight in 50 mL of YPD. Then, the medium was washed off with ultrapure water. The catalytic processes were carried out for the next cycle as described above. To evaluate the catalytic structural intactness after catalytic experiments, the morphologies of the nCdS@Yeasts were characterized again by SEM and XRD.
Protein separation and identification
8 μg of nCdS were mixed with 10 μL of 5 × SDS-PAGE loading buffer and heated (100 °C, 5 min). 12% SDS-PAGE analysis was performed by adding 20 μl of each sample and staining with Coomassie Brilliant Blue solution. Matrix assisted laser desorption ionization time-of-flight mass spectrometry (MALDI-TOF-MS) was carried out to analyse the type of proteins on a MALDI TOF/TOF 5800 mass spectrometer (AB SCIEX, USA).
Author contributions
Xiaoming Ma, Lin Yang and Zhengyu Bai conceived the project, designed the experiments and discussed the results. Peng Liu performed the experiments and wrote the manuscript. Yi Chang and Xueqin Ren helped to design the photocatalytic tests. Tingting Liu and Hongmin Meng conceived the protein identification. Xiangli Ru commented on the manuscript.
Conflicts of interest
There are no conflicts to declare.
Acknowledgements
This work was financially supported by the National Natural Science Foundation of China (No. 21877027, 52072114 and 21601052), Program for Science & Technology Innovation Talents in Universities of Henan Province (No. 23HASTIT002), the Henan Center for Outstanding Overseas Scientists (GZS2022017), the 111 Project (Grant No. D17007) and Program for Science Technology Innovation Teams in Universities of Henan Province (No. 19IRTSTHN023).
References
- D. Kim, K. K. Sakimoto, D. Hong and P. Yang, Angew. Chem., Int. Ed., 2015, 54, 3259–3266 CrossRef CAS PubMed.
- E. E. Moore, V. Andrei, S. Zacarias, I. A. C. Pereira and E. Reisner, ACS Energy Lett., 2020, 5, 232–237 CrossRef PubMed.
- W. Wei, P. Sun, Z. Li, K. Song, W. Su, B. Wang, Y. Liu and J. Zhao, Sci. Adv., 2018, 4, eaap9253 CrossRef PubMed.
- M. Ihara, H. Nishihara, K. S. Yoon, O. Lenz, B. Friedrich, H. Nakamoto, K. Kojima, D. Honma, T. Kamachi and I. Okura, Photochem. Photobiol., 2006, 82, 676–682 CrossRef CAS PubMed.
- K. A. Brown, M. B. Wilker, M. Boehm, G. Dukovic and P. W. King, J. Am. Chem. Soc., 2012, 134, 5627–5636 CrossRef CAS PubMed.
- C. Y. Lee, J. Zou, J. Bullock and G. G. Wallace, J. Photochem. Photobiol., C, 2019, 39, 142–160 CrossRef CAS.
- S. Cestellos-Blanco, H. Zhang, J. M. Kim, Y. X. Shen and P. Yang, Nat. Catal., 2020, 3, 245–255 CrossRef CAS.
- P. Gai, W. Yu, H. Zhao, R. Qi, F. Li, L. Liu, F. Lv and S. Wang, Angew. Chem., Int. Ed., 2020, 59, 7224–7229 CrossRef CAS PubMed.
- X. Wang, J. Zhang, K. Li, B. An, Y. Wang and C. Zhong, Sci. Adv., 2022, 8, eabm7665 CrossRef CAS PubMed.
- P. Q. Nguyen, N. M. D. Courchesne, A. Duraj-Thatte, P. Praveschotinunt and N. S. Joshi, Adv. Mater., 2018, 30, 1704847 CrossRef PubMed.
- T. C. Tang, B. An, Y. Huang, S. Vasikaran, Y. Wang, X. Jiang, T. K. Lu and C. Zhong, Nat. Rev. Mater., 2021, 6, 332–350 CrossRef CAS.
- Y. Ding, J. R. Bertram, C. Eckert, R. R. Bommareddy, R. Patel, A. Conradie, S. Bryan and P. Nagpal, J. Am. Chem. Soc., 2019, 141, 10272–10282 CrossRef CAS PubMed.
- S. Koh, Y. Choi, I. Lee, G. M. Kim, J. Kim, Y. S. Park, S. Y. Lee and D. C. Lee, J. Am. Chem. Soc., 2022, 144, 10798–10808 CrossRef CAS PubMed.
- H. X. Han, L. J. Tian, D. F. Liu, H. Q. Yu, G. P. Sheng and Y. Xiong, J. Am. Chem. Soc., 2022, 144, 6434–6441 CrossRef CAS PubMed.
- K. K. Sakimoto, A. B. Wong and P. Yang, Science, 2016, 351, 74–77 CrossRef CAS PubMed.
- C. Liu, J. J. Gallagher, K. K. Sakimoto, E. M. Nichols, C. J. Chang, M. C. Y. Chang and P. Yang, Nano Lett., 2015, 15, 3634–3639 CrossRef CAS PubMed.
- B. Wang, C. Zeng, K. H. Chu, D. Wu, H. Y. Yip, L. Ye and P. K. Wong, Adv. Energy Mater., 2017, 7, 1700611 CrossRef.
- Y. Honda, H. Hagiwara, S. Ida and T. Ishihara, Angew. Chem., Int. Ed., 2016, 55, 8045–8048 CrossRef CAS.
- H. Wang, H. Li, M. Zhang, Y. Song, J. Huang, H. Huang, M. Shao, Y. Liu and Z. Kang, ACS Appl. Mater. Interfaces, 2018, 10, 16308–16314 CrossRef CAS.
- K. Xiao, T. H. Tsang, D. Sun, J. Liang, H. Zhao, Z. Jiang, B. Wang, J. C. Yu and P. K. Wong, Adv. Energy Mater., 2021, 11, 2100291 CrossRef CAS.
- H. Shen, Y. Z. Wang, G. Liu, L. Li, R. Xia, B. Luo, J. Wang, D. Suo, W. Shi and Y. C. Yong, ACS Catal., 2020, 10, 13290–13295 CrossRef CAS.
- B. Cambou and A. M. Klibanov, Ann. N. Y. Acad. Sci., 1984, 434, 219–223 CrossRef CAS.
- L. Bering, J. Thompson and J. Micklefield, Trends Chem., 2022, 4, 392–408 CrossRef CAS.
- C. Klaus and S. C. Hammer, Trends Chem., 2022, 4, 363–366 CrossRef CAS.
- G. Hughes and J. C. Lewis, Chem. Rev., 2018, 118, 1–3 CrossRef CAS PubMed.
- H. Chen, Z. Bai, X. Dai, X. Zeng, Z. P. Cano, X. Xie, M. Zhao, M. Li, H. Wang, Z. Chen, L. Yang and J. Lu, Angew. Chem., Int. Ed., 2019, 58, 6663–6668 CrossRef CAS PubMed.
- O. Bachar, M. M. Meirovich, Y. Zeibaq and O. Yehezkeli, Angew. Chem., Int. Ed., 2022, 61, e202202457 CrossRef CAS PubMed.
- J. E. Cournoyer, S. D. Altman, Y. L. Gao, C. L. Wallace, D. Zhang, G. H. Lo, N. T. Haskin and A. P. Mehta, Nat. Commun., 2022, 13, 2254 CrossRef CAS PubMed.
- J. Guo, M. Suástegui, K. K. Sakimoto, V. M. Moody, G. Xiao, D. G. Nocera and N. S. Joshi, Science, 2018, 362, 813–816 CrossRef CAS PubMed.
- B. V. Plapp, H. A. Charlier and S. Ramaswamy, Arch. Biochem. Biophys., 2016, 591, 35–42 CrossRef CAS PubMed.
- S. B. Raj, S. Ramaswamy and B. V. Plapp, Biochemistry, 2014, 53, 5791–5803 CrossRef CAS PubMed.
- B. V. Plapp, Arch. Biochem. Biophys., 2010, 493, 3–12 CrossRef CAS PubMed.
- S. R. Guntupalli, Z. Li, L. Chang, B. V. Plapp and R. Subramanian, Biochemistry, 2021, 60, 663–677 CrossRef CAS PubMed.
- A. Dołęga, Coord. Chem. Rev., 2010, 254, 916–937 CrossRef.
- B. L. Vallee and D. S. Auld, Proc. Natl. Acad. Sci., 1990, 87, 220–224 CrossRef CAS PubMed.
- A. Sankhla, R. Sharma, R. S. Yadav, D. Kashyap, S. L. Kothari and S. Kachhwaha, Mater. Chem. Phys., 2016, 170, 44–51 CrossRef CAS.
- S. Pihlasalo, L. Auranen, P. Hänninen and H. Härmä, Anal. Chem., 2012, 84, 8253–8258 CrossRef CAS PubMed.
- Y. Su, Z. Zhang, H. Liu and Y. Wang, Appl. Catal., B, 2017, 200, 448–457 CrossRef CAS.
- Y. Li, H. Wang and S. Peng, J. Phys. Chem. C, 2014, 118, 19842–19848 CrossRef CAS.
- S. H. Kang, K. N. Bozhilov, N. V. Myung, A. Mulchandani and W. Chen, Angew. Chem., Int. Ed., 2008, 47, 5186–5189 CrossRef CAS PubMed.
- J. M. Jacob, P. N. L. Lens and R. M. Balakrishnan, Microb. Biotechnol., 2016, 9, 11–21 CrossRef CAS PubMed.
- G. Ulloa, B. Collao, M. Araneda, B. Escobar, S. Álvarez, D. Bravo and J. M. Pérez-Donoso, Enzyme Microb. Technol., 2016, 95, 217–224 CrossRef CAS PubMed.
- L. Shen, N. Bao, P. E. Prevelige and A. Gupta, J. Phys. Chem. C, 2010, 114, 2551–2559 CrossRef CAS.
- R. Vogel, P. Hoyer and H. Weller, J. Phys. Chem. C, 1994, 98, 3183–3188 CrossRef CAS.
- Z. Y. Guo, C. X. Li, M. Gao, X. Han, Y. J. Zhang, W. J. Zhang and W. W. Li, Angew. Chem., Int. Ed., 2021, 60, 274–280 CrossRef CAS PubMed.
- J. Di, J. Xia, M. F. Chisholm, J. Zhong, C. Chen, X. Cao, F. Dong, Z. Chi, H. Chen, Y. X. Weng, J. Xiong, S. Z. Yang, H. Li, Z. Liu and S. Dai, Adv. Mater., 2019, 31, 1807576 CrossRef PubMed.
- S. Cao and L. Piao, Angew. Chem., Int. Ed., 2020, 59, 18312–18320 CrossRef CAS PubMed.
|
This journal is © The Royal Society of Chemistry 2023 |
Click here to see how this site uses Cookies. View our privacy policy here.