DOI:
10.1039/D2GC03095K
(Critical Review)
Green Chem., 2023,
25, 808-832
A sustainable waste-to-protein system to maximise waste resource utilisation for developing food- and feed-grade protein solutions†
Received
17th August 2022
, Accepted 7th December 2022
First published on 8th December 2022
Abstract
A waste-to-protein system that integrates a range of waste-to-protein upgrading technologies has the potential to converge innovations on zero-waste and protein security to ensure a sustainable protein future. We present a global overview of food-safe and feed-safe waste resource potential and technologies to sort and transform such waste streams with compositional quality characteristics into food-grade or feed-grade protein. The identified streams are rich in carbon and nutrients and absent of pathogens and hazardous contaminants, including food waste streams, lignocellulosic waste from agricultural residues and forestry, and contaminant-free waste from the food and drink industry. A wide range of chemical, physical, and biological treatments can be applied to extract nutrients and convert waste-carbon to fermentable sugars or other platform chemicals for subsequent conversion to protein. Our quantitative analyses suggest that the waste-to-protein system has the potential to maximise recovery of various low-value resources and catalyse the transformative solutions toward a sustainable protein future. However, novel protein regulation processes remain expensive and resource intensive in many countries, with protracted timelines for approval. This poses a significant barrier to market expansion, despite accelerated research and development in waste-to-protein technologies and novel protein sources. Thus, the waste-to-protein system is an important initiative to promote metabolic health across lifespans and tackle the global hunger crisis.
Introduction
Despite continuous efforts to achieve the goal of ‘zero hunger’ Sustainable Development Goals (SDG), the global undernourished population is projected to increase from 688 million to 841 million by 2030.1 A major contributor to this forecast is the occurrence of war and disruptive political situations, and failure to distribute economically accessible food to the poorest societies on our planet. In addition, increasing strains on food security are exacerbated by the unsustainable reliance on finite natural capital resources such as land and water, that are required for traditional farming techniques. Animal-sourced protein is a highly resource-intensive and nutritionally inefficient method of food production based on nitrogen utilisation yet constitutes 18% of the current global protein supply.2–4 Indeed, the projected increase in demand for meat protein (to almost double by 2050) poses significant environmental concerns, particularly in relation to land and water availability and greenhouse gas emissions.5–8 The Covid-19 pandemic has threatened global food supply chains at multiple levels, causing interruptions to the planting, harvesting, and transportation of crops.9–11 Such interruptions exacerbate the issue of food security with the worst post-pandemic scenario estimated to produce 909 million people with undernutrition by 2030,12–14 highlighting the need for a secure yet sustainable food production system.
Rising food waste presents as an abundant resource for alternative protein solutions.15–18 It is estimated that one-third of food produced globally is underutilised for reasons related to logistics of supply and demand. This trend is evident in both developed regions with overnutrition and less developed countries with increasing rates of undernutrition, and is equivalent to 1.3 billion tonnes of wasted food which provides sufficient resources to feed 2 billion people worldwide.15 Globally, considerable amounts of carbon-containing and nutrient-rich waste are generated from the food and drink sector. For instance, in the UK, 1.5 million tonnes of waste is created from the production of meat, dairy, fruits, vegetables, starch products, beverages, brewing by-products, and other food products.19,20
This review focuses on the contaminant-free organic component of three broad waste streams that can be converted to food-grade or animal feed-grade protein through sustainable protein production technologies. We consider (i) food waste streams present in organic fraction of municipal solid waste (OFMSW); (ii) lignocellulosic waste, which is defined here as the lignocellulosic agricultural residues from crop cultivation (e.g. straw) as well as forestry waste (e.g. wood chips); and (iii) food industry waste in the form of organic gas, liquid, and solid streams generated from processing and manufacturing within the food and drink sector. These waste streams offer considerable potential for resource recovery and protein production due to the high concentrations of nutrients, degradable organic compounds and the absence of pathogens, toxic metals, and other hazardous contaminants.
Non-organic wastes have been investigated for a ‘power to protein’ approach;21,22 however, here we explore a range of sustainable technologies to extract or convert nutrients and organic compounds present in contaminant-free waste to produce food- or feed-grade protein. Utilisation of microbial biotechnologies such as fermentation can achieve yields of approximately 40% cell biomass from dry waste matter.23 At least 80 species have been reported to produce microbial protein, but a better understanding of the microbes involved and their potential for protein recovery from waste is needed.24 Higher organisms such as insects can also be used as bio-converters within a waste-to-protein system. These higher organisms typically attain a maximum upgrading efficiency of only 10% but can also yield biomass components of significant functional value. Additionally, biochemical and physical treatments can be used to recover extra nutrients from waste streams, upgrade waste-to-protein systems, or convert waste-carbon to fermentable sugars and other platform chemicals for subsequent conversion to protein. Despite the advances in individual technologies, critical gaps remain in the development of innovative systems that integrate these technologies for optimised protein recovery from diverse waste streams.
We define a ‘waste-to-protein system’ as a collection of pathways using process technologies to recover food-grade and/or feed-grade protein from contamination-free organic waste resources. Accordingly, ‘waste-to-protein’ refers to the proteins derived or produced from non-contaminated food-safe or feed-safe organic materials exhibiting compositional quality suitable for valuable upgrading. Food-grade and feed-grade proteins have differing requirements with regards to feedstock quality (food-safe vs. animal feed-safe, respectively), and must comply with hygienic quality and safety standards set by regulators which vary significantly by country.25
The primary aim of this article is to provide an overview of the strategies and pathways with the potential to transform globally abundant contaminant-free waste into a sustainable ‘waste-to-protein system’ to achieve global protein security and contribute to a circular-economy aspiration.26,27 To achieve this, we critically evaluate the viability of food-safe and feed-safe waste streams as ‘waste-to-protein’ resources, with an emphasis on their abundance and biochemical composition. We then appraise the technologies available for waste-to-protein conversion, focusing on three promising, evidence-based pathways: biochemical and physical treatment, microbial protein, and insects as bio-converters. Finally, we propose a sustainable ‘waste-to-protein’ system that maximises waste resource utilisation for the development of food-grade and feed-grade protein solutions to promote global food security and ameliorate the hunger pandemic.
Waste-to-protein sources
Feed-grade organic fraction of municipal solid waste
Annual global household waste generation is equivalent to 2.01 billion tonnes of municipal solid waste (MSW). The organic fraction of municipal solid waste (OFMSW) accounts for around 40% of global MSW generated each year, presenting as an abundant source of feed-grade organic waste for a waste-to-protein system.28,29 It is an overly abundant resource for high-income countries, and a valuable nutrient resource for low-income countries due to its macronutrient profile.30Fig. 1 illustrates the rate of MSW generation by country, as well as the regional composition. Rates of generation range from 4.94 kg per capita per day (Antigua and Barbuda) to 0.14 kg per capita per day (Nepal). While higher quantities of MSW are produced by high-income countries (Fig. 1a), low-income countries tend to generate a larger organic fraction (food and garden waste) compared to high-income nations (Fig. 1b). On average, 184 g of OFMSW is generated per capita per day with crude protein content ranging from 4.35 g per capita per day (South Asia) to 31 g per capita per day (Caribbean). MSW is projected to increase by 70% in developing countries, and a marked increase in MSW generation has been observed in areas with rapid urbanisation.15,31 Developing regions such as Africa and South East Asia also account for 91.8% of worldwide undernourishment, highlighting the urgent need to explore new protein solutions, e.g. waste-to-protein, to meet increasing nutrient and protein demands in these areas.1
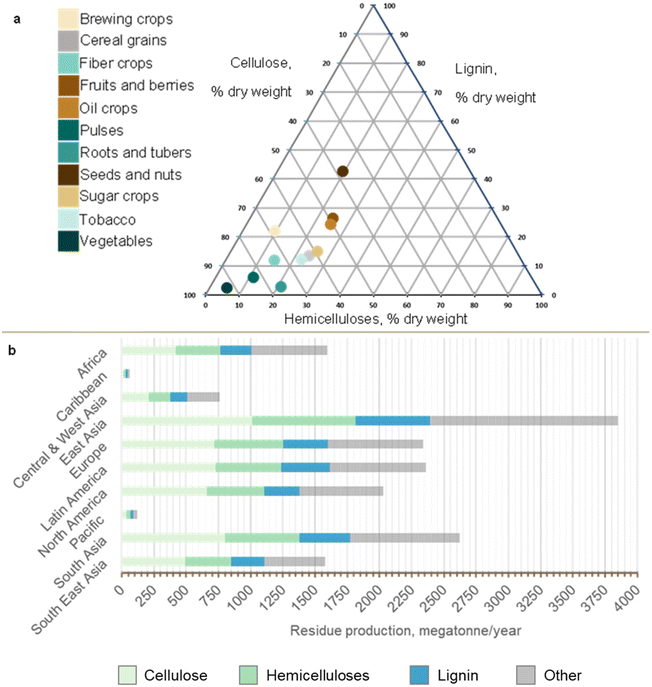 |
| Fig. 1 Global production of Municipal Solid Waste (MSW). (a) Average MSW generation (kg per capita per day) was calculated for each country using data from literature15,30,38,39 where MSW generation was plotted according to a colour gradient scale ranging from low (minimum 0.14 kg per capita per day) to high (maximum 4.93 kg per capita per day). (b) Regional OFMSW composition and average lipid, carbohydrate and protein contents (g per capita per day) were calculated from previously reported values.15,40 Detailed data can be found in ESI-1 and ESI Table ST1.† | |
Safety of feed-grade organic fraction of municipal solid waste
Crops may accumulate antibiotic resistant genes (ARGs) from organic fertiliser (e.g. manure) applied to the soil, potentially contaminating sources of OFMSW.32,33 Furthermore, OFMSW sourced from mixed domestic waste may be further contaminated due to direct contact with other ARG- and pathogen-rich wastes.32 Pre-treatment of OFMSW prior to protein valorisation is therefore imperative to mitigate health effects posed by such contaminants. Ozonation is commonly used to treat wastewater containing ARGs and has been applied to solid wastes in previous works.34–37 However, it requires tightly controlled conditions that are highly dependent on solid waste feedstock properties, such as pH, water content, particle size.35 Furthermore, the impact on protein quality resulting from ozonation pre-treatment of OFMSW requires further investigation to assess the potential for integration into a waste-to-protein valorisation process system. On the other hand, thermal treatments (e.g. microwaves41) and high-pressure processing technologies42,43 have been reported to destroy pathogens through disruption of cell wall structure, while simultaneously increasing protein and sugar solubility.44 However, ARG reduction potential of such technologies is less understood.
Lignocellulosic waste
Agricultural residues.
Lignocellulosic waste from agriculture is a globally distributed, carbon-rich, non-contaminated and food-safe resource presenting as a potential candidate for the recovery of nutritionally valuable protein.11 Although different countries and regions exhibit varying production rates of agricultural crops, all countries generate lignocellulosic waste in the form of agricultural residues.45,46 In this review, we define agricultural crops as terrestrial plants cultivated on a large scale including cereal grains, fruits, vegetables, oil crops, and sugar crops. We assessed the potential carbon and nutritional values of food-grade lignocellulosic wastes from agriculture sector by examining the biochemical composition of non-edible parts of crops, i.e. agricultural residues (Fig. 2). Crude protein content often constitutes less than 8% of agricultural residues. However, sustainable technologies could be deployed to convert the lignocellulosic component to protein. For example, microbial strains capable of metabolising lignocellulosic feedstock could be used to produce food-grade or feed-grade protein.
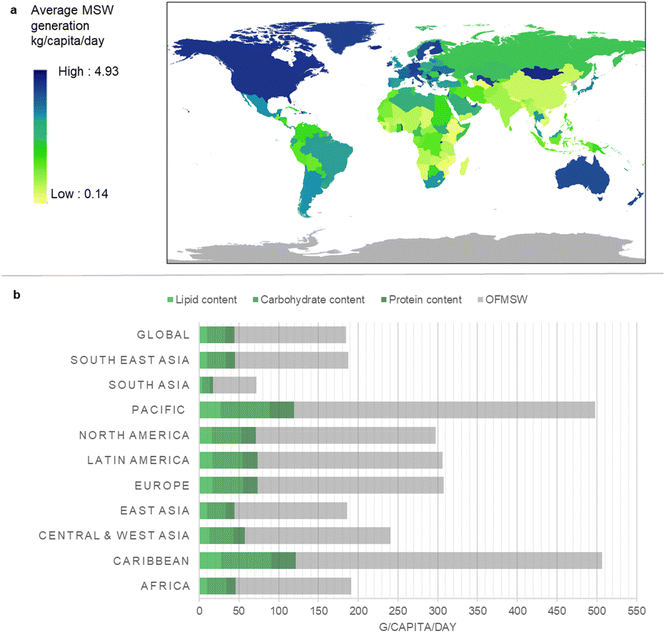 |
| Fig. 2 Biochemical analysis of agricultural lignocellulosic residues. Agricultural products were categorised as: brewing crops; cereal grains; fibre crops; fodder; fruits and berries; oil crops; pulses; roots and tubers; seeds and nuts; sugar crops; tobacco; and vegetables. (a) Biochemical composition of lignocellulosic component of agricultural product residues based on the Phyllis database.47 Values are given as a % of dry weight. (b) Regional lignocellulosic production rate and its biochemical composition as part of the total agricultural residue production. Residue production was estimated by applying residue production ratios to production values for 2018 for each region.47,48 Detailed data can be found in ESI-2 and ESI Table ST2.† | |
Fig. 2a presents the lignocellulosic contents of the main agricultural product residues, ranging from 34% to 60% for lignin, 15% to 43% for cellulose and 17% to 36% for hemicelluloses. We focus on cellulose, hemicelluloses and lignin but acknowledge that other cell wall components (e.g. pectins) and intracellular components (e.g. oligosaccharides and starch) warrant future exploratory research.
Geographical variations in climate and soil conditions contribute to regional differences in production rate and biochemical composition of agricultural residues. East Asia is the largest global producer of lignocellulosic agricultural residues (2389 megatonnes per year), which constitutes approximately 62% of the total residue production. In comparison, the Caribbean agricultural sector generates only 44 megatonnes per year of lignocellulosic residues, constituting 68% of its total residue production. Overall, total residue production is higher in South and Southeast Asia. However, other regions including both high-income and low-income countries also show abundant agricultural residue production, highlighting global potential for lignocellulosic conversion of crop residues to protein (Fig. 2b).
Forestry residue.
Forestry residue is another lignocellulosic waste source.45,49,50 Global forest resources amount to 600
066 megatonnes per year and comprise of above- and below-ground biomass, plus 67
000 megatonnes per year of deadwood. The global distribution and analyses of forestry biomass and corresponding residue biomass can be found in published databases.51 Residues generated by forest management, harvesting and processing (particularly in regions with active forestry industries such as Canada and parts of Latin America, and from areas employing tree-cutting for wildfire prevention) could provide substantial lignocellulosic feedstock for a waste-to-protein process system.52,53 The fact that upgrading of lignocellulosic content from forestry residues to human food or animal feed has not taken any dimensions of scale relates to aspects of logistics and particularly cost competitiveness of the products. Furthermore, protein derivation from forestry waste for human consumption is particularly problematic, as forestry land can have significant contamination e.g. those used for phytoremediation.
Safety of lignocellulosic waste
Based on safety concerns, pre-treatment may be required to remove harmful toxins prior to protein or carbohydrate extraction processes. Technologies such as pressurised liquid extraction (PLE), supercritical fluid extraction (SFE) and subcritical water extraction have shown promising effectiveness at reducing the content of organic pesticides and heavy metals contained in lignocellulosic waste streams.54–58 However, they are rarely employed for industrial processing due to their high cost.57,58 Furthermore, due to the high purity requirements required for human and animal consumption, intense research efforts are required to optimise pre-treatment to achieve maximum contaminant reduction while mitigating deleterious chemical alterations of feedstock compounds, which can significantly reduce downstream efficiency and yield of protein extraction and bioconversion processes.56 Combined contaminant remediation and protein value-upgrading is a promising approach that integrates process stages through multi-objective bioconversion. For example, one strategy is the use of fungal strains capable of simultaneously degrading pollutants/contaminants while assimilating lignocellulose into biomass through subsequent saccharification and fermentation (SSF).59–63 However, the efficacy of this approach is highly dependent on the feedstock composition, process conditions and the strain type employed. Furthermore, due to the high content of chemically stable lignin, a co-culture containing a lignin-degrading species, such as white rot fungi species, may be required to maximise feedstock extraction efficiency and reduce downstream separation burden, at the expense of increased bioremediation process complexity, due to difference in optimal growth conditions of microbial strains.
Food and drink industry waste
Quantifying food industry waste production is challenging, due to its complex nature and enormous scale. We have therefore selected quantifiable waste streams of two industries (shrimp fishing, and brewer's spent grain) as examples to show the potential of food industry waste within a waste-to-protein system.
Shrimp waste
The shrimp fishing industry is a good target for waste-to-protein resource recovery, being well-established in Africa and South East Asia and generating 6–8 megatonnes per year of protein-richorganic waste (40% protein) during the processing phase.64 Shrimp waste also contains chitin, which constitutes 20–30% of its biomass. Chitin can be converted to water-soluble chitosan, a value-added polysaccharide with a range of functional properties and industrial applications (e.g. drug delivery, food thickening and stabilising).65,66 Combined recovery of protein and value-added polysaccharides such as chitosan has the potential to improve the economics and sustainability of waste-to-protein system processes.
Brewer's spent grain
The most abundant by-product generated by the brewing industry is brewer's spent grain (BSG), which offers great potential for protein recovery due to its protein and carbon-rich chemical composition.67 The major component of BSG tissues are the cell walls consisting primarily of non-starch polysaccharides (NSP), some of which are lignified.68 The NSP include cellulose and non-cellulosic polysaccharides (‘hemicelluloses’), particularly arabinoxylans which constitute 25–52% of BSG composition. BSG also has high protein contents, comprising 15–31% of its composition.69,70 Research efforts have focussed on existing chemical processes (e.g. solvent pre-treatment followed by enzymatic hydrolysis) to fractionate the protein components and convert NSP to fermentable sugars for microbial protein production.71,72 However, optimised routes to integration of BSG into the conventional feed and food supply chains using novel processing methods remains as an outstanding research gap.
Safety of food and drink industry waste
Despite the relative lack of chemical contaminants (for example heavy metals), waste streams from the food and drink sector are highly susceptible to contamination through growth of potentially pathogenic microbes.73 Employment of controlled pasteurisation at sufficiently high temperatures before processing is therefore used to prevent contamination of downstream products. Integration of continuous toxicological and pathogen testing of feedstock pre- and post-processing should also be employed to assure food/feed safety and for adequate quality control.73 However, research and development of novel pasteurisation technologies such as high-pressure processing is required, as current high temperature processes have been shown to impact sensory and functional properties of valorised protein.43,74
Sustainable protein production technologies
Promising technologies presenting sustainable methods of protein recovery include: (i) biochemical, chemical, and physical treatments, (ii) bioconverters (microbial protein and insects).
Biochemical, chemical and physical treatments
A wide range of biochemical, chemical, or physical treatments can be applied to contaminant-free organic waste streams to extract valuable proteins, produce protein hydrolysates with favourable functionality, palatability and reduced allergenicity, or to transform carbohydrates to sugars as feedstock for bioconversion technologies.75,76
Protein extraction and purification technologies
Membrane filtration (e.g. ultrafiltration, reverse osmosis) and precipitation (e.g. isoelectric precipitation, salting out, organic solvent methods) and adsorption technologies offer great advantages as cost-effective techniques for continuous protein extraction from waste feedstock. The advantages and drawbacks of these technologies with regards to process operation and product safety/nutrition are summarised in Table 1.
Table 1 Protein extraction and purification technologies: advantages and drawbacks of process efficiency/operation and product safety/nutrition (MW = molecular weight; COD = chemical oxygen demand; OPEX = operating expenditure; CAPEX = capital expenditure)
Technology |
Extraction and purification technologies |
Ref. |
Membrane filtration |
Process advantages
|
Process drawbacks
|
99, 104, 110, 115 and 191–195 |
Can be integrated with simple pre-treatment processes (centrifugation, pre-filtration, dissolved air flotation) to reduce fouling by waste particles containing fat, starch, and high MW proteins |
Protein agglomeration on membrane surface leads to concentration polarisation and pore blocking, which causes severe membrane fouling |
High yield of non-denatured proteins due to low operating temperatures |
High OPEX/CAPEX for membrane regeneration/replacement |
Low energy consumption |
|
Membrane unit configurations such as rotating disk membranes can reduce fouling by increasing shear rate |
Permeate may contain high COD due to presence of residual waste and chemicals used in pre-treatment steps, requiring further downstream processing before discharge |
Cascading membrane systems of varying pore size can increase protein yield and water recovery while reducing COD of effluent |
Throughput levels capped by flooding and loading limits |
Modular and flexible usage, highly scalable for industrial processing with small physical footprint |
Use of harsh chemicals may be required to regenerate fouled membrane |
Backflushing and rinsing of the membrane during or after operation can decrease fouling |
Potentially large solvent inventory for cleaning purposes |
Safety/nutritional advantages
|
Safety/nutritional drawbacks
|
Enhanced functional/nutritional properties of extracted proteins compared to precipitation |
High MW proteins associated with allergenicity and digestibility issues (post-extraction hydrolysis may be required) |
Denaturation of high MW proteins may occur which can improve digestibility and reduce allergenicity |
|
Precipitation (organic solvent, pH-shift, salting-out) |
Process advantages
|
Process drawbacks
|
95, 104, 108, 110, 196 and 197 |
Increased efficiency when integrated with membrane filtration |
Low overall protein yield, sensitive to impurities in feed |
Isolate can be processed downstream (e.g., enzymatic hydrolysis) to produce shorter peptides with higher solubility and improved functionality |
pH shift requires controlled addition of harsh alkali/acid chemicals |
|
Chemical/salt addition may introduce further impurities, intensifying the downstream purification load |
Long-established technology in bioprocessing industry |
High environmental impact when using organic solvents |
Relatively simple, inexpensive and highly scalable process (especially salting-out) |
Intense centrifugation is often required downstream to remove chemicals and impurities, increasing energy costs |
Mild operating temperature (but must be controlled carefully for sensitive proteins) |
Operating at extreme pH may result in functionality loss of many proteins in waste stream |
Safety/nutritional advantages
|
Safety/nutritional drawbacks
|
Products often used as emulsifiers, stabilisers, and foaming agents and as fortifiers to enhance the nutritional value of food products due to favourable functionality |
High MW proteins associated with allergenicity and digestibility issues (post-extraction hydrolysis may be required) |
Precipitating agents or flocculants used to increase efficiency are food safe |
Use of acid-alkali impacts functionality and amino acid content of proteins due to denaturation effects |
Adsorption |
Process advantages
|
Process drawbacks
|
99, 198 and 199 |
Mesoporous silica structure can be modified to include functional groups to extract specific proteins |
Unmodified silica adsorbent is electronically neutral and has lower affinity for charged proteins, resulting in leaching of proteins |
Low operating temperature |
Increased CAPEX/OPEX due to adsorbent replacement/regeneration |
Adsorbent can be used to immobilise enzymes which hydrolyse incoming feed (e.g., to hydrolyse carbohydrates and lipids in waste stream) |
Enzyme leaching from surface can occur for poorly selected adsorbent (enzyme regeneration can also be an issue) |
Effective for targeted extraction of bioactive proteins |
Difficult and expensive to modify silica adsorbent |
Many adsorbents are low-cost (e.g. silica) |
Extraction efficiency and selectivity is highly dependent on process conditions and adsorbent surface structure |
Safety/nutritional advantages
|
Safety/nutritional drawbacks
|
Products often used as emulsifiers, stabilisers, and foaming agents and as fortifiers to enhance the nutritional value of food products due to favourable functionality |
Relatively little available research on the mechanisms of protein–surface interactions and effect on protein structure (especially for complex waste feedstock) |
Membrane filtration.
Membrane filtration has been well-established as a physical treatment to mitigate nutrient concentration and carbon oxygen demand (COD) of industrial effluents, as in the dairy industry to recover value-added caseins and whey proteins from wastewater.77 Such methods have demonstrated high efficiency, for example Das et al. (2015) were able to achieve 90% protein recovery from whey waste using combined ultrafiltration and nanofiltration.78 Filtration methods are also low in energy consumption and protein denaturation but are challenged by performance issues such as membrane fouling caused by particle deposition and coagulation of charged proteins at the membrane surface. This issue has been observed in various studies, including tuna and dairy wastewater processing, as well as commercially, for example during production trials of flavour enhancer Mycoscent (Quorn), a concentrate containing glutamate and ribonucleotides from mycoprotein wastewater.79,80
Precipitation.
A variety of methods exist to precipitate proteins from solution, including isoelectric precipitation, salting out, and organic solvent methods. Typically, precipitation is a rapid, easily scalable process that can be operated at low temperatures, enabling high throughput, low heat duty and recovery of proteins without denaturation effects. Taskila et al. (2017) investigated the use of low-temperature evaporation followed by ethanol precipitation to recover value-added proteins from potato fruit juice. Implementation at pilot scale demonstrated a 50% recovery of proteins from industrial starch waste streams.81 Xu et al. (2019) studied epigallocatechin-3-gallate (a polyphenol derived from green tea) as a precipitating agent for protein valorisation from soy whey wastewater, achieving a high recovery of 60.7% with a protein purity of 69.51%.82
Adsorption.
Adsorption technologies have been explored primarily to extract valuable enzymes from waste, as detailed in a review by Shahid et al. (2021). Typically, various structural forms of mesoporous silica with modified surface properties are employed for targeted protein valorisation and are capable of operating at low temperatures. However, residence time, adsorption capacity and operating pH vary significantly as a function of adsorbent, substrate, and target protein of study.79
Despite promising results of new filtration, precipitation, and adsorption technologies, further studies are required to determine recovery performance and protein structure alterations when targeting proteins of high nutritional value from a wider range of waste streams. Research efforts focused on adsorbent/membrane regeneration and precipitant recovery and recycle capacity are also essential to ensure sustainability and economic viability of extraction.
Assisted extraction technologies.
A variety of technologies can be used in hybrid with extraction and hydrolysis technologies to improve process efficiency and environmental impact, while improving the functional properties of product. These include hydrodynamic cavitation extraction (HCE), microwave assisted extraction (MAE), pulsed electric fields (PEF) and ultrasound assisted extraction (UAE), the working mechanisms of which have been reviewed in-depth in other works.83–107 Key advantages and drawbacks of these technologies with regards to process operation and product safety/nutrition are summarised in Table 2.
Table 2 Assisted extraction technologies: advantages and drawbacks of process efficiency/operation and product safety/nutrition (OPEX = operating expenditure; CAPEX = capital expenditure)
Technology |
Assisted extraction technologies |
Ref. |
Hydrodynamic cavitation extraction (HCE) |
Process advantages
|
Process drawbacks
|
90, 94, 96, 100 and 107 |
Good scalability for continuous processing compared with UAE |
Relatively little available research (denaturation effects and efficiency at industrial scale are largely unknown) |
Lowers CAPEX, OPEX and production time |
|
Higher efficiency compared with UAE |
|
Recent scale-up studies have demonstrated improved economics at pilot/industrial scale compared to lab scale |
|
Can be used in conjunction with other extraction techniques (e.g., enzymatic hydrolysis, solvent extraction) to significantly increase yield |
Process efficiency is highly dependent on interaction between reactor configuration, operational parameters, and feedstock properties |
Potential use as one-step valorisation process, reducing downstream processing burden |
|
Relatively low environmental impact due to reduced energy consumption |
|
Safety/nutritional advantages
|
Safety/nutritional drawbacks
|
Enhances nutritional quality, solubility, and digestibility of product |
Evaluation of potential toxic by-product generation is yet to be fully evaluated |
Microwave assisted extraction (MAE) |
Process advantages
|
Process drawbacks
|
83, 84, 88, 90, 91, 93, 98, 103 and 105 |
Can be used in conjunction with other extraction techniques (e.g., enzymatic hydrolysis, solvent extraction) to significantly increase yield |
In conjunction with solvent extraction, organic/inorganic solvents are preferred to water due to lower relative electrical permittivity |
Relatively simple and inexpensive compared to SFE |
Relatively difficult to operate compared to UAE |
Reduces energy consumption and environmental impact of process |
High equipment CAPEX |
Shorter extraction time compared to UAE |
Denaturation an issue when operating at high power and prolonged time periods |
Can reduce solvent/chemical requirement of extraction process |
|
Safety/nutritional advantages
|
Safety/nutritional drawbacks
|
Reported to assist pathogen expulsion during thermal pre-treatment of waste by disrupting cell wall structure |
Relatively little research to understand the full effects of MAE on food/feed products and their safety |
Enhances nutritional quality, solubility, and digestibility of product |
|
Pulsed electric fields (PEF) |
Process advantages
|
Process drawbacks
|
85, 86, 90, 99, 101 and 106 |
|
Modular and flexible technology is highly scalable for continuous processing |
Can cause air bubble entrapment in the treatment chamber, lowering efficiency |
|
Significantly increases protein yield and functionality when used in combination with other technologies (e.g., enzymatic, acid/alkali hydrolysis) |
Further research is required to fully understand the molecular mechanisms of the process |
|
Enhances product purity reducing downstream processing load |
Higher OPEX when implemented for complex waste streams is expected to increase product cost |
|
Non-thermal and can reduce solvent/chemical requirement of extraction process) |
Implementation at industrial scale has been limited |
|
Reduces energy consumption, duration, and environmental impact of process |
Process parameters must be optimised to achieve best efficiency and yield increase at industrial scale (contributing to complexity) |
|
Safety/nutritional advantages
|
Safety/nutritional drawbacks
|
|
Demonstrated to preserves nutritional value, flavour, texture, and colour of product, reduces allergenicity, and can enhance product functionality |
Food/feed safety assurance requires further investigation of protein functionality alteration when waste exposed to PEF |
|
Can destroy pathogens in dairy wastewater |
|
Ultrasound assisted extraction (UAE) |
Process advantages
|
Process drawbacks
|
89, 90, 92, 95, 97, 102 and 104 |
|
Reduces overall energy consumption and environmental impact of process |
Probe-type reactor configuration improves efficiency, but is not simple to implement |
|
|
Water can be used as solvent with greater efficiency than MAE (and lowers required organic solvent if used) |
Pilot studies indicate very poor scaling with diminished increase in protein yield compared to lab-scale |
|
|
Simple applicability of bath-type reactor configuration |
Attenuation of ultrasound waves is an issue leading to operational losses |
|
|
Can be used in conjunction with other extraction techniques (e.g., enzymatic hydrolysis, solvent extraction) to significantly increase yield |
Efficiency is highly dependent on reactor configuration, power intensity, duration, and is specific to substrate factors |
|
|
Shortens extraction time |
High energy consumption |
|
|
Safety/nutritional advantages
|
Safety/nutritional drawbacks
|
|
|
Favourable amino acid profile for well-optimised processes |
Protracted sonication at high power can result in severe protein denaturation and loss of functionality/solubility |
|
|
Favourable protein functionality for well-optimised processes |
Amino acid and nutritional profile can be diminished for poorly optimised processes |
|
Hydrolysis technologies.
Hydrolysis technologies can be employed to produce both protein and carbohydrate hydrolysate from waste streams. Hydrolysis agents include acid, alkali, organic solvents, subcritical water, and enzymes. The advantages and drawbacks of these technologies with regards to process operation and product safety/nutrition are summarised in Table 3, and a critical evaluation of enzymatic hydrolysis is given in the following section.
Table 3 Hydrolysis technologies: advantages and drawbacks of process efficiency/operation and product safety/nutrition (OPEX = operating expenditure; CAPEX = capital expenditure)
Technology |
Hydrolysis technologies |
Ref. |
Acid/alkali hydrolysis |
Process advantages
|
Process drawbacks
|
108–110 and 200 |
Inexpensive compared to enzymatic hydrolysis |
Unpleasant flavour of product |
Can be integrated with assisted extraction techniques for increased yield |
Harsh chemical conditions and high operating temperatures |
Proteins highly soluble under these conditions |
Relatively low yield |
Safety/nutritional advantages
|
Safety/nutritional drawbacks
|
Harsh temperatures and pH sterilise the feedstock |
Acid: Destruction of tryptophan, asparagine, and glutamine (partial destruction of methionine and cysteine). Alkali: Destruction of majority of amino acids but tryptophan is retained |
Enzymatic hydrolysis |
Process advantages
|
Process drawbacks
|
90, 99, 109–113 and 115 |
Mild operating temperature and pH |
Longer operational time compared to acid-alkali hydrolysis due to low temperature operation |
Harsh chemicals replaced by biological catalysts (enzymes) |
Acid/alkali is added to maintain optimum pH |
Available research is relatively extensive |
Difficult to operate at industrial scale due to tight multiparameter control requirements and sensitivity of enzymes |
Alcalase has broad substrate specificity (can achieve high yields for variety of waste feedstocks) |
Impurities in the feedstock such as phytochemicals in food waste can act as enzyme inhibitors, reducing efficiency |
Hydrolysate has improved rheological (texture) and taste profile |
Hydrolysate can retain impurities from feedstock (polluted downstream effluent requires purification) |
Low environmental impact |
High OPEX (requires the use of expensive enzymes) |
Specificity of enzymes minimises undesirable side-reactions and enables control of the degree of hydrolysis |
Substrate specificity of individual enzymes – “enzyme cocktail” may be required to efficiently hydrolyse the waste feedstock containing complex array of proteins and other compounds |
Carbohydrases can be employed to hydrolyse pre-treated lignocellulosic biomass into platform sugars for microbial protein fermentation |
Pre-treatment of lignocellulosic biomass required to fractionate complex carbohydrates |
Genetic engineering can be used to broaden enzyme specificity and increase efficiency |
Genetic engineering to broaden enzyme specificity is restricted in many countries. |
Safety/nutritional advantages
|
Safety/nutritional drawbacks
|
Protein hydrolysate has higher solubility and smaller peptides with improved functionality and reduced allergenicity compared with whole protein extraction |
Heat inactivation step may impact physiochemical properties of hydrolysate |
No destruction of amino acids (protein quality retained) |
|
Food-grade enzymes available and commonly employed (e.g., alcalase) |
|
Subcritical water hydrolysis |
Process advantages
|
Process drawbacks
|
42, 99, 108 and 201–213 |
Expensive enzymes not required |
High temperatures and pressures required compared to enzymatic hydrolysis |
Addition of acid, alkali and organic solvents not required (but addition of sodium bicarbonate, NaOH and acetone modifiers has been shown to increase yield) |
If required, reducing temperature to preserve protein quality will lead to increased reaction times |
|
Presence of other compounds in the feed may impact process yields (may be necessary to remove them through pre-treatment) |
Concentrated CO2 and O2 gas to pressurise the atmosphere can increase amino acid yield and decrease reaction time |
High CAPEX due to expensive equipment |
|
Moisture extraction (e.g., evaporation) is required downstream to obtain purified protein |
Safety/nutritional advantages
|
Safety/nutritional drawbacks
|
Hydrolysates have demonstrated improved functionality and compared to enzymatic hydrolysis in some studies |
Health and nutritional effect of modifications to the side chains and the amino acid profile have not been evaluated |
Amino acid profile is usually not significantly impacted (process condition dependent) |
Addition of O2 gas can decrease functionality of hydrolysate due to amino acid alterations |
Enzymatic hydrolysis.
In contrast with chemical, solvent, and subcritical water hydrolysis, enzymatic hydrolysis is effective at low temperatures, pressures, and mild pH, reducing reactor capital cost, heat duty and preservation of amino acid profile and other nutrients.87,108–110 Furthermore, favourable functional characteristics and improvements in safety and palatability for consumption (e.g., reduced allergenicity of smaller peptides, better digestibility, colour, and texture) have been observed in hydrolysates.87,111–113 However, enzymes remain expensive due to their production complexity and are highly sensitive to operating conditions (which must be tightly controlled) due to low stability, presenting a significant economic and complexity barrier to commercial implementation.87,99,114
Furthermore, enzymes generally have high substrate specificity, requiring careful consideration of enzyme choice for a given waste stream or potential use of a “cocktail” solution incorporating various enzyme types to broaden specificity, increasing process cost and complexity further.90 Enzymatic hydrolysis using proteases can be applied to waste feedstock directly to recover protein, however this can cause issues of enzyme inhibition by impurities in the feedstock. One way to address this is to apply enzymatic hydrolysis to high purity isolate recovered from upstream protein extraction processes.87,110,113,115
Carbohydrase enzymes can also be employed to produce sugar hydrolysate from organic waste containing complex carbohydrates by breaking down cell wall components (e.g. lignocellulosic cell walls) into constituent monomers, in addition to releasing dissolved proteins and reducing sugars from the intracellular matrix.116 Research into enzymatic hydrolysis of lignocellulosic waste has been detailed in previous reviews.2,114,117,118 In brief, according to Modenbach and Nokes (2013) cellulases and xylanases are the most commonly adopted enzymes to degrade cellulose and xylan, respectively.117 The degradation mechanisms of these enzymes on their corresponding carbohydrate substrates are discussed by Aditiya et al., (2016). In addition to the common sugars (e.g. sucrose, glucose, fructose, galactose, mannose, ribose, xylose, and arabinose), which occur in nature in the free form, or as monomers of oligosaccharides and polysaccharides, other rare monosaccharides and sugar alcohols (e.g. xylitol, mannitol, erythritol as sugar substitutes) can also be produced by enzyme-catalysed reactions.119 The wide range of platform chemicals, in particular the fermentable sugars, provide substrates to produce microbial protein or alternative protein sources. The capacity of microbial protein produced from such resources to replace conventional protein from animal husbandry was estimated by Pikaar and colleagues.23 The authors calculated that in terms of amino acid requirements, up to 10–19% of current global feed crops (occupying 6% of global arable area and equivalent to the entire current cropland of China) could be replaced by microbial protein, freeing up arable land area for other important agricultural practices.
However, with regards to lignocellulosic waste in particular, pre-treatments are required to fractionate complex carbohydrates from the biomass to increase substrate degradability and downstream process performance. Fractionation pre-treatment technologies include chemical (e.g. alkali, acid, ionic liquid), thermal (e.g. steam), biological (e.g. ligninolytic microbes) and physical (e.g. extrusion) methods, individually or in combination. Extensive research has focused on pre-treatment technologies, as detailed in several reviews.120–125 In short, these reviews conclude that the chemical processes successfully render effective fractionation but introduce design challenges such as solvent recycling and the need for reactor anti-corrosion steps. Physical and thermal routes may lead to cost-effective, solvent-free but energy-intensive solutions. Despite the advantages of low-energy demand and effective lignin depolymerisation, biological routes might be challenged by low reaction rate and inhibitor generation issues. Furthermore, food-safe methods of pre-treatment that are capable of high efficiency fractionation requires further research and development to improve economic viability and food/feed safety assurances for the downstream production chain.
The variety of technology options available offers great potential for novel protein solutions capable of transforming global food production as we know it. For example, Indonesia primarily relies on imported feed-protein such as soybean meal, fish meal and meat bone meal from America and Brazil, exposing the country to feed shortages in the event of global supply chain disruptions.126 Recognising this, Indonesian researchers have focused on protein recovery from local palm and coconut oil waste using microbial enzymes.127 Transitioning to local waste-to-protein solutions has the potential to significantly improve protein security and sustainability, while reducing the cost of meeting regional and national nutritional demands.
Bioconverter technologies.
Bioconverter technologies refer specifically to the use of value-upgrading organisms through metabolism of waste protein, nutrients, and waste-derived sugars into biomass, namely microbes and insects. The advantages and drawbacks of these technologies with regards to process operation and product safety/nutrition are summarised in Table 4 and 5.
Table 4 Bioconverter technologies (microbial protein): advantages and drawbacks of process efficiency/operation and product safety/nutrition (OPEX = operating expenditure; CAPEX = capital expenditure; R&D = research and development)
Technology |
Bioconverter technologies |
Ref. |
Microbial protein |
Process advantages
|
Process drawbacks
|
99, 174 and 214–232 |
Intracellular proteases eliminate the requirement of costly cell-free enzymes as in enzymatic hydrolysis |
Nutrient assimilation efficiency and selectivity is highly dependent on microbial strain |
|
Wastewater stream may require further processing to reduce nutrient content to acceptable limits |
Rapid (exponential) growth rates – high productivity compared to traditional protein sources |
High R&D cost for microbial strain screening, improvement, and process design/scale-up |
|
Genetic engineering of microbial strains is restricted in many countries |
Microbial co-culture can increase conversion efficiency of complex waste resources, autotrophic bacteria as potential carbon capture solution |
Sterility of cultivation broth is required and is difficult to achieve |
|
Difficulty of scale-up for continuous industrial processes |
Relatively large availability of research and expertise |
High CAPEX of process equipment |
|
Shear stress from agitation and aeration can negatively impact growth efficiency of microbes and product texture |
Reduced environmental impact due to lower water/energy consumption and no arable land requirement compared to traditional farming |
Strain evolution occurring over lengthy production times can result in dominant strain with undesirable phenotype (e.g., lower protein content, poor texture, protein functionality). |
|
Considerable downtime between batches (required even for continuous processes to reduce contamination risk and ensure product quality) |
Growth is season/climate-independent so can be operated year-round |
Requires tight control of many process parameters for maximum efficiency, increasing operation complexity |
|
High cost of product relative to traditional food/feed protein resources |
Microorganisms are relatively easy to genetically modify |
Batch variability of feedstock impacts process performance |
|
Inhibitory and unfermentable compounds in feedstock can negatively impact process performance (requires upstream purification) |
Safety/nutritional advantages
|
Safety/nutritional drawbacks
|
High protein content compared to many traditional sources of protein |
Novel feed/food protein and regulatory approval process can be time and resource intensive (use of waste feedstock may further complicate this) |
|
Low consumer acceptance as food source in some global regions |
Long history of use as human food protein source in many global regions |
Requires downstream processing to remove intracellular compounds unsafe for consumption (particularly nucleic acid, which causes severe gastrointestinal and other health problems) |
|
Strains may produce toxic compounds under certain conditions (e.g., mycotoxins) |
Favourable nutrient profiles including vitamins, minerals and no cholesterol compared to traditional sources of protein |
Allergic reactions to consumption have been reported for several microbial strains |
|
Long feeding trials required to assess toxicological and carcinogenic potential of product |
Animal feed replacement has demonstrated favourable digestibility and prolonged survival of animals due to probiotic contents (of yeast) |
Composition and quality are highly dependent on feedstock content. Can be difficult to ensure safety and consistent nutritional profile due to batch variability in waste feedstock |
|
Some microbes (especially bacteria) have poor palatability and colour, making them unsuitable for human food purposes |
Cell walls contribute to significant fibre content, thus potentially improving gut function and metabolism |
High risk of contamination during production and processing |
|
Feedstock must be/derived from food/feed-safe resource to avoid introduction of toxic contaminants |
Table 5 Bioconverter technologies (insects): advantages and drawbacks of process efficiency/operation and product safety/nutrition
Technology |
Bioconverter technologies |
Ref. |
Insects |
Process advantages
|
Process drawbacks
|
162–164, 166–169, 233 and 234 |
Can be further processed downstream (e.g., enzymatic hydrolysis/precipitation) to produce protein hydrolysate/isolate with favourable functionality |
Processing techniques are currently poorly optimised and regulated at scale |
Low energy consumption and environmental impact |
Processing methods (e.g., boiling, drying, freezing) can reduce lipid and protein yield and quality |
Can be formed using 3D printing to improve texture & appearance |
|
Insects are fast-growing (high productivity) |
Efficiency is sensitive to pH, oxygen, light, and temperature conditions |
Low temperatures required during cultivation |
Sanitary environment is difficult to achieve during cultivation, processing, storage |
Safety/nutritional advantages
|
Safety/nutritional drawbacks
|
Demonstrated to increase protein content, improve amino acid, nutrient profile and digestibility when insect powder used as a food additive |
Use as food additive can negatively affect the colour and palatability of the product |
No significant difference in allergenicity of insects and traditional food sources |
Spore-generating bacteria species can survive microbiocidal processing, increasing risk of potential food-borne diseases |
|
May accumulate harmful chemicals such as persistent organic pollutants (POPs) from feedstock if not food-safe |
Chitin and/or chitosan contribute to high fibre content (has been reported to reduce cholesterol and improve gastrointestinal function) |
Insect biomass has a high fat content (boiling process may be necessary) |
Long history of use as human food protein source in many global regions |
Flame retardants and plasticisers used in processing may accumulate in insect biomass |
Microbiocidal processing step (e.g., boiling) can be used to eliminate pathogens |
Cooking stage can damage nutrient profile and reduce protein content |
Low moisture content can improve texture when used as food additive |
Toxicological and carcinogenic identification is difficult due to high biodiversity of insect species |
Insect powders have demonstrated favourable mineral, fatty acids, and vitamin profiles |
Microbial contamination of insect communities is significant (novel high-pressure microbiocidal techniques should be explored to eliminate spore-forming bacteria while retaining functional and sensory properties) |
Favourable gel-forming ability, emulsion capacity, and water/oil absorption ability |
Allergic reactions in humans have been reported due to high chitin, and uric acid content of insects |
Has demonstrated decreased leaching of nutrients from animal feed when used as additive |
Very low consumer acceptance as food source, especially in developed regions |
Microbial protein
Microbial protein technology utilises yeast, fungal, bacterial, or algal strains capable of converting sources of carbon, nitrogen, and oxygen into protein-rich biomass fit for human consumption or animal feeding. Approximately 80 different microbial strains have been reported to enable the production of food-grade or feed-grade protein (Fig. 3).
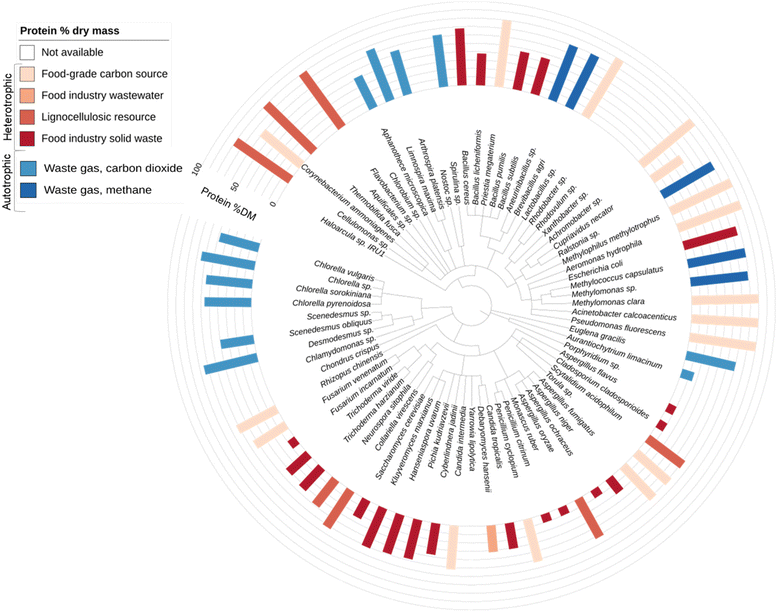 |
| Fig. 3 Taxonomic tree of reported microbial protein producing species. Species are sorted according to the National Centre for Biotechnology Information (NCBI) taxonomy database.132 Species are grouped by domain: Archaea, Eukaryota or Bacteria. Reported protein contents (% dry mass) are indicated by bar chart ranging from 10% to 80% dry mass (ESI Table ST3†). Where multiple protein values have been reported an average was calculated. Food-grade carbon source refers to pure food-grade soluble compounds such as glucose, lactose and maltose. Detailed data can be found in the ESI-3 and ESI Table ST3.† | |
Microalgae and bacteria.
Microalgae and bacteria represent the most protein-rich sources, within the range of 60–70 wt% and 50–80 wt%, respectively, whereas fungi/yeasts contain approximately 30–50 wt% protein, followed by protists at 10–20 wt%.128 The high protein content positions bacteria as a desirable candidate for microbial protein conversion. However, reported palatability issues are yet to be addressed, posing a challenge to the successful commercialisation of bacterial protein as a food product.129,130
Fungi.
Fungi (including yeast) have a longstanding history of use in the production of microbial protein food products, some of which are now mass-produced and widely distributed e.g. tempeh. Oncom, a traditional food closely related to tempeh and consumed mainly in West Java, Indonesia, is produced by fermenting Rhizopus oligosporus and Neurospora sitophila. Interestingly, waste by-products from food production such as soy pulp, peanut press cake and cassava tailings are typically employed as substrates for the fermentation process. Despite serving as a historical waste-to-protein proof of concept, a high quality, mass-produced oncom product has not yet been realised, and very few research efforts have been made to this end.131
Industrial production of microbial protein.
As early as the 1970s, a variety of high-quality upgrade products that are rich in microbial protein were established on farm and industrial scales, e.g. volatile fatty acids from Candida yeast133,134 and methanol to Pruteen.135 Despite relative ease of operation and access to a large body of expertise built by long-established fermentation industries, established supply chains (e.g. soybean-based protein) held an economically competitive edge, stifling many early businesses.
Mycoprotein has become one of the most successful food-grade microbial proteins and was originally produced in response to concerns regarding the insufficiency of meat as a sustainable and healthy protein source. It has been commercialised since 1985 as Quorn™
136 and is currently sold in 17 countries, predominantly in Europe but also in developing nations such as the Philippines, and is the largest microbial protein meat alternative (by sales) with over 6 billion meals supplied globally in 2020.137 Quorn™ mycoprotein is produced via fermentation of fungus species Fusarium venenatum A3/5 utilising glucose as feedstock, with the addition of oxygen, nitrogen, vitamins, and minerals.138 Mycoprotein has a moderate protein content (45% of biomass) and contains all essential amino acids (44% of total protein).139 Additionally, it offers positive health attributes compared with animal protein, such as a favourable fatty acid profile and high fibre content.140
These properties make Quorn™ mycoprotein well-suited to regions with high prevalence rates of obesity-related diseases such as North America and Europe.1,141 A series of recent studies in human physiology by Monteyne et al. (2020) have examined the capacity for mycoprotein to regulate skeletal muscle protein metabolism in young and older adults, with encouraging results.142
Other industrial pioneers have utilised microbial protein technologies to produce protein for human consumption, as well as for animal and aquaculture feed purposes. Notable feed-grade protein products that have been commercialised include All-G Rich® (Alltech), UniProtein® (Unibio) and Feedkind® (Calysta).143–145 Fungal species Neurospora sitophila also has a longstanding history of involvement in food production.146 White Dog Labs, Inc. (New Castle, Delaware) actively produces microbial protein for animal feed but has not disclosed strain information. Moreover, the carbon transformation company Kiverdi, Inc. (Pleasanton, California) recently introduced ‘Air Protein™’, which converts CO2 to food-grade protein by microbial fermentation; however, no detailed information has been disclosed on the hydrogenotrophic microorganisms used.147 Solar Foods (Helsinki, Finland) also produces food-grade microbial protein (Solein®) via CO2 fermentation at pilot scale and has recently been awarded €35 million in funding. Avecom (Ghent, Belgium) aims to integrate their microbial protein technology with existing food processing businesses as a waste recovery solution, allowing them to produce proteins for food or feed purposes. Furthermore, Avecom's ‘Power-to-Protein’ research partnership has been investigating renewable hydrogen and atmospheric CO2 as drivers for autotrophic and mixotrophic upgrading of nitrogen from waste to produce feed protein.120 However, issues of poor hydrogen mass transfer are still being addressed to ensure adequate rates of production. Phototrophic bacteria are also being explored to produce human food and animal feed from secondary resources.
Research and development of microbial protein.
Many microorganisms are still at the research and development stage. Microbial protein production that utilises lignocellulosic waste resources have generated increasing research attention. Two potential technology solutions have been reported, namely Fusarium venenatum A3/5 fed on glucose and xylose derived from lignocellulosic biomass11 and cellulose-consuming strains such as Aspergillus niger, Neurospore sitaphila, and Trichoderma viridae.121,122 Recently, SylPro® Arbiom has gained attention for scaling up trials of protein production based on the conversion of lignocellulosic forestry waste by yeast species for aquaculture feed.123,128 Producing novel food ingredients with desirable techno-functional and sensory qualities for use in the food and drink industry remains a formidable challenge,124 and the development of microbial protein ingredients is no exception.120 Currently, the preferred strategy is to focus on the nutritional value (amino acid composition) of microbial proteins and then search for smart combinations with other food ingredients to provide properties such as taste, texture and structure to the final food, such is the case with current mycoprotein products.125
Regulation and safety of microbial protein.
Although there is a large list of potential upgraders, the legislator formulates strict requirements regarding which organisms are accepted as human food. In the European Union, applications for novel food status require preparation of detailed technical dossiers as evidence for the safety of products. When added to the considerable costs and complexity involved in the application procedure, this creates an significant barrier to the development and commercialisation of novel foods148 in the EU and in countries adopting a similarly ‘cautionary’ regulatory model. With regards to the safety of microbial protein, it is imperative that toxicological testing is performed continuously for the detection of secondary metabolites (e.g. mycotoxins) as a food/feed safety assurance protocol.149 Furthermore, food processing operation must be performed under controlled conditions to mitigate the risk of contamination of the microbial culture, which could lead to the introduction of pathogens. Although this can prove difficult to achieve for industrial scale processing, good operator training, oversight and development of sound operation and testing procedures should be employed to address this issue. Furthermore, allergenicity and digestibility issues have been reported for several microbial strains, for example, cases of allergic reactions have been reported as a result of mycoprotein consumption.150 Therefore, extensive animal feeding trials are required prior to distribution to fully characterise the potential health risks posed by consumption of a particular microbial strain.
Insects
Contamination-free biowaste provides a theoretical feed stream for insects to act as waste-to-protein bio-converters. High conversion rates for Orthoptera sp. (1.7 kg feed
:
1 kg liveweight)65 and Hermentia illucens, i.e. black soldier fly larvae (1.95–13.42% carbon and 5.4–18.93% nitrogen recycling) have been reported.151 The cultivated insects can be harvested and converted into human food through relatively simple processing methods. For example, caterpillars and mealworms are prepared by scalding, drying and cooking (i.e. roasting or boiling), and insect protein bars are prepared by milling and processing (i.e. baking).20,152 According to recent estimates, one billion of the world's population are estimated to rely on insects as a primary protein source, particularly in African, South American and South East Asian countries.153 Insect-based foods are seeing increasing global acceptance in recent years, with the combined insect market of the US, Belgium, France, UK, the Netherlands, China, Thailand, Vietnam, Brazil and Mexico, predicted to increase from £25 million in 2015 to £398 million in 2023.154
Insect protein nutritional value analysis
Most insects are rich in protein and other nutrients such as iron and vitamin A.155 Oibiopka et al., (2018) found that the protein content of a diet consisting of Orthoptera, Lepidoptera and Blattodea fed to rats exhibits a 12–20% higher biological value compared to the standard protein casein.156 Moreover, in vitro digestion experiments evaluating mineral bioavailability indicated that Orthoptera sp. and Tenebrio molitor contain significantly higher chemically available calcium, magnesium, manganese, and zinc than sirloin beef.157
Fig. 4 shows the amino acid profiles of different food-grade benchmark animal-based, plant-based, and microbial proteins, as well as waste-to-protein insect and microbial protein sources. Compared to food-grade benchmark protein sources, waste-to-protein insect and microbial sources are richer in the essential amino acids.158 Waste-to-protein Fusarium spp. demonstrated the highest total essential amino acid contents of all protein sources, followed by food-grade egg and Quorn™ mycoprotein products, while Diptera sp. (including Hermetia illucens) protein exhibited a similar profile of essential amino acids to egg. Amongst insect proteins, Diptera sp. (including Hermetia illucens) and Coleoptera sp. (including Tenebrio molitor) appear to have the highest total amino acid contents (Fig. 4). However, the nutritional quality of edible insect protein could diminish during digestion due to low content of the limiting essential amino acids, tryptophan and lysine.65 Previous research also reported that methionine and cysteine were limiting amino acids in Blattodea sp., whereas isoleucine was limiting in some Orthoptera sp.159
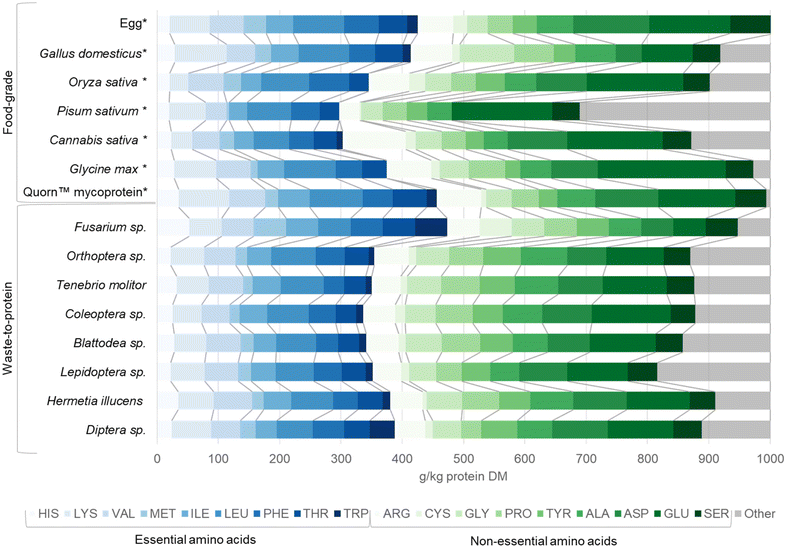 |
| Fig. 4 Amino acid profile of various microbial and insect protein sources. Egg albumin is included as a standard for comparison. Eighteen amino acids are included: Histidine (HIS), Lysine (LYS), Methionine (MET), Isoleucine (ILE), Leucine (LEU), Phenylalanine (PHE), Threonine (THR), Tryptophan (TRP), Arginine (ARG), Cysteine (CYS), Glycine (GLY), Proline (PRO), Tyrosine (TYR), Alanine (ALA), Aspartic acid (ASP), Glutamic acid (GLU) and Serine (SER). We were unable to obtain values for asparagine and glutamine. Amino acid profiles are displayed for waste-to-protein protein sources including: Fusarium sp. (mycoprotein), Orthoptera sp. (crickets, grasshoppers, locusts), Tenebrio molitor (mealworm) Coleoptera sp. (beetles), Blattodea sp. (cockroaches, termites), Lepidoptera sp. (butterflies, moths), Hermetia illucens (black soldier fly larvae) and Diptera sp. Bench mark food-grade* protein sources were provided for comparison including Gallus domesticus (chicken), Oryza sativa (Asian rice), Pisum sativum (pea), Cannabis sativa (hempseed), Glycine max (soya), and Quorn™ mycoprotein. Essential amino acid profiles are shown in blue, non-essential amino acids are shown in green on a g kg−1 protein dry mass basis. ‘Other’ is presented in grey and represents missing values or errors due to methodology limitations reported in original literature. Detailed data can be found in ESI-4 and ESI Table ST4.† | |
Accounting for the time taken for insects to reach maturity, Hermetia illucens and Tenebrio molitor larvae may be considered favourable new protein sources for rapid technology scale-up due to their relatively short lifecycles (ESI-6†). Depending on the grade of organic waste used as substrate, insect farming technologies provide a source of protein for human consumption or animal feed purposes. As efficient waste-to-protein bio-converters, insects achieve high conversion efficiency to turn low-grade waste into protein sources. For example, 100 g of Hermetia illucens prepupae fed on food waste produced 80–85 g of pressed cake with a high protein content of 53.1%.160 There is a growing number of institutions and programmes dedicated to researching insect farming as a means to address increasing global feed demands, including the International Centre of Insect Physiology and Ecology, the Sanergy project in Kenya, the Entofood partnership with Veolia in Malaysia, and Innovafeed in France (ESI Table ST6.4†). Introducing insects such as Hermetia illucens as protein feed substitutes for livestock and aquaculture could bring significant socio-economic benefits such as job creation and circular economy opportunities.
Safety of insect protein
With regards to safety and quality assurance of insects for food and feed purposes, a major complicating issue is with regards to the high content of insect-borne microbes, that if not treated effectively, can be a source of food/feed-borne disease.161 Commonly employed microbiocidal techniques such as commercial and domestic cooking (e.g. boiling) are effective as destroying the microbiome post-harvesting, and subsequent drying and refrigeration can be employed to maintain sterility.162 However, due to the large biodiversity of insects and consequently large variability in microbiome composition, safety assurance can become more complicated. For example, spore-forming bacterial species such as Bacillus sp. and Clostridia can effectively survive traditional microbiocidal techniques.163–165 It is therefore imperative that pathogen testing is performed pre- and post-processing as part of a food and feed safety assurance protocol, yet this remains a challenging endeavour.166 The application of new microbiocidal techniques such as high-pressure processing should be investigated at scale, which have demonstrated greater effectiveness at eliminating spore-forming bacteria while mitigating negative impacts on functional and sensory properties of insects that occur during high temperature processing.43 Another safety concern is allergenicity and digestibility of consumed insects. Post-processing using protein extraction and hydrolysis techniques may be required to reduce risks of adverse reactions to compounds found in whole insects such as high chitin and uric acid content.90,109,149,167 As insect processing is relatively poorly optimised for efficiency and safety, greater research focus is required to improve the efficacy of commercialisation of insect protein globally, and to improve consumer and regulatory acceptance rates.166,168–171
Waste-to-protein system
A waste-to-protein system has the potential to converge waste-recovery and protein security towards a resource-circular protein future. To date, waste-to-protein technologies have been safely developed and scaled-up including the food-waste derived insect protein as animal feed (e.g. Entofood and Livalta technologies) and waste-gas to microbial protein as aquafeed (e.g. Deep Branch gas fermentation technology). Under the waste-to-protein vision, we propose to synergistically integrate biotechnologies to maximise the recovery of food or feed-grade protein from contaminant-free organic waste while systematically considering regional characteristics on a global scale. This initiative would consider waste resource abundance and composition as well as existing industries and waste recovery infrastructure. Specifically, there is a need to develop and introduce efficient logistical approaches of supply and demand in cooperation with regulators and feed/food safety authorities.
Bioprocess analysis
Fig. 5 displays a range of chemical, physical, and biological processes that can be applied to extract protein and nutrients directly from waste, or to convert waste-carbon to sugars or other platform chemicals for subsequent protein production. As presented in Fig. 6, considerable amounts of food/feed grade waste are generated globally every year, including feed-grade OFMSW, lignocellulosic waste from agriculture and forestry sectors, and waste streams from the food and drink industry. Our estimated protein recovery potential was based on conversion rates (ESI Table ST5.3†) of different technologies reported to be food- or feed-grade. With highly efficient insect bio-converters, it is estimated that 68 to 135 megatonnes per year of insect proteins could be recovered from carbon-rich OFMSW waste, depending on the insect species employed. Microbial protein technologies represent an effective lignocellulosic carbon-to-protein conversion pathway, offering protein recovery in the range of 562 megatonnes per year using food grade F. venenatum, or up to 1352 megatonnes per year using feed-grade K. marxianus species. The estimated protein recovery potential from global food and drink industry waste (135 megatonnes per year) ranges between 15 to 22 megatonnes per year. However, our estimated recovery value focuses on F. venenatum due to its history as an widely-accepted food source.136 This pathway offers a potential 562 megatonnes per year recovery of food protein from the 11
108 megatonnes per year cellulosic waste content produced by global agricultural and forestry sectors, supplying 72 g per capita per year (197 g per capita per day) waste-derived protein to meet the average adult daily protein recommendation (50 g per 70 kg).172 However, as these estimates were based on conversion rates derived from literature data, further characterisation of region-specific waste composition and exploratory research on resource recovery potential at scale are essential to provide evidence for informed decision-making.
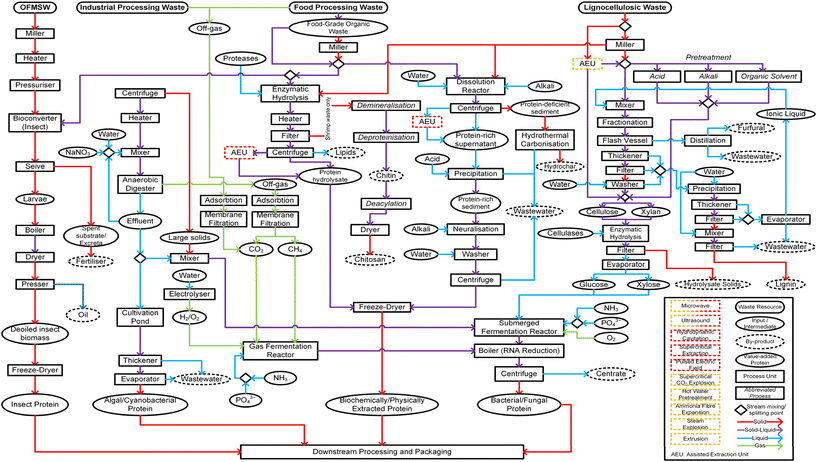 |
| Fig. 5 Waste-to-protein system. Process flow diagram demonstrating potential pathways for protein valorisation from organic fraction of municipal solid waste (OFMSW); industrial processing waste; food processing waste; and lignocellulosic waste to obtain value-added protein. Nodes: rectangle (rounded) = waste resource; oval (thin border) = input/intermediate; oval (dashed border) = by-product; oval (thick border) = value-added protein; rectangle = process unit; rectangle (italicised font) = abbreviated process; diamond = stream mixing/splitting point. Stream arrows: red = solid phase; purple = solid–liquid mixture; blue = liquid phase; green = gas phase. Assisted extraction unit (AEU) (red-dashed) refers to any of the following: microwave; ultrasound; supercritical extraction; pulsed electric field. AEU (orange-dashed) refers to any of the following: microwave; ultrasound; supercritical CO2 explosion; hot water pre-treatment; ammonia fibre expansion; steam explosion; extrusion.18,70,90,166,235–245 | |
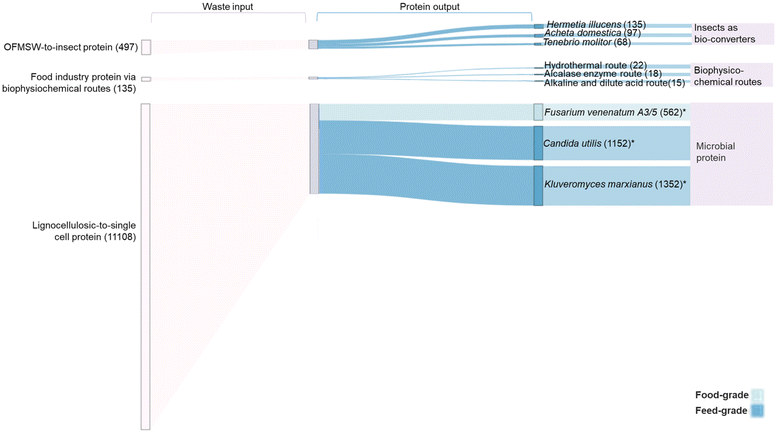 |
| Fig. 6 Quantitative mass balance for a theoretical waste-to-protein system. Input waste streams are shown on the left: OFMSW-to-insect protein, agricultural lignocellulosic-to-microbial protein, and food industry (including fishing, aquaculture and brewery industry) protein via biophysicochemical routes. The protein outputs are shown on the right. *Candida Utilis and Kluveromyces marxianus are capable of utilising hexose and pentose sugars. Values given are for glucose utilisation only. Inclusion of pentose sugars increases conversion outputs to an upper range of 893 megatonne per year, 1831 megatonne per year and 2149 megatonne per year for Fusarium venenatum A3/5, Candida utlilis, and Kluveromyces marxianus, respectively. All values in brackets are given in megatonne per year. Detailed data can be found in ESI-5 and ESI Table ST5.† | |
Geographical analysis
It should be noted that both waste compositions (Fig. 1 and 2) and existing waste recovery systems differ significantly across countries. Developed and urbanised regions tend to produce higher quantities of MSW with a lower organic component than low-income countries and offer established centralised waste collection and treatment infrastructure. Thus, centralised waste-to-protein systems represent great potential for increased efficiency.15 In less developed countries, there are still large amounts of untapped waste resources including OFMSW, agricultural and forestry lignocellulosic waste, and food and drink industry waste that represent unexploited future potential for a waste-to-protein system.30 The more sporadic distribution of organic waste and lack of sustainable waste-recovery systems positions decentralised waste-to-protein solutions as the most suitable approach for such countries. Examples include those in recent studies focused on Hermetia illucens as bio-converters of food processing waste, and microbial protein routes developed by Deep Branch for aquafeed production from decentralised waste gas streams.173,174 The significant global variations discussed above call for a systems approach to synergistically integrate centralised and decentralised technologies and optimise waste-to-protein solutions, which consider the spatial distribution of regional waste and existing industries and infrastructures.
Consumer perception analysis and safety
Perceptions of a ‘waste-to-protein’ concept vary significantly by country and also warrant consideration. African and South East Asian countries appear to be good candidates for expansion of technologies that utilise insects as bio-converters due to their relatively strong cultural acceptance of insects as food.175 Microbial fermentation is already well-established in Europe and North America, with Quorn™ being a popular and mainstream food product in both regions. These regions would therefore be a good target for expanding microbial protein technologies. It is essential that upgraded ‘waste-to-protein’ products are regarded as high-quality and safe by consumers globally. As such, conversion and upgrading must proceed within the conditions set out by the feed/food chain alliance and must comply with hygiene quality and safety standards set by regulators176,177
Regulatory analysis
New protein sources have been highlighted as novel food, which need to meet general food safety requirements stipulated in national or regional food safety regulations.178 Global approaches to the regulation of novel food vary significantly. In the EU, Canada, Singapore, and India, evidence of ‘history of safe use’ (HOSU) is considered globally, whereas in China, Australia/New Zealand (AU/NZ) and Brazil, the scope of HOSU is restricted to native consumption.179–183 AU/NZ and Canada are exceptional in that there is no rigid cut-off date defined for HOSU, giving their respective regulatory authorities an extra degree of freedom in determining novel status.179 In these countries, if a protein for food purposes is deemed novel by the responsible authoritative body, a risk assessment is then undertaken considering evidence submitted in the form of a dossier by the manufacturer.179–183 Pre-submission consultations can help to identify missing information and errors in the dossier to avoid ‘clock-stop’ delays in the risk assessment stage. Food Standards Australia/New Zealand, Singapore Food Association and Health Canada have established organisations specifically for this purpose.179,180 In the US, novel status is commonly self-determined by the manufacturer in accordance with generally recognised as safe (GRAS) standards, through convening of an expert panel to review publicly available scientific data on the HOSU of their product.179 Alternatively, a food additive petition can be submitted to the Food and Drug Administration. However, data from in-house testing pertaining to safety of the product is required in this case, incurring similar issues of high cost and extended timelines from submission-to-market as in countries adopting an EU-style model.179,184 Further details on global novel food/protein regulations and notification processes can be found in ESI-7.†
Recent regulatory advances on waste-to-protein for animal feed purposes in the EU includes Regulation (EU) 2021/1372, an amendment that allows the use of insect-processed proteins as feed.185 Subsequently, Regulation (EU) 2021/1925 was implemented to authorise the use of Bombyx mori (silkworm) processed animal proteins in animal feed, the eighth insect species to be approved.186 It should be noted that regulatory discrepencies between feed and food safety assurance criteria for novel proteins exist. For example, the Singapore Food Agency requires that substances used to feed insects are “properly handled and traceable” to ensure the safety of insect-derived animal feed, but does not require that microbiocidal post-harvest treatment is performed to destroy pathogens, which is required when harvesting insects for human food purposes.187Furthermore, EU regulations allow the use of processed animal protein derived from eight species of insects for aquaculture, poultry, and pig feed purposes.188,189 However, substrates used for the cultivation of insects for human food purposes are restricted to those of vegetal origin, restricting the scope of waste-to-protein system with respect to the types of waste that can be valorised for a particular region and usage purpose.186,189,190
Future research and technology development
Insect proteins and microbial proteins offer environmental advantages over conventional animal-source or plant-sourced proteins, in particular on climate change mitigation and arable land use reduction (ESI-8 and ESI Table ST7†). However, novel protein research and technologies are still at the infant stage in contrast to conventional protein sources, which operate at higher technological readiness levels (TRL) 7–9. Thus, future research into waste-to-protein scale-up potential, particularly with regards to process integration and optimisation, is necessary to enable novel waste-to-protein products to become economically competitive.
Safety/nutritional assurance and process efficacy at each stage of the waste-to-protein system remains a complex and significant barrier to implementation. There are significant knowledge gaps regarding the efficacy of novel waste-to-protein technologies, particularly insects/microbial protein as bioconverters, subcritical water hydrolysis, and assisted extraction techniques (Table 1–4). Due to large compositional variety of waste feedstocks (and species of bioconverters), the effects of these processing methods on protein structures and subsequent functionality are not comprehensively understood. Hence, greater research efforts are required to formalise the underlying mechanisms of protein extraction for novel technologies. Additionally, feeding trials and rigorous testing should be performed for protein valorised from a wide range of waste feedstocks to assess the allergenicity, digestibility and toxicity potentials.
Furthermore, knowledge of the regional regulations regarding feed-safe and food-safe novel proteins should be a major consideration when defining key design specifications for industrial production, such as feedstock type and composition, protein valorisation technology flowsheet and operating conditions, as well as product functionality and purity. On the other hand, despite promising process efficiencies, protein yields and functionalities at lab-scale demonstrated by novel technologies, future work should also focus on pilot and/or industrial studies to evaluate scale-up effects on the process performance and product quality. Utilising this information to perform technoeconomic analyses and process optimisation is key to maximising the value of waste-derived protein while minimising environmental impacts and comporting with regional safety assurance standards.
Nevertheless, new protein sources have the potential to contribute towards food systems that operate within scientifically defined targets for sustainability, both at local and Earth system scales, i.e. planetary boundaries.246
Overall, it is not only conversion efficiency and nutritional quality of proteins recovered from waste that are of importance, but also the processability, scalability and acceptability of a waste-to-protein system that are highly relevant to future work. Thus, future research and technology development should focus on the waste resources and protein solutions that (i) offer food- or feed-grade nutrition values; (ii) are easily processed and harvested, and thereby able to fit into existing food supply chains; (iii) consider perception, safety and acceptability to the consumers and regulators; and (iv) advance the understanding of waste-to-protein technology performance, including process optimisation at scale, techno-economic viability, and environmental sustainability.
Conclusions and future work
Animal-sourced proteins are not only carbon-intensive and resource-demanding, but also vulnerable to pandemic effects (e.g. Covid-19) due to long-production cycles (except for chicken, ESI-6†) and animals being susceptible to infection. These factors, combined with increasing protein demands and the persistent global hunger pandemic, highlight the complex challenges of ensuring protein security for human health within environmental boundaries. In this quantitative analysis, we have proposed a waste-to-protein upgrading system. By synergistically integrating waste-to-protein technologies, this system has the potential to solve a significant component of the global challenge of a planet degrading food system and converge innovations on zero-waste and protein security towards a sustainable protein future. Our study emphasises the importance of upstream quality preservation by assuring contaminant-free organic waste streams and systems analysis to estimate the waste-to-protein potential involving chemical, physical, and biological conversion pathways. We quantified global waste streams, which are rich in carbon and nutrients and absent of pathogens and hazardous substances. These streams present a global annual resource potential of 497 megatonnes of OFMSW, 135 megatonnes of by-products from the brewing and shrimp fishing industries and 11
108 megatonnes of lignocellulosic agricultural and forestry waste. This is equivalent to 9386 megatonnes of holocellulosic contents, which can be converted to fermentable sugars amounting to 2503 megatonnes of glucose, or 3980 megatonnes of glucose and xylose.
Over 80 microbial species have been discovered to enable efficient waste recovery of microbial protein with preferable amino acid profiles that are characteristic of proteins of high biological value. A concerted effort to broaden the range of micro-organisms is warranted, either independently or in combination with microbiomes or designed cultures that can be regarded as safe for upgrading secondary resources to safe animal feed and foods. Insects as bio-converters offer efficient mechanisms to convert different grades of waste to food or feed proteins, which are generally rich in protein, vitamins, and minerals such as iron, calcium, manganese and zinc compared with other animal-sourced proteins.
Despite advances in individual technologies, critical gaps remain in the development of innovative systems which will enable ‘plug-and-play’ solutions, synergistic technology integration, and optimisation of the protein recovery from diverse waste streams. Although we demonstrate that waste-to-protein system has the potential to recover waste and catalyse novel protein solutions, scientific targets that define healthy and sustainable protein production remain absent. Integrated assessment and optimisation of waste-to-protein value chains that consider scientifically quantified planetary boundaries246 represent a future research frontier to further understand the implications of a waste-to-protein transition for water, land, biodiversity, carbon, nitrogen and phosphorus (5 of the 9 planetary boundaries). Notably, evidence-informed regulatory response timelines are considerably lagging behind the accelerated food and feed technology innovations including novel proteins. For waste-to-protein, many aspects remain unknown, such as the quality of low-value waste streams, nutritional values and health effects. Such regulatory barriers hinder the development of waste-to-protein technologies.
Future research to enable deep scanning of the fast-paced protein innovation landscape and develop a system for rapid regulatory response is needed. A sustainable protein system can only be achieved by multi-sector, multi-level actions that include a substantial global shift towards reduction in food loss and waste, and deployment of innovative protein technologies. Under the international policy framework, human health and environmental sustainability are included in most of the United Nations Sustainable Development Goals (SDGs).247 Integrated analyses of different future diet and protein scenarios and their impacts on humans (SDGs 1 and 2) and on planetary boundaries (SDGs 6, 13, 14, 15, on water, climate, ocean, and biodiversity) are necessary to inform future policy and technology development. A crucial element is the linkage of the waste-to-protein supply chains, environment footprint and the overall regulatory measures in relation to the sustainability and safety of upgrade-protein to help ameliorate the persistent and ongoing hunger pandemic and to protect the planet.
Author contributions
M.G., G.K., A.K. and H.F.R. designed the study. E.P., M.B., Y.H. and M.G. performed research and data analyses. E.P., W.V., P.E., M.B. and M.G. drafted the manuscript sections. J.R., P.S., O.W., C.H., J.H. and M.B. contributed significantly to the paper revision. A.L. contributed to the data visualisation. All authors contributed to the final paper revision and approved the final manuscript.
Conflicts of interest
There are no conflicts to declare.
Acknowledgements
G. K., A. K., H. F. R. and M. G. would like to acknowledge the UK Royal Academy of Engineering for Frontiers of Engineering for Development Seed funding. E. P. and M. G. would like to acknowledge the UK Engineering and Physical Sciences Research Council (EPSRC) and Monde Nissin Corporate for providing financial support under EPSRC iCASE programme. E. P. and M. G. would like to acknowledge Thomas Upcraft for providing data on lignocellulosic mycoprotein technologies.
References
- FAO, IFAD, UNICEF, WFP and WHO, FAO, 2020.
- P. W. Gerbens-Leenes, M. M. Mekonnen and A. Y. Hoekstra, Water Resour. Ind., 2013, 1–2, 25–36 CrossRef.
- P. Alexander, C. Brown, A. Arneth, J. Finnigan, D. Moran and M. D. A. Rounsevell, Agric. Syst., 2017, 153, 190–200 CrossRef PubMed.
-
Food and Agriculture Organization, State of World Fisheries and Aquaculture, Rome, 2020, p. 200 Search PubMed.
- J. Poore and T. Nemecek, Science, 2018, 360, 987–992 CrossRef CAS PubMed.
- D. G. A. B. Oonincx, S. Van Broekhoven, A. Van Huis and J. J. A. Van Loon, PLoS One, 2015, 10, e0144601 CrossRef PubMed.
- T. Bhalla and M. Joshi, World J. Microbiol. Biotechnol., 1994, 10, 116–117 CrossRef CAS PubMed.
- M. Øverland and A. Skrede, J. Sci. Food Agric., 2017, 97, 733–742 CrossRef PubMed.
- J. Schmidhuber, FAO Food Outlook, 2020, 9, 1–169 Search PubMed.
- Y. A. Nasereldin, R. Brenya, A. P. Bassey, I. E. Ibrahim, F. Alnadari, M. M. Nasiru and Y. Ji, Open Journal of Business and Management, 2021, 9, 184 CrossRef.
- T. Upcraft, W.-C. Tu, R. Johnson, T. Finnigan, N. Van Hung, J. Hallett and M. Guo, Green Chem., 2021, 23(14), 5150–5165 RSC.
-
J. von Braun, B. Beyene Chichaibelu, M. Torero Cullen, D. Laborde and C. Smaller, Policy Brief by the Center for Development Research (ZEF) of Bonn, University with the Food and Agriculture Organization of the United Nations (FAO) and Ceres2030, Bonn, 2020 Search PubMed.
- V. Ramakrishnan, A. Ghaly, M. Brooks and S. Budge, Bioprocess. Biotech., 2013, 3, 2 Search PubMed.
- C. Wen, J. Zhang, Y. Duan, H. Zhang and H. Ma, J. Food Sci., 2019, 84, 3330–3340 CrossRef CAS PubMed.
-
S. Kaza, L. Yao, P. Bhada-Tata and F. Van Woerden, What a waste 2.0: a global snapshot of solid waste management to 2050, The World Bank, 2018 Search PubMed.
- T. Aggelopoulos, K. Katsieris, A. Bekatorou, A. Pandey, I. M. Banat and A. A. Koutinas, Food Chem., 2014, 145, 710–716 CrossRef CAS PubMed.
- E. Calzoni, A. Cesaretti, S. Tacchi, S. Caponi, R. M. Pellegrino, F. Luzi, F. Cottone, D. Fioretto, C. Emiliani and A. Di Michele, Catalysts, 2021, 11, 167 CrossRef CAS.
- S. Cappellozza, M. G. Leonardi, S. Savoldelli, D. Carminati, A. Rizzolo, G. Cortellino, G. Terova, E. Moretto, A. Badaile, G. Concheri, A. Saviane, D. Bruno, M. Bonelli, S. Caccia, M. Casartelli and G. Tettamanti, Animals, 2019, 9(5), 278 CrossRef PubMed.
-
W. Wrap, WRAP, London, 2018 Search PubMed.
- G. Melgar-Lalanne, A. J. Hernández-Álvarez and A. Salinas-Castro, Compr. Rev. Food Sci. Food Saf., 2019, 18, 1166–1191 CrossRef CAS PubMed.
- A. Mishra, J. N. Ntihuga, B. Molitor and L. T. Angenent, Joule, 2020, 4, 1142–1147 CrossRef.
- B. Molitor, A. Mishra and L. T. Angenent, Energy Environ. Sci., 2019, 12, 3515–3521 RSC.
- I. Pikaar, J. De Vrieze, K. Rabaey, M. Herrero, P. Smith and W. Verstraete, Sci. Total Environ., 2018, 644, 1525–1530 CrossRef CAS PubMed.
- J. De Vrieze, K. Verbeeck, I. Pikaar, J. Boere, A. Van Wijk, K. Rabaey and W. Verstraete, New Biotechnol., 2020, 55, 12–18 CrossRef CAS PubMed.
-
Fsa, Comparing international approaches to food safety regulation of GM and novel foods, Campden BRI (Chipping Campden) Ltd, 2021 Search PubMed.
- M. Carus and L. Dammer, Ind. Biotechnol., 2018, 14, 83–91 CrossRef.
- T. M. W. Mak, X. Xiong, D. C. W. Tsang, I. K. M. Yu and C. S. Poon, Bioresour. Technol., 2020, 297, 122497 CrossRef CAS PubMed.
- S. Sakai, S. Sawell, A. Chandler, T. Eighmy, D. Kosson, J. Vehlow, H. Van der Sloot, J. Hartlen and O. Hjelmar, Waste Manage., 1996, 16, 341–350 CrossRef CAS.
- M. E. Edjabou, M. B. Jensen, R. Götze, K. Pivnenko, C. Petersen, C. Scheutz and T. F. Astrup, Waste Manage., 2015, 36, 12–23 CrossRef PubMed.
-
D. Hoornweg and P. Bhada-Tata, Urban development series; knowledge papers no. 15, 2012 Search PubMed.
- G. L. Ooi, Curr. Opin. Environ. Sustainability, 2009, 1, 187–191 CrossRef.
- C. Zhao, L. Xin, X. Xu, Y. Qin and W.-X. Wu, J. Hazard. Mater., 2021, 424, 127526 CrossRef PubMed.
- M. Zalewska, A. Błażejewska, A. Czapko and M. Popowska, Front. Microbiol., 2021, 12, 610656 CrossRef PubMed.
- F. Baghal Asghari, M. H. Dehghani, R. Dehghanzadeh, D. Farajzadeh, D. Shanehbandi, A. H. Mahvi, K. Yaghmaeian and A. Rajabi, Sci. Rep., 2021, 11, 24519 CrossRef CAS PubMed.
- A. Cesaro and V. Belgiorno, Ultrason. Sonochem., 2013, 20, 931–936 CrossRef CAS PubMed.
- S. Lim, J. L. Shi, U. von Gunten and D. L. McCurry, Water Res., 2022, 213, 118053 CrossRef CAS PubMed.
- A. Tufail, W. E. Price and F. I. Hai, Chemosphere, 2020, 260, 127460 CrossRef CAS PubMed.
- N. Scarlat, V. Motola, J. F. Dallemand, F. Monforti-Ferrario and L. Mofor, Renewable Sustainable Energy Rev., 2015, 50, 1269–1286 CrossRef.
- K. Kawai and T. Tasaki, J. Mater. Cycles Waste Manage., 2016, 18, 1–13 CrossRef.
-
S. Esteves and D. Devlin, in Waste and Resources Action Programme, 2010, pp. 1–33 Search PubMed.
- I.-S. Woo, I.-K. Rhee and H.-D. Park, Appl. Environ. Microbiol., 2000, 66, 2243–2247 CrossRef CAS PubMed.
- H.-W. Huang, C.-P. Hsu, B. Yang and C.-Y. Wang, Compr. Rev. Food Sci. Food Saf., 2014, 13(1), 78–90 CrossRef PubMed.
- H.-W. Huang, S.-J. Wu, J.-K. Lu, Y.-T. Shyu and C.-Y. Wang, Food Control, 2017, 72, 1–8 CrossRef.
- K. Paritosh, M. Yadav, S. Mathur, V. Balan, W. Liao, N. Pareek and V. Vivekanand, Front. Energy Res., 2018, 6 DOI:10.3389/fenrg.2018.00075.
-
A. Koopmans and J. Koppejan, Regional consultation on modern applications of biomass energy, 1997, vol. 6, p. 10 Search PubMed.
- V. Daioglou, E. Stehfest, B. Wicke, A. Faaij and D. P. Van Vuuren, GCB Bioenergy, 2016, 8, 456–470 CrossRef.
- Phyllis2-Database for (treated) biomass, algae, feedstocks for biogas production and biochar.
-
FAO, IFAD, UNICEF, WFP and WHO, The State of Food Security and Nutrition in the World 2017. Building resilience for peace and food security, FAO, Rome, 2017 Search PubMed.
- M. Parikka, Biomass Bioenergy, 2004, 27, 613–620 CrossRef.
- R. J. Keenan, G. A. Reams, F. Achard, J. V. de Freitas, A. Grainger and E. Lindquist, For. Ecol. Manage., 2015, 352, 9–20 CrossRef.
-
P. Baruya, World forest and agricultural crop residue resources for cofiring, IEA Clean Coal Centre London, UK, 2015 Search PubMed.
- R. Liguori and V. Faraco, Bioresour. Technol., 2016, 215, 13–20 CrossRef CAS PubMed.
- S. V. Hanssen, V. Daioglou, Z. J. N. Steinmann, J. C. Doelman, D. P. Van Vuuren and M. A. J. Huijbregts, Nat. Clim. Change, 2020, 10, 1023–1029 CrossRef CAS.
- M. Herrero, A. Cifuentes and E. Ibañez, Food Chem., 2006, 98, 136–148 CrossRef CAS.
- M. N. Islam, Y. T. Jo, Y. J. Jeong and J. H. Park, Environ. Technol., 2019, 40, 125–131 CrossRef CAS PubMed.
-
H. Haller, G. Paladino, G. Dupaul, S. Gamage, B. Hadzhaoglu, S. Norström, A. Eivazi, S. Holm, E. Hedenström and A. Jonsson, Clean Technologies and Environmental Policy, 2021, DOI:10.1007/s10098-021-02147-3.
- M. Raventós, S. Duarte and R. Alarcón, Food Sci. Technol. Int., 2002, 8, 269–284 CrossRef.
- S. Gbashi, O. A. Adebo, L. Piater, N. E. Madala and P. B. Njobeh, Sep. Purif. Methods, 2017, 46, 21–34 CrossRef CAS.
-
J. Bumpus and G. M. Gadd, Fungi in Bioremediation, Cambridge University Press, Cambridge, 2001 Search PubMed.
- A. T. N. Dao, J. Vonck, T. K. S. Janssens, H. T. C. Dang, A. Brouwer and T. E. de Boer, Ind. Crops Prod., 2019, 128, 153–161 CrossRef CAS.
- H. Haller and A. Jonsson, Chemosphere, 2020, 254, 126826 CrossRef CAS PubMed.
- N. Dutta, M. Usman, G. Luo and S. Zhang, Sustainable Chem., 2022, 3, 35–55 CrossRef CAS.
- A. Gil, Chem. Eng. J. Adv., 2021, 8, 100186 CrossRef CAS.
- H. D. De Holanda and F. M. Netto, J. Food Sci., 2006, 71, C298–C303 CrossRef.
- A. Van Huis, Annu. Rev. Entomol., 2013, 58, 563–583 CrossRef CAS PubMed.
- M. Rinaudo, Prog. Polym. Sci., 2006, 31, 603–632 CrossRef CAS.
- K. M. Lynch, E. J. Steffen and E. K. Arendt, J. Inst. Brew., 2016, 122, 553–568 CrossRef CAS.
-
C. T. Brett and K. W. Waldron, Physiology and biochemistry of plant cell walls, Springer Science & Business Media, 1996 Search PubMed.
- R. Wahlström, K. Rommi, P. Willberg-Keyriläinen, D. Ercili-Cura, U. Holopainen-Mantila, J. Hiltunen, O. Mäkinen, H. Nygren, A. Mikkelson and L. Kuutti, ChemistrySelect, 2017, 2, 9355–9363 CrossRef.
- S. I. Mussatto, G. Dragone and I. C. Roberto, J. Cereal Sci., 2006, 43, 1–14 CrossRef CAS.
- A. Paz, D. Outeiriño, N. Pérez Guerra and J. M. Domínguez, Bioresour. Technol., 2019, 275, 402–409 CrossRef CAS PubMed.
- A. Bianco, M. Budroni, S. Zara, I. Mannazzu, F. Fancello and G. Zara, Appl. Microbiol. Biotechnol., 2020, 104, 8661–8678 CrossRef CAS PubMed.
- B. Socas-Rodríguez, G. Álvarez-Rivera, A. Valdés, E. Ibáñez and A. Cifuentes, Trends Food Sci. Technol., 2021, 114, 133–147 CrossRef.
- M. Giavoni, M. J. Villanueva-Suárez, R. De la Peña-Armada, A. Garcia-Alonso and I. Mateos-Aparicio, Foods, 2022, 11(13), 1973 CrossRef CAS PubMed.
-
C. M. Galanakis, Food waste recovery: processing technologies and industrial techniques, Academic Press, 2015 Search PubMed.
- N. A. Sagar, S. Pareek, S. Sharma, E. M. Yahia and M. G. Lobo, Compr. Rev. Food Sci. Food Saf., 2018, 17, 512–531 CrossRef CAS PubMed.
- M. Reig, X. Vecino and J. L. Cortina, Foods, 2021, 10(11), 2768 CrossRef PubMed.
- B. Das, S. Sarkar, A. Sarkar, S. Bhattacharjee and C. Bhattacharjee, Process Saf. Environ. Prot., 2015, 101, 27–33 CrossRef.
- K. Shahid, V. Srivastava and M. Sillanpää, Environ. Sci. Pollut. Res., 2021, 28, 10262–10282 CrossRef CAS PubMed.
-
Marlow Foods Limited, UK Pat., 79109925, 2002 Search PubMed.
- S. Taskila, M. Ahokas, J. Järvinen, J. Toivanen and J. P. Tanskanen, Scientifica, 2017, 2017, 5120947 CrossRef PubMed.
- Z. Xu, N. Hao, L. Li, Y. Zhang, L. Yu, L. Jiang and X. Sui, ACS Sustainable Chem. Eng., 2019, 7, 15504–15513 CrossRef CAS.
- T. Alvi, Z. Asif and M. K. Iqbal Khan, Food Biosci., 2022, 46, 101580 CrossRef CAS.
- F. Arrutia, M. Adam, M. Á. Calvo-Carrascal, Y. Mao and E. Binner, Chem. Eng. J., 2020, 395, 125056 CrossRef CAS.
- L. Buchmann and A. Mathys, Front. Bioeng. Biotechnol., 2019, 7, 265 CrossRef PubMed.
- R. Buckow, P. S. Chandry, S. Y. Ng, C. M. McAuley and B. G. Swanson, Int. Dairy J., 2014, 34, 199–212 CrossRef CAS.
- G. Fadimu, T. Le, H. Gill, A. Farahnaky, O. Olatunde and T. Truong, Foods, 2022, 11, 1823 CrossRef CAS PubMed.
-
L. Gomez, B. Tiwari and M. Garcia-Vaquero, in Sustainable Seaweed Technologies, ed. M. D. Torres, S. Kraan and H. Dominguez, Elsevier, 2020, pp. 207–224, DOI:10.1016/B978-0-12-817943-7.00008-1.
- A. M. Goula, M. Ververi, A. Adamopoulou and K. Kaderides, Ultrason. Sonochem., 2017, 34, 821–830 CrossRef CAS PubMed.
- H. Kamal, C. F. Le, A. Salter and A. Ali, Compr. Rev. Food Sci. Food Saf., 2021, 20(3), 2455–2475 CrossRef PubMed.
- A. M. N. Lal, M. V. Prince, A. Kothakota, R. Pandiselvam, R. Thirumdas, N. K. Mahanti and R. Sreeja, Innovative Food Sci. Emerging Technol., 2021, 74, 102844 CrossRef CAS.
- J. Lonchamp, M. Akintoye, P. Clegg and S. Euston, Eur. Food Res. Technol., 2020, 246(4), 767–780 CrossRef CAS.
- A. C. Mellinas, A. Jiménez and M. C. Garrigós, LWT, 2020, 127, 109361 CrossRef CAS.
- D. Panda, V. Saharan, S. Manickam and R. Parate, Processes, 2020, 8(2), 220 CrossRef CAS.
- S. Pezeshk, M. Rezaei, H. Hosseini and M. Abdollahi, Food Hydrocolloids, 2021, 118, 106768 CrossRef CAS.
- K. E. Preece, N. Hooshyar, A. J. Krijgsman, P. J. Fryer and N. J. Zuidam, Innovative Food Sci. Emerging Technol., 2017, 41, 47–55 CrossRef CAS.
- M. M. Rahman and B. P. Lamsal, Compr. Rev. Food Sci. Food Saf., 2021, 20, 1457–1480 CrossRef CAS PubMed.
- J. P. Rivadeneira, T. Wu, Q. Ybanez, V. P. Migo, A. A. Dorado, F. R. P. Nayve and K. A. Israel, Int. J. Food Sci., 2020, 2020, 1–9 CrossRef PubMed.
- K. Shahid, V. Srivastava and M. Sillanpää, Environ. Sci. Pollut. Res.Int. J. Food Sci., 2021, 28, 1–21 CrossRef PubMed.
- A. Szaja, A. Montusiewicz and M. Lebiocka, Appl. Sci., 2022, 12, 7936 CrossRef CAS.
- S. Toepfl, Procedia Food Sci., 2011, 1, 776–779 CrossRef.
- K. Vilkhu, R. Mawson, L. Simons and D. Bates, Innovative Food Sci. Emerging Technol., 2008, 9, 161–169 CrossRef CAS.
- T. Yoshida, S. Tsubaki, Y. Teramoto and J.-i. Azuma, Bioresour. Technol., 2010, 101, 7820–7826 CrossRef CAS PubMed.
- F. Yusree, A. P. Peter, M. Z. Mohd Nor, P. L. Show and M. N. Mokhtar, Foods, 2021, 10(11), 2748 CrossRef CAS PubMed.
- G. Zhang, M. Hu, L. He, P. Fu, L. Wang and J. Zhou, Food Bioprod. Process., 2013, 91, 158–168 CrossRef CAS.
- S. Zhang, L. Sun, H. Ju, Z. Bao, X.-a. Zeng and S. Lin, Food Res. Int., 2021, 139, 109914 CrossRef CAS PubMed.
- H. Zheng, Y. Zheng and J. Zhu, Engineering, 2022 DOI:10.1016/j.eng.2022.04.027.
- L. Du, P. J. Arauzo, M. F. Meza Zavala, Z. Cao, M. P. Olszewski and A. Kruse, Molecules, 2020, 25, 488 CrossRef CAS PubMed.
- Y. Hou, Z. Wu, Z. Dai, G. H. Wang and G. Wu, J. Anim. Sci. Biotechnol., 2017, 8, 24 CrossRef PubMed.
- M. Peydayesh, M. Bagnani, W. L. Soon and R. Mezzenga, Chem. Rev., 2022 DOI:10.1021/acs.chemrev.2c00236.
- V. García Arteaga, V. Demand, K. Kern, A. Strube, M. Szardenings, I. Muranyi, P. Eisner and U. Schweiggert-Weisz, Foods, 2022, 11(1), 118 CrossRef PubMed.
- X. Liang, G. Qian, J. Sun, M. Yang, X. Shi, H. Yang, J. Wu, Z. Wang, Y. Zheng and X. Yue, Sci. Rep., 2021, 11, 18623 CrossRef CAS PubMed.
- A. Moure, H. Domínguez and J. C. Parajó, J. Agric. Food Chem., 2005, 53, 7600–7608 CrossRef CAS PubMed.
- H. B. Aditiya, T. M. I. Mahlia, W. T. Chong, H. Nur and A. H. Sebayang, Renewable Sustainable Energy Rev., 2016, 66, 631–653 CrossRef CAS.
- J. Wang, M. Zhao, X. Yang and Y. Jiang, J. Cereal Sci., 2006, 44, 93–100 CrossRef CAS.
- D. S. Lee, Y. G. Lee, E. J. Cho, Y. Song and H. J. Bae, Biotechnol. Biofuels, 2021, 14, 37 CrossRef CAS PubMed.
- A. A. Modenbach and S. E. Nokes, Biomass Bioenergy, 2013, 56, 526–544 CrossRef CAS.
- H. Kamal, C. F. Le, A. M. Salter and A. Ali, Compr. Rev. Food Sci. Food Saf., 2021, 20, 2455–2475 CrossRef PubMed.
- W. Zhang, T. Zhang, B. Jiang and W. Mu, Biotechnol. Adv., 2017, 35, 267–274 CrossRef CAS PubMed.
- S. Matassa, N. Boon, I. Pikaar and W. Verstraete, Microb. Biotechnol., 2016, 9, 568–575 CrossRef PubMed.
- A. Srividya, V. Vishnuvarthan, M. Murugappan and P. Dahake, Int. J. Pharm. Res. Scholars, 2013, 2, 1–4 Search PubMed.
- M. Moo-Young, Y. Chisti and D. Vlach, Biotechnol. Adv., 1993, 11, 469–479 CrossRef CAS PubMed.
- P. Lancheros, V. Lagos and H. H. Stein, J. Anim. Sci., 2020, 98, 62–62 CrossRef.
- C. H. Edwards, P. Ryden, A. M. Pinto, A. van der Schoot, C. Stocchi, N. Perez-Moral, P. J. Butterworth, B. Bajka, S. E. Berry, S. E. Hill and P. R. Ellis, J. Funct. Foods, 2020, 68, 103918 CrossRef CAS.
- M. Griep, T. Mets and D. Massart, Food Qual. Preference, 1997, 8, 151–156 CrossRef.
- N. D. Wahyono and M. M. D. Utami, J. Phys.: Conf. Ser., 2018, 953, 012125 CrossRef.
- K. H. A. Rahman, S. J. H. M. Yusof and Z. Zakaria, Trop. Agric. Sci, 2016, 39, 29–39 Search PubMed.
- S. W. Jones, A. Karpol, S. Friedman, B. T. Maru and B. P. Tracy, Curr. Opin. Biotechnol., 2020, 61, 189–197 CrossRef CAS PubMed.
- A. Zamani, M. Khajavi, M. H. Nazarpak and E. Gisbert, Animals, 2020, 10, 1676 CrossRef PubMed.
- R. W. Hardy, B. Patro, C. Pujol-Baxley, C. J. Marx and L. Feinberg, Aquacult. Res., 2018, 49, 2218–2224 CrossRef CAS.
- D. D. Sastraatmadja, F. Tomita and T. Kasai, J. Grad. School Agric., Hokkaido Univ., 2002, 70, 111–127 Search PubMed.
- S. Ciufo, D. M. Domrachev, H. C. L. Hotton, K. S. Kannan, K. R. Khovanskaya, D. Leipe, R. Mcveigh, K. O’Neill, B. Robbertse, S. Sharma, V. Soussov, J. P. Sullivan, L. Sun, S. Turner and I. Karsch-Mizrachi, Database, 2020, baaa062 Search PubMed.
-
H. Vanstaen, Verhandelingen van de Faculteit Landbouwwetenschappen te Gent (Belgium), 1976.
- D. Henry, Aust. Vet. J., 1975, 51, 317–319 CrossRef CAS PubMed.
- J. Hanssen, Z. Tierphysiol., Tierernaehr. Futtermittelkd., 1981, 46, 182–196 CrossRef CAS.
- M. Wiebe, Appl. Microbiol. Biotechnol., 2002, 58, 421–427 CrossRef CAS PubMed.
- T. J. A. Finnigan, B. T. Wall, P. J. Wilde, F. B. Stephens, S. L. Taylor and M. R. Freedman, Curr. Dev. Nutr., 2019, 3(6), nzz021 CrossRef PubMed.
- A. P. J. Trinci, Mycol. Res., 1992, 96, 1–13 CrossRef CAS.
- M. O. Coelho, A. J. Monteyne, M. V. Dunlop, H. C. Harris, D. J. Morrison, F. B. Stephens and B. T. Wall, Nutr. Rev., 2020, 78(6), 486–497 CrossRef PubMed.
- A. Denny, B. Aisbitt and J. Lunn, Nutr. Bull., 2008, 33, 298–310 CrossRef.
- B. M. Popkin and M. M. Slining, Obes. Rev., 2013, 14, 11–20 CrossRef PubMed.
- A. J. Monteyne, M. O. Coelho, C. Porter, D. R. Abdelrahman, T. S. Jameson, S. R. Jackman, J. R. Blackwell, T. J. Finnigan, F. B. Stephens and M. L. Dirks, Am. J. Clin. Nutr., 2020, 112, 318–333 CrossRef PubMed.
-
F. Somaye, M. N. Marzieh and N. Lale, Single Cell Protein (SCP) production from UF cheese whey by Kluyveromyces marxianus, National Congress of Food Industry 18, 2008 Search PubMed.
-
J. de la Gueriviere, Industries Agricoles et Alimentaires SCEES, 1981 Search PubMed.
- K. J. Phelps, J. S. Drouillard, T. G. O'Quinn, D. D. Burnett, T. L. Blackmon, J. E. Axman, C. L. Van Bibber-Krueger and J. M. Gonzalez, J. Anim. Sci., 2016, 94, 4016–4029 CrossRef CAS PubMed.
- L. R. Beuchat and S. M. M. Basha, Eur. J. Appl. Microbiol., 1976, 2, 195–203 CrossRef CAS.
- T. Linder, Food Secur., 2019, 11, 265–278 CrossRef.
- EFSA, Panel on Dietetic Products, Allergies, D. Turck, J. L. Bresson, B. Burlingame, T. Dean, S. Fairweather-Tait, M. Heinonen, K. I. Hirsch-Ernst, I. Mangelsdorf and H. McArdle, EFSA J., 2016, 14, e04594 Search PubMed.
- P. A. Bauman, A. C. Doxey, I. Eberini, E. Islamovic, F. Jungo, C. Kessenich, J. Kough, M. Krishan, L. Palazzolo, L. Privalle, C. E. Rodriguez, K. J. F. Satchell, A. Silvanovich and L. Pereira Mouriès, Regul. Toxicol. Pharmacol., 2022, 131, 105146 CrossRef CAS PubMed.
- R. D. Tee, D. J. Gordon, J. A. Welch and A. J. N. Taylor, Clin. Exp. Allergy, 1993, 23, 257–260 CrossRef CAS PubMed.
- W. Pang, D. Hou, J. Chen, E. E. Nowar, Z. Li, R. Hu, J. K. Tomberlin, Z. Yu, Q. Li and S. Wang, J. Environ. Manage., 2020, 260, 110066 CrossRef CAS PubMed.
- C. M. Collins, P. Vaskou and Y. Kountouris, Ann. Entomol. Soc. Am., 2019, 112, 518–528 CrossRef PubMed.
- D. Raheem, C. Carrascosa, O. B. Oluwole, M. Nieuwland, A. Saraiva, R. Millán and A. Raposo, Crit. Rev. Food Sci. Nutr., 2019, 59, 2169–2188 CrossRef CAS PubMed.
- D. Dobermann, J. A. Swift and L. M. Field, Nutr. Bull., 2017, 42, 293–308 CrossRef.
- L. Kouřimská and A. Adámková, NFS J., 2016, 4, 22–26 CrossRef.
- F. I. Oibiopka, H. O. Akanya, A. A. Jigam, A. N. Saidu and E. C. Egwim, Food Sci. Hum. Wellness, 2018, 7, 175–183 CrossRef.
- G. O. Latunde-Dada, W. Yang and M. Vera Aviles, J. Agric. Food Chem., 2016, 64, 8420–8424 CrossRef CAS PubMed.
- T. A. Churchward-Venne, P. J. M. Pinckaers, J. J. A. Van Loon and L. J. C. Van Loon, Nutr. Rev., 2017, 75, 1035–1045 CrossRef PubMed.
- R. Köhler, L. Kariuki, C. Lambert and H. Biesalski, J. Asia-Pac. Entomol., 2019, 22, 372–378 CrossRef.
- K. C. Surendra, R. Olivier, J. K. Tomberlin, R. Jha and S. K. Khanal, Renewable Energy, 2016, 98, 197–202 CrossRef CAS.
- G. Poma, Y. Fujii, S. Lievens, J. Bombeke, B. Gao, Y. Jeong, T. J. McGrath and A. Covaci, Food Chem. Toxicol., 2021, 154, 112311 CrossRef CAS PubMed.
- T. Veldkamp, N. Meijer, F. Alleweldt, D. Deruytter, L. Campenhout, L. Gasco, N. Roos, S. Smetana, A. Fernandes and H. J. Van der Fels-Klerx, Insects, 2022, 13, 281 CrossRef PubMed.
- M. Fraqueza and L. Patarata, Future Foods, IntechOpen, 2017, ch. 05, DOI:10.5772/intechopen.69300.
- B. Acosta-Estrada, A. Reyes, C. Rosell, D. Rodrigo and C. Ibarra, Front. Nutr., 2021, 8, 687712 CrossRef PubMed.
- A. Osimani and L. Aquilanti, Curr. Opin. Food Sci., 2021, 37, 112–117 CrossRef.
- EFSA Scientific Committee, EFSA J., 2015, 13(10), 4257 CrossRef.
- J. Ribeiro, L. Cunha, B. Sousa-Pinto and J. Fonseca, Mol. Nutr. Food Res., 2018, 62(1), 1700030 CrossRef PubMed.
- Y. H. Jo and J. Lee, Entomol. Res., 2016, 46, 2–4 CrossRef.
- A. Cappelli, E. Cini, C. Lorini, N. Oliva and G. Bonaccorsi, Food Control, 2020, 108, 106877 CrossRef CAS.
- B. Arru, R. Furesi, L. Gasco, F. Madau and P. Pulina, Sustainability, 2019, 11, 1697 CrossRef CAS.
- H. P. S. Makkar, G. Tran, V. Heuzé and P. Ankers, Anim. Feed Sci. Technol., 2014, 197, 1–33 CrossRef CAS.
- W. M. Rand, P. L. Pellett and V. R. Young, Am. J. Clin. Nutr., 2003, 77, 109–127 CrossRef CAS PubMed.
- G. D. P. Da Silva and T. Hesselberg, Neotrop. Entomol., 2020, 49, 151–162 CrossRef CAS PubMed.
- M. Banks, R. Johnson, L. Giver, G. Bryant and M. Guo, Curr. Opin. Biotechnol., 2022, 75, 102707 CrossRef CAS PubMed.
- T.-K. Kim, H. I. Yong, Y.-B. Kim, H.-W. Kim and Y.-S. Choi, Food Sci. Anim. Resour., 2019, 39, 521–540 CrossRef PubMed.
- S. B. Meyer, A. M. Wilson, M. Calnan, J. Henderson, J. Coveney, D. McCullum, A. R. Pearce, P. Ward and T. Webb, BMC Public Health, 2017, 17, 189 CrossRef PubMed.
- M. F. Stringer and M. N. Hall, Food Control, 2007, 18, 755–765 CrossRef.
-
FSA, Emerging technologies that will impact on the UK food system: Rapid evidence assessment, FSA, 2021 Search PubMed.
-
Campden BRI (Chipping Campden) Ltd, Comparing international approaches to food safety regulation of GM and Novel Foods, Food Standards Agency, 2021, Online, 20 April 2021 Search PubMed.
-
Singapore Food Agency, Requirements for the Safety Assessment of Novel Foods and Novel Food Ingredients, 2021, Online, 13 December 2021 Search PubMed.
- FSSAI Gazette notification of Food Safety and Standards (Approval for Non-Specified Food and Food Ingredients) Regulations, 2017.
-
J. Buijs, B. M. J. van der Meulen and L. Jiao, Pre-market Authorization of Food Ingredients and Products in Chinese Food Law: Legal Systematic Analysis of the Pre-Market Authorization Requirements of Food Ingredients and Products in the People's Republic of China, 2018, Online, October 14, 2018 Search PubMed.
- B. Magnuson, I. Munro, P. Abbot, N. Baldwin, R. Lopez-Garcia, K. Ly, L. McGirr, A. Roberts and S. Socolovsky, Food Addit. Contam., Part A, 2013, 30, 1147–1220 CrossRef CAS PubMed.
- A. Lähteenmäki-Uutela, S. B. Marimuthu and N. Meijer, J. Insects Food Feed, 2021, 7, 1–8 CrossRef.
- Commission Regulation (EU) 2021/1372 of 17 August 2021 amending Annex IV to Regulation (EC) No 999/2001 of the European Parliament and of the Council as regards the prohibition to feed non-ruminant farmed animals, other than fur animals, with protein derived from animals, OJ L 295, 18.8.2021, p. 1–17.
- Commission Regulation (EU) 2021/1925 of 5 November 2021 amending certain Annexes to Regulation (EU) No 142/2011 as regards the requirements for placing on the market of certain insect products and the adaptation of a containment method, OJ L 393, 8.11.2021, p. 4–8.
- Consultation on regulation of insect and insect products (imports and locally farmed/processed).
- Commission Regulation (EU) 2021/1372 of 17 August 2021 amending Annex IV to Regulation (EC) No 999/2001 of the European Parliament and of the Council as regards the prohibition to feed non-ruminant farmed animals, other than fur animals, with protein derived from animals. OJ L 295, 18.8.2021, p. 1–17.
- Commission Regulation (EU) 2017/893 of 24 May 2017 amending Annexes I and IV to Regulation (EC) No 999/2001 of the European Parliament and of the Council and Annexes X, XIV and XV to Commission Regulation (EU) No 142/2011 as regards the provisions on processed animal protein. OJ L 138, 25.5.2017, p. 92–116.
- Regulation (EC) No 999/2001 of the European Parliament and of the Council of 22 May 2001 laying down rules for the prevention, control and eradication of certain transmissible spongiform encephalopathies. OJ L 147, 31.5.2001, p. 1–40.
- O. Birrenbach, F. Faust, M. Ebrahimi, R. Fan and P. Czermak, Front. Chem. Eng., 2021, 3, 656345 CrossRef.
- R. Tacharatanamanee, K. Cherdrungsi and W. Youravong, J. Teknol., 2004, 41(1), 1–10 Search PubMed.
- S. Nagappan, D. M. Phinney and D. R. Heldman, Appl. Sci., 2018, 8, 2694 CrossRef CAS.
-
L. K. Wang, N. K. Shammas, M. Cheryan, Y.-M. Zheng and S.-W. Zou, in Membrane and Desalination Technologies, ed. L. K. Wang, J. P. Chen, Y.-T. Hung and N. K. Shammas, Humana Press, Totowa, NJ, 2011, pp. 237–269, DOI:10.1007/978-1-59745-278-6_6.
- B. Das, S. Sarkar, A. Sarkar, S. Bhattacharjee and C. Bhattacharjee, Process Saf. Environ. Prot., 2016, 101, 27–33 CrossRef CAS.
-
H. Hultin, H. Kristinsson, T. Lanier and J. Park, in Surimi and Surimi Seafood, 2005, ch. 3, pp. 107–139, DOI:10.1201/9781420028041.ch3.
- J. Veide Vilg and I. Undeland, J. Appl. Phycol., 2017, 29(1), 585–593 CrossRef PubMed.
- M. Assfalg, Molecules, 2021, 26, 7079 CrossRef CAS PubMed.
- S. M. Andler and J. M. Goddard, npj Sci. Food, 2018, 2, 19 CrossRef PubMed.
- I. Undeland, S. D. Kelleher and H. O. Hultin, J. Agric. Food Chem., 2002, 50, 7371–7379 CrossRef CAS PubMed.
- C. Rivas Vela, E. Castaño-Tostado, S. Amaya-Llano and G. Castillo-Herrera, Molecules, 2021, 26, 6655 CrossRef CAS PubMed.
- M. B. Esteban, A. J. García, P. Ramos and M. d. C. Márquez, Bioresour. Technol., 2009, 101, 2472–2476 CrossRef PubMed.
- X. Zhu, C. Zhu, L. Zhao and H. Cheng, Chin. J. Chem. Eng., 2008, 16, 456–460 CrossRef CAS.
- S. Sunphorka, W. Chavasiri, Y. Oshima and S. Ngamprasertsith, J. Supercrit. Fluids, 2012, 65, 54–60 CrossRef CAS.
- A. Espinoza and R. Morawicki, J. Agric. Food Chem., 2012, 60, 5250–5256 CrossRef CAS PubMed.
- I. Marcet, C. Álvarez, B. Paredes and M. Díaz, J. Agric. Food Chem., 2014, 62(32), 8179–8186 CrossRef CAS PubMed.
- H. Ziero, L. Buller, A. Mudhoo, L. Castro Ampese, S. Mussatto and T. Forster-Carneiro, J. Environ. Chem. Eng., 2020, 8, 104406 CrossRef.
- R. Melgosa, M. Marques, A. Paiva, A. Bernardo, N. Fernández, I. De Sá Nogueira and P. Simões, Foods, 2021, 10, 1222 CrossRef CAS PubMed.
- H. Yoshida and O. Tavakoli, J. Chem. Eng. Jpn., 2004, 37, 253–260 CrossRef CAS.
- T. Powell, S. Bowra and H. Cooper, J. Am. Soc. Mass Spectrom., 2017, 28(9), 1775–1786 CrossRef CAS PubMed.
- T. Powell, S. Bowra and H. Cooper, Anal. Chem., 2016, 88(12), 6425–6432 CrossRef CAS PubMed.
- M. Álvarez-Viñas, P. Rodriguez Seoane, N. Flórez-Fernández, M. Torres, B. Reinoso, A. Moure and H. Domínguez, Food Bioprocess Technol., 2021, 14(3), 373–387 CrossRef.
- I. Marcet, C. Álvarez, B. Paredes and M. Díaz, Waste Manage., 2016, 49, 364–371 CrossRef CAS PubMed.
- K. Spalvins, L. Zihare and D. Blumberga, Energy Procedia, 2018, 147, 409–418 CrossRef CAS.
- M. Molfetta, E. Morais, L. Barreira, G. Bruno, F. Porcelli, E. Dugat-Bony, P. Bonnarme and F. Minervini, Foods, 2022, 11, 2065 CrossRef CAS PubMed.
- A. Ritala, S. Häkkinen, M. Toivari and M. Wiebe, Front. Microbiol., 2017, 8, 2009 CrossRef PubMed.
-
J. Whittaker, R. Johnson, T. Finnigan, S. Avery and P. Dyer, in Grand Challenges in Fungal Biotechnology, Springer, Cham, 2020, pp. 59–79, DOI:10.1007/978-3-030-29541-7_3.
- M. Hoff, R. Trüeb, B. Ballmer-Weber, S. Vieths and B. Wüthrich, J. Allergy Clin. Immunol., 2003, 111, 1106–1110 CrossRef PubMed.
- M. Areniello, S. Matassa, G. Esposito and P. N. L. Lens, Trends Biotechnol., 2022 DOI:10.1016/j.tibtech.2022.07.008.
- J. Bader, E. Mast-Gerlach, M. Popović, R. Bajpai and U. Stahl, J. Appl. Microbiol., 2010, 109, 371–387 CrossRef CAS PubMed.
-
P. Bajpai, Single cell protein production from lignocellulosic biomass, Springer, 2017, pp. 1–78 Search PubMed.
- X. Hu, P. Vandamme and N. Boon, Chem. Eng. J., 2021, 429, 132535 CrossRef.
- M. Spiller, M. Muys, G. Papini, G. de Sousa, M. Sakarika, M. Buyle and S. Vlaeminck, Water Res., 2019, 171, 115406 CrossRef PubMed.
- A. Tesfaw and F. Assefa, Biotechnol. Mol. Biol. Rev., 2014, 9, 12–20 CrossRef.
- G. Yu, Y. Sun, H. Han, X. Yan, Y. Wang, X. Ge, B. Qiao and L. Tan, Front. Microbiol., 2021, 12, 663924 CrossRef PubMed.
- M. Sharif, M. Zafar, A. Aqib, M. Saeed, M. Farag and M. Alagawany, Aquaculture, 2021, 531, 735885 CrossRef CAS.
- A. T. Nasseri, S. Rasoul-Amini, M. H. Morowvat and G. Younes, Am. J. Food Technol., 2011, 6(2), 103–116 CrossRef CAS.
- H. Onyeaka, C. Anumudu, C. Okpe, A. Okafor, F. Ihenetu, T. Miri, O. Odeyemi and A. Anyogu, Open Microbiol. J., 2022, 16, e187428582206160 CrossRef CAS.
- S. Jones, S. Friedman, B. Maru and B. Tracy, Curr. Opin. Biotechnol., 2020, 61, 189–197 CrossRef CAS PubMed.
-
P. Bajpai, in Single Cell Protein Production from Lignocellulosic Biomass, 2017, pp. 59–63, DOI:10.1007/978-981-10-5873-8_8.
- S. F. S. Reihani and K. Khosravi, Electron. J. Biotechnol., 2018, 37, 34–40 CrossRef.
- P. Ravindra, R. Rudravaram, A. Chandel, V. Linga and Y. Z. Hui, Food Energy Secur., 2009, 73–97 Search PubMed.
- M. Mezes, Acta Aliment., 2018, 47, 513–522 CrossRef CAS.
- I. S. Modahl and A. Brekke, SN Appl. Sci., 2022, 4, 183 CrossRef.
- P. J. Arauzo, L. Du, M. P. Olszewski, M. F. Meza Zavala, M. J. Alhnidi and A. Kruse, Bioresour. Technol., 2019, 293, 122117 CrossRef CAS PubMed.
- V. Vasudevan Ramakrishnan, A. E. Ghaly, M. S. Brooks and S. M. Budge, J. Bioprocess. Biotech., 2013, 3, 2–9 Search PubMed.
- F. Liew, M. E. Martin, R. C. Tappel, B. D. Heijstra, C. Mihalcea and M. Köpke, Front. Microbiol., 2016, 7, 694 Search PubMed.
- J. Strong, M. Kalyuzhnaya, J. Silverman and W. P. Clarke, Bioresour. Technol., 2016, 215, 314–323 CrossRef PubMed.
- C. Wen, J. Zhang, Y. Duan, H. Zhang and H. Ma, J. Food Sci., 2019, 84, 3330–3340 CrossRef CAS PubMed.
- A. Gildberg and E. Stenberg, Process Biochem., 2001, 36, 809–812 CrossRef CAS.
- Q. Lu, H. Liu, W. Liu, Y. Zhong, C. Ming, W. Qian, Q. Wang and J. Liu, Water Sci. Technol., 2017, 76, 1852–1866 CrossRef CAS PubMed.
- T. Upcraft, W.-C. Tu, R. Johnson, T. Finnigan, N. Van Hung, J. Hallett and M. Guo, Green Chem., 2021, 23, 5150–5165 RSC.
- K. Rachwał, A. Waśko, K. Gustaw and M. Polak-Berecka, PeerJ, 2020, 8, e9427–e9427 CrossRef PubMed.
- K. Lelicińska-Serafin, A. Rolewicz-Kalińska and P. Manczarski, Int. J. Environ. Res. Public Health, 2019, 16, 3009 CrossRef PubMed.
- D. Batista Meneses, G. Montes de Oca-Vásquez, J. R. Vega-Baudrit, M. Rojas-Álvarez, J. Corrales-Castillo and L. C. Murillo-Araya, Biomass Convers. Biorefin., 2022, 12, 547–564 CrossRef CAS.
- J. Rockström, W. Steffen, K. Noone, Å. Persson, F. S. Chapin III, E. Lambin, T. M. Lenton, M. Scheffer, C. Folke, H. Schellnhuber and J. Foley, Ecol. Soc., 2009, 14, 32 CrossRef.
- W. Willett, J. Rockström, B. Loken, M. Springmann, T. Lang, S. Vermeulen, T. Garnett, D. Tilman, F. DeClerck and A. Wood, Lancet, 2019, 393, 447–492 CrossRef PubMed.
Footnotes |
† Electronic supplementary information (ESI) available: Supplementary information 1–8 (PDF) and Supplementary Tables 1–7 (Excel). See DOI: https://doi.org/10.1039/d2gc03095k |
‡ Equivalent contributions as co-first authors. |
§ Equivalent contributions. |
|
This journal is © The Royal Society of Chemistry 2023 |
Click here to see how this site uses Cookies. View our privacy policy here.