DOI:
10.1039/D2GC02483G
(Critical Review)
Green Chem., 2023,
25, 2930-2957
Leveraging the bioeconomy for carbon drawdown†
Received
4th July 2022
, Accepted 10th March 2023
First published on 6th April 2023
Abstract
Stringent climate change mitigation scenarios rely on large-scale drawdown of carbon dioxide from the atmosphere. Amongst drawdown technologies, bioenergy with carbon capture and sequestration (BECCS) has received considerable attention in the climate mitigation literature. Recently, attention has shifted further from a relatively narrow focus on BECCS to a broader focus on Biomass Carbon Removal and Storage (BiCRS). The concept of BiCRS has the potential to enable a future where the climate mitigation value of biomass resources is more valuable than the energy value, due to the potential to remove and sequester large quantities atmospheric CO2. This article provides a qualitative overview of prominent BiCRS technologies from which a set of the most promising technologies are assessed quantitively through life cycle assessment. There are numerous opportunities to incorporate carbon removal and management within the bioeconomy, but the majority of immediate carbon removal potential exists in four bioproducts: bioenergy, bioplastics, biochar, and wood products. We analyze the life cycle greenhouse gas emissions and disposition of sequestered carbon over 10
000 years for four bioproducts representative of each broader category: an advanced BECCS pathway, biopolyethylene, oriented strand board, and biochar soil amendment. We find that the BECCS pathway has the greatest magnitude and durability of CO2 storage over all time horizons. However, non-BECCS pathways achieve 34–64% of the drawdown magnitude relative to BECCS and retain 55–67% of their initial drawdown over 100 years (central estimate). We identify three engineering strategies for enhancing carbon drawdown: reducing biomass supply chain emissions, maximizing carbon stored in long-lived products, and extending the term of carbon storage. Finally, we highlight the need to characterize both the magnitude and permanence of carbon drawdown as a means for policymakers and technology developers to deploy limited biomass resources to maximize mitigation benefits.
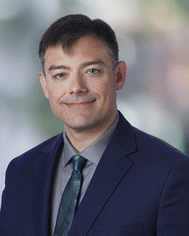 John P. Dees | John Dees is a PhD Candidate at the Energy and Resources Group, University of California, Berkeley and Graduate researcher under Dr Dan Sanchez. He is an interdisciplinary scholar with a recent emphasis on life cycle and technoeconomic assessment of biomass carbon removal and storage (BiCRS). He previously collaborated with Lawrence Livermore National Lab to understand decarbonization options in the corn ethanol industry. Specific interests include bioenergy with carbon capture and sequestration, carbon storage in biopolymers, biochar, and harvested wood products, and economic valuation of non-permanent carbon storage using integrated assessment models. |
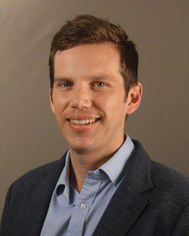 William Joe Sagues | Joe Sagues is an Assistant Professor in Biological & Agricultural Engineering at North Carolina State University and Principal Investigator of the Biocarbon Utilization & Sequestration Lab. Prior to his current appointment, he conducted research at the US Department of Energy and scaled up an advanced biofuel technology. His lab takes an interdisciplinary and applied approach to identify low-risk, near-term opportunities to leverage the bioeconomy for carbon removal. Specific interests include integrating CO2 capture in existing and emerging bioprocesses, such as fermentation, anaerobic digestion, chemical pulping, and graphitization. |
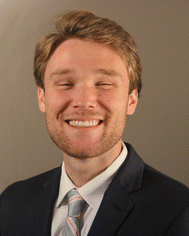 Ethan Woods | Ethan Woods is a PhD student at North Carolina State University and a Graduate Research Assistant in the Biocarbon Utilization and Sequestration Lab. He previously spent time as a Technology-to-Market Summer Scholar for the Advanced Research Project Agency, and also as a delegate and policy consultant for the Consortium for Advanced Bioeconomy Leadership Education. His research is focused on the enhancement and acceleration of natural carbon sequestration pathways, and techno-economic modelling of the commercialization of these pathways. |
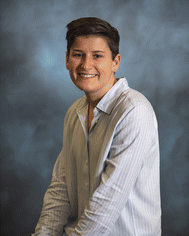 Hannah M. Goldstein | Hannah is an Energy Technology Analyst at the Lawrence Livermore National Laboratory (LLNL). Since starting at LLNL, Hannah has been involved in many diverse projects including the LLNL Flow Chart initiative, the Carbon Initiative, cyber security and more recently joining the Nuclear Enterprise Working Group. Hannah assesses the cost and performance of technologies that reduce the environmental climate impacts of energy and materials production. During her time at LLNL, Hannah has assessed several energy technologies and policies that focus on carbon capture, reducing life cycle emissions of carbon dioxide to the atmosphere, and those that produce negative emissions. Through these assessments she applies tools such as Life Cycle Analysis (LCA), techno-economic assessment (TEA), and energy system modeling. Hannah holds a B.A. in Engineering with a minor in Mechanical Engineering from Lafayette College. Hannah also holds an M.S. in Energy Science, Technology and Public Policy from Carnegie Mellon University. Hannah is currently pursuing further education at George Washington University for an M.S. in Project Management. |
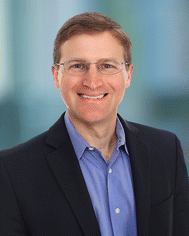 A.J. Simon | A.J. Simon is the Head of Industrial Decarbonization at Carbon Direct, where he is responsible for the firm's advisory practice in hybrid and engineered carbon removal strategies. Prior to Carbon Direct, he was the Group Leader for Energy at Lawrence Livermore National Laboratory (2008–2022), an Energy Systems Analyst at Stanford's Global Climate and Energy Project (2003–2008) and a Mechanical Engineer at General Electric's Global Research Center (2001–2003). He has led diverse technology assessment and systems analysis projects at multiple scales and across numerous disciplines including carbon dioxide capture and drawdown, the energy-water nexus, fusion energy, nuclear fuel reprocessing, hydrogen fuel, building energy efficiency, and soldier-portable power. At Livermore, A.J. was the principal investigator for the Lab's Energy Flow Charts (https://flowcharts.llnl.gov). He served as the Scientific Editor of LLNL's flagship magazine, Science and Technology Review (https://str.llnl.gov) and he was a core author on the U.S. Department of Energy's 2015 Quadrennial Technology Review (https://energy.gov/qtr). In 2018, he completed the Department of Energy's Oppenheimer Science and Energy Leadership Program. A.J. holds an M.S. and B.S. in Mechanical Engineering from Stanford University. |
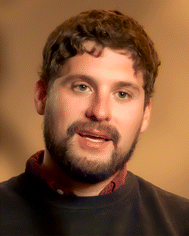 Daniel L. Sanchez | Daniel L. Sanchez studies engineered biomass & bioenergy systems that remove CO2 from the atmosphere. Trained as an engineer and energy systems analyst, Sanchez's work and engagement spans the academic, nongovernmental, and governmental sectors. As an Assistant Professor of Cooperative Extension, he runs the Carbon Removal Lab, which aims to commercialize sustainable carbon dioxide removal technologies, and supports outreach to policymakers and technologists in California and across the United States. Prior to joining the faculty of UC Berkeley, Daniel was a AAAS Congressional Science and Engineering Fellow serving in the Office of Senator Michael Bennet (D-CO). Dr Sanchez is currently on entrepreneurial leave from UC Berkeley. |
Introduction
The bioeconomy is a complex set of economic activities that utilize renewable forms of biogenic carbon from agriculture, forestry, and aquaculture for their conversion into food, feed, fiber, polymers, bioenergy, and other bioproducts.1 A central motive for the bioeconomy is the principle of circularity, which is applied at different steps of the value chain in order to retain the value of all resources in the economic cycle for as long as possible before these resources reach their end-of-life stage. One of the major benefits of adopting the principles of circularity is a reduction in greenhouse gas emissions, which mitigates the effects of climate change. Transitioning from the fossil resource-dependent linear economic model of “take-make-waste” to a circular bioeconomy will involve a coordinated effort from stakeholders across the value chain. As such, there is not just one singular bioeconomy, but many regional bioeconomies that vary technically, culturally, and politically.
Governments from many nations are formally embracing policies to enhance circularity, and their respective bioeconomies will play pivotal roles.2 As primary stakeholders, governments play a key role both in agenda-setting and financial incentives and support for the bioeconomy. In the United States, the bioeconomy represents more than 22% of total economic activity, valued at more than $1 trillion, and employs ∼28% of the workforce.3 The US bioeconomy has evolved into a highly productive engine of economic activity providing essential products to societies around the globe; however, there are striking inefficiencies. Approximately 30–50% of mass in food and agricultural systems is lost between biomass cultivation and end product sale.3 A significant portion of this waste is in the gaseous forms of carbon dioxide and methane, two of the leading greenhouse gases contributing to climate change, with the latter methane having a warming effect 27 to 83 times more powerful than carbon dioxide over 100 years and 20 years respectively.4 Reducing inefficiencies through the principles of circularity and related practices, such as bioproduct cascading, will sequester carbon in useful products—thereby avoiding emissions and mitigating climate change. In addition to eliminating efficiencies, increasing the supply of affordable and sustainable biomass resources will increase the economic and environmental impacts of a particular bioeconomy.
In the US, the Department of Energy has estimated that ∼1 billion dry tons of lignocellulosic biomass could be sustainably produced each year by 2040.5 Lignocellulosic biomass encompasses biomass material with lignin and cellulose within the cell wall, conferring woody characteristics, to include wood, grasses, agricultural residues, and similar materials. The intended application for a majority of the additional biomass resources has been toward avoiding fossil carbon emissions through the production and consumption of bioenergy products (e.g. biogas, biofuels, bio-hydrogen), which produce considerably less life cycle carbon emissions than fossil energy products.6 Notably, attention toward purely bioenergy products has shifted to bioenergy products with carbon capture and sequestration (BECCS) as the need for carbon-negative energy has become increasingly apparent.7 BECCS technologies include biomass to power, heat, steam, hydrogen, or other gaseous or liquid fuels, combined with technologies that can capture carbon dioxide emitted by biochemical or thermochemical processes specific to the energy product conversion technology. Carbon capture and sequestration (CCS) technologies include capture via solvent adsorption, pressure swing adsorption, cryogenic, or membrane approaches, among others.8 Process engineering, CO2 concentration, energy requirements, and cost inform the selection of CCS technologies, with dilute CO2 streams, as are found in direct biomass combustion processes, requiring more technical intervention than high-purity streams such as are found in fermentation (e.g. fuel ethanol from corn). According to the IPCC, most emissions pathways that limit global warming to 1.5 C–2.0 C require the removal and sequestration of 1–20 billion tonnes of atmospheric CO2 per year by 2050.7 For reference, the global transportation sector emits ∼10 billion tonnes of CO2 per year.
Recently, attention has shifted further from a relatively narrow focus on BECCS to a broader focus on Biomass Carbon Removal and Storage (BiCRS).9 BiCRS is relatively new nomenclature intended to supplant the energy product focus of BECCS, to include a much broader set of approaches that utilize biomass to capture and store atmospheric CO2. BiCRS is defined by its progenitors to include all approaches that “(a) use[ ] biomass to remove CO2 from the atmosphere, (b) store[ ] that CO2 underground or in long-lived products, [and] (c) do[ ] no damage to—and ideally promote[ ]—food security, rural livelihoods, biodiversity conservation and other important values”.9 The concept of BiCRS has the potential to enable a future where the carbon content of biomass resources is more valuable than the energy content, due to the potential to remove and sequester large quantities atmospheric CO2.9 In practice, BiCRS enables and expands the production of a variety of carbon-negative bioproducts including wood products (e.g. Oriented Strand Board/OSB), bioplastics (e.g. polyethylene), biocarbon (e.g. biochar), and purified biogenic CO2 with geological sequestration, among others.
Such a future would require robust and reliable economic incentives for BiCRS, most likely through policy frameworks.9 Stakeholders across the bioeconomy, including public and private sectors, must be engaged and involved in the process of crafting incentives that place a higher value on sequestered biocarbon.10,11 In the US, the bioeconomy is in the early stages of embracing the concept of BiCRS with the help of several policy tools, such as the low carbon fuel standard of California and the 45Q tax credit.12,13 However, a robust policy framework specific to BiCRS would significantly increase the bioeconomy's impact on mitigating climate change. The federal Renewable Fuel Standard is a good example of a policy framework that has a focused impact on the bioeconomy through rapid adoption and implementation of biofuels. A similar framework focused on BiCRS might catalyze rapid adoption and implementation of biomass-enabled carbon dioxide removal (CDR). Notably, to achieve the 1.5 C target set forth by the IPCC, the implementation of carbon-negative technologies must not be limited to developed countries, likely requiring governments to be open to cross-cutting international agreements.14
The number of carbon-negative technologies under development has expanded rapidly over the last 5–10 years to include a variety of disciplines such as genetic engineering, chemical engineering, and soil science, to name a few. Carbon-negative technologies have been refined into seven general classifications: bioenergy with carbon capture and storage (BECCS), afforestation and reforestation, direct air carbon capture and storage (DACCS), enhanced weathering, biochar, and soil carbon sequestration.15,16 Potential CO2 removal capacities in the year 2050 were estimated to be 0.5–5 GtCO2 per year for BECCS, 0.5–3.6 GtCO2 per year for afforestation and reforestation, 0.5–5 GtCO2 per year for DACCS, 2–4 GtCO2 per year for enhanced weathering, 0.5–2 GtCO2 per year for biochar, and up to 5 GtCO2 per year for soil carbon sequestration.16 Cumulatively, these technologies combined could provide 9–24.6 GtCO2 per year, which would meet the aforementioned requirement set forth by the IPCC.
Estimated costs of leading carbon-negative technologies range from $5–$300 per tCO2, with BECCS costing $100–$200 per tCO2, afforestation and reforestation costing $5–$50 tCO2, DAC costing $100–$300 per tCO2 (for nth of a kind plant, i.e. beyond first-of-kind), enhanced weathering costing $50–$200 per tCO2, biochar costing $90–$120 per tCO2, and soil carbon sequestration costing $0–$100 per CO2.15 Notably, the aforementioned costs do not incorporate degree of carbon permanence or risk of reversal. Afforestation, reforestation, and soil carbon sequestration have the lowest cost, but also have the lowest permanence and highest risk of reversing carbon storage through re-emission to the atmosphere. The life cycle carbon intensity of emerging carbon negative technologies must be thoroughly and responsibly assessed on cradle-to-grave bases to avoid the promotion of unrealistic carbon removal benefits.17
Overall, costs of strictly capturing and sequestering atmospheric CO2 are not justified with existing policy frameworks.8 Opportunities for CO2 capture, utilization, and sequestration appear to be more economically feasible in the near-term.18 However, the carbon permanence of technologies aimed at strictly removing CO2 from the atmosphere, particularly geologic storage of CO2, are overall greater than for technologies that incorporate CO2 utilization.19 Soil carbon sequestration is one particular approach that has uncertain carbon permanence based on many factors, thereby requiring significant advancements in carbon monitoring and LCA methodologies to reduce risk and improve carbon permanence reliability.20 BECCS relying on dedicated energy crops with geologic storage of CO2 has high permanence, but has significant implications regarding land use change, thereby warranting caution to avoid food displacement and biodiversity loss.21
Although widespread commercial deployment of BiCRS technologies has not yet been achieved in the US or abroad, there has been significant advancement in the research, development, and demonstration of BiCRS technologies. While numerous high quality reviews have covered the topics of BECCS22,23 and carbon dioxide removal (CDR)20 more generally, we are unaware of a comprehensive review of the broader range of CDR options offered by the BiCRS framework. This article offers a novel qualitative overview of leading BiCRS technologies from which a set of the most promising technologies are assessed quantitively through life cycle assessment, opening the door to a broader suite of biomass-based CDR options beyond the narrow BECCS framework.
Carbon negative bioproducts
The bioeconomy is essentially a facilitator and promoter of organized biological CO2 fixation into a wide range of different end products with varying degrees of permanence (Fig. 1). There are numerous opportunities to incorporate carbon removal and management within the bioeconomy, but the majority of the near-term carbon removal potential exists in four bioproducts: bioenergy, bioplastics, biochar, and wood products. Herein, we explain the various mechanisms by which carbon removal can be incorporated into each of these four bioproducts. We also provide a qualitative assessment of two emerging bioproducts for carbon removal, namely steel and concrete, that have significant potential for carbon removal of the long-term.
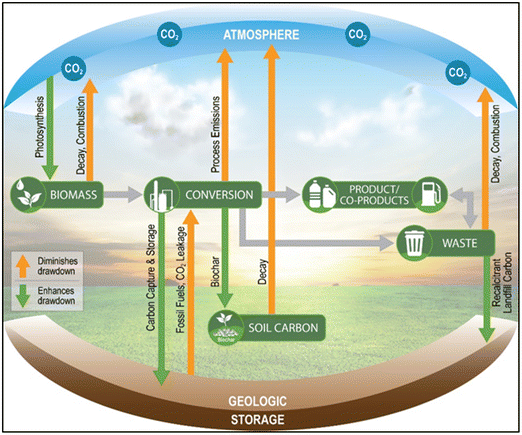 |
| Fig. 1 Carbon flows associated with carbon drawdown from bioproducts. To enhance carbon drawdown, the flows represented by the green arrows need to increase in magnitude while minimizing the flows represented by the orange arrows. The grey arrows represent biogenic carbon moving through the bioeconomy life cycle while the orange and green arrows indicate carbon losses and storage, respectively. | |
Bioenergy
Many different bioenergy technologies exist and span a wide range of technology readiness levels (TRLs), but they can generally be classified as either thermochemical or biochemical. Biochemical technologies use microorganisms and/or enzymes to convert the biomass resource into a bioenergy product, whereas thermochemical technologies rely on heat and catalysts. There are three primary, high TRL thermochemical pathways for bioenergy products with carbon removal, as shown in Fig. 2.
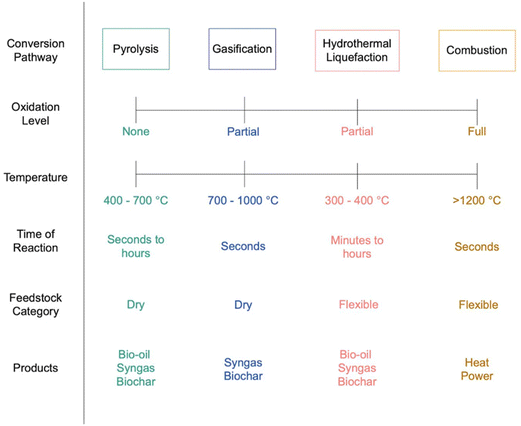 |
| Fig. 2 Thermochemical bioenergy pathways. | |
Pyrolysis entails the thermal treatment of biomass in an oxygen-free environment, wherein biocarbon is transformed into bio-oil, pyrogas, and biochar. Gasification entails the thermal treatment of biomass in an oxygen-lean environment, wherein biocarbon is partially oxidized into syngas (CO, CO2, and H2). Combustion entails the thermal treatment of biomass in an oxygen-rich environment, wherein biocarbon is fully oxidized into CO2. Hydrothermal liquefaction is a thermochemical biomass conversion process in which feedstocks with high moisture content are converted to bio-oil, syngas, and biochar. The process entails the degradation of biomolecular compounds in the feedstock by high pressure water in a medium temperature setting to form bio-oil which can then be upgraded into hydrocarbons vis hydrotreating.24,25 Complex thermochemical biomass conversion processes for carbon removal including gasification26–28 and combustion29 typically require large scales of operation to be economically viable, thereby limiting opportunities to areas with high densities of low-cost biomass feedstocks. Hydrothermal liquefaction (HTL)25 and pyrolysis26,30 have the potential to be economically viable at smaller scales due to the relatively mild process requirements. However, should they be necessary, operations for upgrading of biocrude and bio-oil from HTL and pyrolysis typically require economies of scale.31 Fortunately, thermochemical biomass conversion technologies are amenable to processing multiple different biomass feedstocks, whereas biochemical approaches typically require one feedstock.
Pyrolysis and gasification both have potential for relatively low-cost carbon removal due to the generation of biochar in the former case and high purity H2, fuels, and CO2, in latter case. Specifically, the biochar generated via pyrolysis can be land applied for soil carbon sequestration and the concentrated CO2 generated via gasification and gas clean up can be sequestered geologically with H2 and catalytically-produced fuels providing additional revenues. It is important to note that only gasification to H2 and power can achieve 100% carbon removal. When gasification yields liquid fuels (GtL), a significant portion of the carbon is carried by the liquid fuel which may be impractical to capture at the point of combustion (e.g. tailpipe). The bio-oil generated via pyrolysis has traditionally been viewed as a potential fuel precursor, but more recent work has illuminated its potential to store carbon via geological sequestration.32 The efficacy of bio-oil geological sequestration is still uncertain and requires continued research.32 Incorporating carbon removal in the combustion of biomass for electrical power, traditionally referred to as bioenergy with carbon capture and sequestration (BECCS), requires costly CO2 capture technologies, such monoethanolamine (MEA) scrubbing and stripping or oxycombustion. In general, BECCS for biopower is not viewed as an ideal pathway for biomass carbon removal and storage (BiCRS) due to the significant land requirement and high costs relative to alternative, low-carbon power generation technologies (solar, wind, geothermal, nuclear, etc.).9,33 Nonetheless, there exists the potential to sequester 737 million metric tons of CO2 per year at costs of $42–$92 per metric ton by 2040 with widespread implementation of BECCS for biopower across the United States.34 Relative to combustion, gasification-enabled BECCS for biopower has advantages, although most experts believe hydrogen, not electrical power, will be the most economically competitive bioenergy product from gasification.35,36 The decreasing costs of carbon-free electricity (e.g. solar and wind power) coupled with the need to remove carbon from the atmosphere and decarbonize hydrogen production have made biomass gasification highly advantageous.37–39
Regional biomass availability, climate, water availability, and land type, process conditions, and scale of operation all play critical factors in determining the techno-economic feasibility a particular BECCS technology.40,41 Coupling thermochemical BECCS with other carbon removal technologies, including DACCS and soil carbon sequestration, has the potential to offer benefits such as locational flexibility and enhanced carbon removal per unit area.42 Leveraging existing thermochemical bioenergy facilities for carbon removal also has the potential to enhance carbon removal and reduce costs. For example, US pulp mills emit ∼115 million tonnes of biogenic CO2 per year that are available for carbon removal without the new capital and extensive land use that is required of greenfield BECCS plants.43 Thus, near-term efforts for carbon removal via thermochemical energy processes could focus on the existing pulp and paper industry.
Relative to thermochemical pathways, biochemical pathways for bioenergy offer several advantages, including the ability to operate under mild conditions, generate relatively pure products, and produce gaseous waste streams of high CO2 concentration. However, the volumetric productivity (g L−1 h−1) of biochemical pathways may be one to two orders of magnitude lower than those of thermochemical pathways and thus require large reactors to achieve the same level of production.44,45 Thus carbon removal will generally be an auxiliary benefit to biochemical conversion for energy and materials. There are two primary, high TRL biochemical pathways for bioenergy products with carbon removal, as shown in Fig. 3.
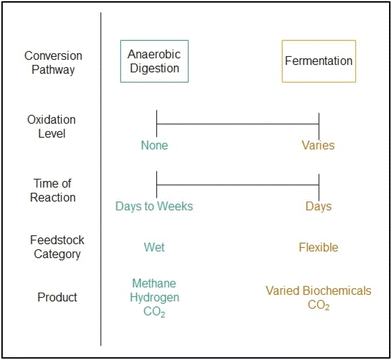 |
| Fig. 3 Two primary biochemical energy pathways. | |
Fermentation, in the context of this review article, entails the conversion of biomass to biofuels via pure culture fermentation under aerobic or anaerobic environments. Anaerobic digestion, in the context of this review article, entails the conversion of biomass to biofuels via mixed culture fermentation under anaerobic environments. In the US, existing industrial operations for the fermentation of corn starch to ethanol and anaerobic digestion of biowaste to biogas have the potential to remove ∼45 Mt-CO2 and ∼110 Mt-CO2, respectively.46–49 For comparison, in Brazil, existing industrial operations for the fermentation of sugarcane to ethanol have the potential to remove ∼28 Mt-CO2 per year.50 Thus, near-term efforts for carbon removal via biochemical energy processes could focus on the existing ethanol and biogas industries. The CO2 concentrations in waste streams associated with ethanol fermentation and anaerobic digestion are relatively high, thereby justifying their consideration for carbon removal since the costs of CO2 capture and sequestration are highly dependent on incoming CO2 concentration.51 In the US, 60% of CO2 from ethanol refineries could be captured and compressed for less than $25 per tonne, which is considerably less than costs for traditional BECCS.46 Ethanol fuel prices would increase by ∼3.5% with incorporation of CO2 capture and sequestration, which is not significant. Notably, the CO2 off gassed during ethanol fermentation requires minimal separation, allowing for such low costs of removal. Historically, CO2 from ethanol fermentation has been used for carbonating beverages and other food applications, but there is significant potential for geological sequestration.52 Archer Daniels Midland (ADM) successfully captured, compressed, and injected over 1 million metric tons of fermentation-derived CO2 into the Mt. Simon Sandstone geological formation in Decatur, Illinois, thereby demonstrating the ethanol industry's ability to rapidly and successfully scale carbon capture & sequestration.53 In addition, techno-economic assessments have shown the potential for microalgae growth and cultivation using CO2-derived from ethanol fermentation.54 The CO2 concentration and availability from biogas operations is a bit less clear given the diversity of sources (landfills, agricultural digesters, and wastewater treatment plants) and biogas end-uses (venting, flaring, and combustion).
Although there is significant potential to utilize and sequester existing biogenic CO2 from industrial operations in the US, the need for new biorefining pathways for enhanced carbon removal are required. Several recent studies have shown the immense potential for carbon removal via soil carbon sequestration coupled with fermentation of lignocellulosic biomass into ethanol.55,56 Specifically, the cultivation of switchgrass for biofuel production coupled with CO2 capture and sequestration has climate mitigation potential 4 and 15 times larger than forest and grassland restoration, respectively.55 Second generation bioenergy crops have significant potential to address climate change when the CO2 emitted during the biorefining process is captured and sequestered, ultimately achieving carbon-negative biofuels (<−22 gCO2 per MJ).55,56 Decentralized biorefineries have been proposed as a means of utilizing disperse, low-density biomass feedstocks, but the relatively small scales of operation pose a challenge due to the lack of economies of scale. Conversely, large, centralized biorefineries are challenged by high feedstock costs. Techno-economic modeling indicates that carbon-negative biorefineries should aim for biomass supply rates of ∼2000 metric tons per day.56 Unlike ethanol fermentation, the CO2 from anaerobic digestion of biogas requires purification prior to compression and sequestration. Traditionally, biogas is utilized in one of two ways: direct combustion or upgrading followed by combustion. Anaerobic digestion produces both hydrogen and methane. Both are valuable energy products, but the methane portion is preferred for electricity generation. Biogas destined to be pipeline-ready biomethane, or renewable natural gas (RNG), must undergo an upgrading process wherein CO2 and H2S are removed via a separation process.57 Pressure swing adsorption, chemical absorption, water scrubbing, and membrane separation are the leading technologies used for biogas upgrading, with water scrubbing being most common method due to its low cost and high efficiency.58,59 However, water scrubbing is not the best choice for high purity CO2 production. New swing adsorption technologies provide flexible load operations, high energy efficiency, and low capital costs, relative to baseline systems.60 However, sulfur containing species reduce efficacy and increase cost. Biological treatments are emerging as a low-cost method for removing H2S before the aforementioned non-biological upgrading methods. In particular, chemo- and photo-trophic methods of biofiltering, biotrickling, and bioscrubbing show promise for sulfur contaminate removal.61 Moving forward, continued advancements are needed in the modularization of biogas upgrading systems to enable low-cost CO2 capture from small AD systems.60 Small scale biogas upgrading systems could enable the purification and sequestration of regionally diffuse biogas sources from readily available organic wastes.62 Methane leakage from anaerobic digestion systems is a great concern due to the high global warming impact of methane, relative to CO2, and therefore must be tightly regulated as biomethane production increases in a growing bioeconomy. Dark fermentation is an emerging method of anaerobic digestion wherein CO2 and H2 are the primary products, thereby eliminating the risk of methane leaks.63 For both biochemical and thermochemical pathways, initial deployment efforts should focus on sustainable feedstocks from marginal agricultural lands or existing waste materials to minimize transport costs and avoid indirect land use change.64 In addition, near-term siting of biorefineries should prioritize regions with suitable geology for permanent CO2 sequestration, such as the Illinois basin, Gulf region, and western North Dakota in the United States.65
Bioplastics
Polymers and plastic materials are ubiquitous in modern life and store significant quantities of carbon for extended periods of time, albeit the majority of such carbon is derived from fossil resources. Specifically, over 380 million metric tonnes of plastic are currently produced globally each year, storing over 285 million tonnes of carbon with an emissions potential of over 1 billion tonnes of CO2 (assuming plastics are 75 wt% carbon on average).66,67 The production of plastics is expected to reach over 1 billion metric tonnes by 2050 each year, storing over 750 million tonnes of carbon with an emissions potential of over 2.75 billion tonnes of CO2.66 Traditionally, there have been four end-of-life applications for plastics: landfilling, incineration, recycling, and littering. Less than 10% of plastics are recycled, leaving the majority of the plastic-carbon either being landfilled, incinerated, and littered.68–70 Over the past several decades, a variety of bioplastics have risen to industrial relevance to help reduce the use of fossil carbon resources and associated emissions during refining and at end-of-life. As shown in Table 1, a significant fraction of the leading bioplastics are biodegradable, meaning the carbon is biologically released/decomposed as CO2 or CH4 in natural or controlled environments, with the remainder being relatively inert.71,72
Table 1 Relative market share of bioplastics. Adapted from European Bioplastics72 PE = polyethylene, PA = polyamide, PTT = polytrimethylene terephthalate, PET = polyethylene terephthalate, PP = polypropylene, PEF = polyethylene furanoate, PBAT = polybutylene adipate terephthalate, PLA = polylactic acid, PBS = polybutylene succinate, PHA = polyhydroxyalkanoates
Bio-based/non-biodegradable |
% of total |
Biodegradable |
% of total |
PE |
9.5 |
PBAT |
19.2 |
PA |
9.1 |
PLA |
18.9 |
PTT |
8.1 |
Starch blends |
16.4 |
PET |
6.2 |
PBS |
3.5 |
PP |
1.9 |
Cellulose films |
3.2 |
Other |
1.0 |
PHA |
1.8 |
PEF |
0.0 |
Other |
1.2 |
Currently, bioplastics make up less than 1% of the global plastics market, however that is expected to increase significantly if the plastics industry is to achieve net-zero emissions by 2050.71 Bioplastics face similar limits to scale as other bioproducts in that feedstocks often compete with land for food production and will also compete directly with other uses of biomass.73 Moreover, fossil polymer production systems are highly optimized and generally low-cost making many biopolymer options uncompetitive with current technology and infrastructure. Still, industrial systems models have been utilized to highlight the potential of removing over 1 billion tonnes of CO2 per year via bioplastic production in the year 2050, thereby justifying increased research and development into the development of carbon-negative bioplastics.66 Based on recent trends, the global bioplastics industry is expected to grow by 216% between 2021 and 2026, thereby providing strong evidence that bioplastics will likely be at a significant scale by mid-century.72
There are two main pathways for bioplastic-enabled carbon removal: (1) capture and sequestration of gaseous carbon at end-of-life decomposition or (2) long term carbon storage in the product itself. Option 1 is the only viable pathway for carbon removal with biodegradable and compostable bioplastics, whereas both options are viable for carbon removal with inert bioplastics. In 2021, the five most popular bioplastics represent 73% of production and include PBAT, PLA, starch blends, PE, and PA, of which PE and PA are the only non-biodegradable, inert bioplastics (Table 1).72 Traditionally, biodegradable bioplastics have been preferred over inert due to concerns over pollution and accumulation in the environment and associated effects on marine and terrestrial ecosystems. However, inert bioplastics are more amenable to carbon removal and thus climate change mitigation. Currently, bioplastic production does not prioritize carbon removal, with the majority of biocarbon entering the atmosphere as methane or CO2 at end-of-life via degradation in landfills, compost, and energy recovery. Composting of bioplastic has gained significant attention due to its production of value-added soil amendment, but the majority of carbon in the bioplastic is lost as CO2via respiration.74 Notably, cradle-to-grave LCAs have shown that the carbon intensities of certain bioplastics with end-of-life composting are higher than their petroleum-derived counterparts, particularly for bioplastics made from land-intensive feedstocks such as corn starch.75 The carbon intensities associated with bioplastics can be decreased with high solids compositing techniques that limit microbial respiration, and should be investigated further.76 Negative carbon intensities were shown to be possible with the production of PLA from waste biomass feedstocks and landfilling at end-of-life, although the permanence of the sequestered carbon was unclear.77
PE has an increased potential for carbon removal relative to other bioplastics for a variety of reasons, including its composition that is resistant to microbial degradation, the opportunity for CO2 capture during its manufacturing process, and the existing fossil-PE market of which it can serve as a drop-in replacement. Specifically, the lack of oxygen in PE makes it highly inert and an ideal material for carbon sequestration. The manufacturing of bio-based PE requires upstream ethanol fermentation, thereby offering an opportunity for low-cost CO2 capture from the fermentation vessels. Finally, PE is the most popular plastic in the world, representing 31% of global demand, thereby offering an opportunity for carbon removal at significant scale. Specifically, over 100 million tonnes of primary PE are produced each year, equivalent to 86 million tonnes of carbon. Thus, the current PE market demand has the theoretical potential to remove 315 million tonnes of CO2 if all carbon is derived from biomass.78 Moreover, the intermediate chemical ethylene, which is also made from ethanol and used in a variety of different polymers, has a global demand of 200 million tonnes per year, equivalent to 630 million tonnes of CO2 if all carbon is derived from biomass.79,80
The majority of bioplastics currently on the market are indirectly derived from CO2 in the form of biomass feedstocks rich in carbohydrates or lipids, such as sugarcane, corn starch, and soybean oil, or cellulose, such as pine and poplar. Recently, there has been significant interest in producing plastics directly from CO2 using novel biological or chemical routes, which reduces the use of land-intensive biomass feedstocks. A particularly promising route for producing PE in a sustainable fashion with minimal impact on land use and food crops is via fermentation of CO2 and H2 into ethanol with subsequent conversion to PE. Advancements in genome engineering of acetogens with tools such as CRISPR-Cas9 have enabled cost competitive fermentation of CO2 into ethanol, thereby warranting serious attention from decision makers interested in decarbonization.81 Unlike PE, which can be synthesized using a variety of biological substrates other than CO2, several bioplastics require CO2 for synthesis. Fig. 4 illustrates representative direct and indirect polymer production from CO2.
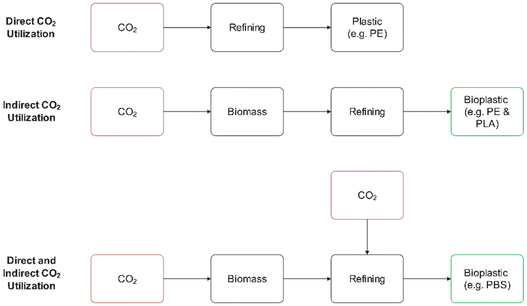 |
| Fig. 4 Indirect and direct utilization of CO2 for polymer production. PE = polyethylene, PLA = polylactic acid, PBS = polybutylene succinate. | |
For example, the primary metabolic pathways used by industrial microbes for producing succinic acid, the intermediate biochemical to polybutylene succinate (PBS), require CO2. PBS is on track to be the 2nd highest demand bioplastic by 2026, meaning that significant quantities of CO2 will be required for synthesis.72 Notably, PBS synthesis typically involves co-fermentation of carbohydrates with CO2, thereby still requiring biomass feedstocks. A recent cradle-to-grave LCA study demonstrated the potential carbon-negative PBS production when using wheat straw and miscanthus as biomass feedstocks for fermentation and land management practices that promote soil carbon sequestration.82 Another LCA study assessed a multitude of the most common bioplastics and determined that PBS and PE had the lowest carbon intensities.83 Similar to PBS, biomass-derived polycarbonates require CO2 as a reagent in synthesis. Polylimonene carbonate is particularly intriguing since it requires the catalytic reaction of biomass-derived limonene with CO2 gas and can serve as a direct replacement to fossil-derived poly(propylene carbonate).84 Carbon-negative polylimonene carbonate production has been demonstrated to be possible with direct mineralization of CO2 gas and use of waste biomass for energy generation in the process.85 Notably, the chemistry of poly(limonene carbonate) can be modified quite easily, thereby presenting an opportunity to use this CO2-derived material as a platform polymer in the production of many functional materials. Currently, the TRL of bio-based poly(limone carbonate) is still relatively low, and thus there is a need for continued innovation and large-scale demonstration. As the technology matures, LCA modeling will be needed to quantify and compare its carbon intensity with other, higher TRL, bioplastics such as polyethylene and polylactic acid.86–88 Finally, polyhydroxyalkonates (PHAs) are an emerging class of bioplastic with tunable properties that might make them more amenable to carbon sequestration. Microbes directly synthesize the PHAs from various carbon substrates, including biomass-derived compounds and air-derived CO2, and genome engineering holds the potential to enable tailored bioplastics.89,90
Moving forward, research on carbon-negative bioplastics must involve thorough and reliable LCA methodologies to ensure reported carbon intensities are realistic. Inconsistencies in LCA methodologies have been identified in the accounting of biogenic carbon in the bioplastic materials. End-of-life distinction between biogenic and fossil carbon in the bioplastic must be made to enable accurate cradle-to-grave CI values, particularly when recycling is involved.83 In addition, dynamic accounting of biogenic carbon indicates that rapid biomass growth and harvest cycles are required to ensure beneficial climate impacts; thus, the traditional assumption of carbon neutrality without time consideration may no longer be defensible. However, dynamic accounting is less important if biomass waste materials or sustainable, highly productive crops with short rotations are used. Overall, LCA modelers must be transparent and consistent in the methods used to account for biogenic carbon when quantifying the carbon intensity of a particular bioplastic.83
Biochar
Biochar is a carbon-rich, highly porous, and solid material produced from pyrolysis where biomass is thermally treated at 400–700 C in the absence of oxygen. Biochar products have been developed for a multitude of different applications, including adsorbents, catalyst supports, soil amendments, electrodes, carbon fibers, and many more (see Fig. 5). Importantly, biochar is highly inert and thus offers an opportunity for carbon sequestration.91 Activated biochar products, often referred to as activated carbon, undergo physical or chemical activation after pyrolysis to enhance physiochemical properties, such as specific surface area and functionality.92 Activated biochar products primarily include adsorbents, catalyst supports, and electrodes and are used for many applications, including environmental remediation, heterogenous catalysis, CO2 capture, and energy storage, to name a few.92
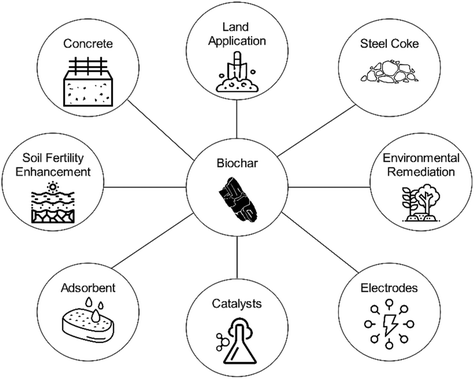 |
| Fig. 5 Biochar product applications. | |
Regarding CO2 capture, biochar is a unique material that has the potential to capture and store atmospheric carbon in two different ways: (1) in the structural make-up of the char via carbonized biomass and (2) on the surface of the char via CO2 gas adsorption. Biochar composition and textural properties can vary widely and are critical predictors of performance as an adsorbent.93 Activation of biochar via chemical treatment, including amine functionalization, can provide high-performing CO2 adsorption/desorption materials (∼5 mmol per gram at 1 bar and 25 C). However, there is a significant lack of data demonstrating durability and recyclability of activated biochar for CO2 adsorption at large scale and with representative flue gas streams.94,95 Activated biochar is also highly effective as adsorbing other compounds of interest, particularly inorganic nitrogen and phosphorous from wastewater and agricultural runoff. Notably, the mechanisms involved in N and P adsorption to biochar differ, with N involving ion exchange and electrostatic adsorption and P involving surface deposition and precipitation with metallic compounds. In addition, fluctuations in pH have the potential to significantly affect N & P adsorption. Thus, activated biochars for nutrient removal from wastewater often require custom design for optimal performance, which increases cost.96 Biochar has been shown to be a beneficial additive to anaerobic digestion processes through increased biogas productivity. Specifically, biochar provides micropore habitats for robust growth, buffer capacity for stable operation, and electrical conductivity for enhanced electron transfer.97 Electrodes for energy storage are an emerging class of biochar products that have the potential for large scale carbon sequestration. Electric vehicles and modular stationary energy storage systems have created a fierce demand for lithium-ion batteries, which is in turn causing a dramatic increase in demand for graphite anode materials.98,99 Recently, several new methods of catalytic pyrolysis have been developed to convert biomass into battery-grade biographite.100–106 Other ion batteries, such as sodium and potassium, typically also require carbon anodes, thereby providing another market for biochar electrodes. In addition, energy storage devices based on capacitance, such as supercapacitors, are typically comprised of activated carbon. The energy storage industry is growing rapidly, and more attention is warranted towards using biomass precursors to develop carbon-negative energy storage devices. Carbon fibers are used in high performance, high strength, lightweight materials for structural supports in applications including transportation, athletics, and buildings. The incumbent carbon precursor used in carbon fibers is polyacrylonitrile, which is expensive and derived from fossil carbon precursors. There is growing interest in using biocarbon precursors such as lignin and bio-based PAN for carbon fiber synthesis.107,108 The multi-stage process of carbon fiber synthesis involves pyrolysis around 1000 C, thereby classifying biocarbon fibers as a form of biochar product. Lignin carbon fibers have been under development for several decades, but have yet to reach commercial adoption due primarily to high costs and poor performance.109 Moving forward, lignin carbon fiber products should be tailored for applications that require relatively low strength and performance properties. A recent innovation has enabled the production of PAN from biocarbon precursors, thereby offering an opportunity to produce a drop-in PAN carbon fiber material derived from sustainable biomass.110 The process of producing bio-based PAN carbon fibers is energy-intensive, and thus thorough life cycle assessment is still required to understand the potential for carbon sequestration.109,111
All of the aforementioned biochar products and applications have the potential to create value and sequester atmospheric carbon, but not at any appreciable scale (<0.5 GtCO2 per years).92 However, there is significant potential in carbon sequestration via application of biochar to soils (0.5–2 GtCO2 per years).16 In addition to carbon sequestration, biochar offers of a multitude of benefits to soil health including increased biological activity and organic carbon accumulation, reduced runoff, increased crop productivity, and reduced nutrient leaching.112 Also, recent evidence shows the ability of biochar to reduce N2O emissions from soils, which is important given the strong global warming impact of N2O.
Specifically, a meta-analysis showed that biochar application leads to an average reduction of ∼50% in soil N2O emissions across laboratory and field trials, particularly in sandy soils. Notably, reductions in N2O emissions have been found to diminish with time, and thus further research is needed to better understand this dynamic.113 Although N2O emissions reductions are highly beneficial, the majority of long-term benefits from biochar application to soils are in the form of carbon sequestration. The extent of carbon storage durability for particular combinations of biochars and soil types is a major research gap that requires continued investigation. Nonetheless, there has been significant progress in understanding biochar durability in soils over the last 5–10 years. Through meta-analysis, the rate of biochar decomposition was found to vary significantly with experimental duration, feedstock, pyrolysis temperature, and clay content.114 On average, over 95% of biochar mass results in long-term carbon sequestration of greater than 500 years. Biochar application has a substantial effect on soil microbial activity, particularly for sandy soils where the mineralization of soil organic matter has been shown to increase by 20% with application of biochar. Also, crop-derived biochar, fast pyrolysis, low pyrolysis temperature, and small application amounts all had negative soil priming effects, meaning the SOM degradation rates of the soils are reduced upon application.114 Upon initial application of biochar there is a relatively rapid increase in CO2 emissions from the labile carbon followed by a reduction in priming.115 Overall, the stability of carbon in biochar is proportional to the temperature used during pyrolysis. Regardless of feedstock, temperatures in the range of 500–700 C were found to be optimal for carbon stability.116 Highly stable biochars are achievable at lower temperatures with other process modifications, including extended reaction times, pressurized reactors, and feedstocks with high initial lignin contents.
Typically, highly stable biochars are produced in relatively low yields.116 The optimal composition of biochar for soil carbon sequestration was found to have an O/Corg ratio < 0.2 and H/Corg ratio < 0.4.115 Notably, particular biomass feedstocks and pyrolysis conditions, excessive application of biochar, as well as other variables can decrease soil health, thereby warranting more research into elucidating these complexities.114 Specifically, potential negative impacts of biochar application to soils include suppression of soil nutrient availability and crop productivity, reduction in carbon mineralization, and accumulation of polycyclic aromatic hydrocarbons (PAHs), polychlorinated dibenzodioxins, and dibenzofurans (PCDD/DF).117 Excessive application of biochar, exceeding 72 tonnes per hectare, has been found to decrease maize and wheat grain yields by 46 and 70%, respectively. The reduced crop productivity was due to immobilization of nutrients, most notably nitrogen.117 Fast pyrolysis biochar was found to immobilize substantially more nitrogen than biochar made via slow pyrolysis. Pyrolysis reactors that do not sufficiently separate tars and vapors produce biochar products saturated in polycyclic aromatic hydrocarbons, which have the potential to negatively affect soil and human health. Thus, standards for allowable PAH concentrations in biochar products must be established to ensure producers are using the appropriate pyrolysis technologies and avoiding the risk of PAH contamination.117 A thorough risk assessment of biochar application to soils for carbon sequestration determined the majority of risk is not present in the feedstock variability, supply chain logistics, or pyrolysis process scale-up, but rather in the ability to predict and monitor the carbon sequestered in soils.
In summary, permanence of biochar carbon in soils is highly variable and new tools must be developed to cost-effectively monitor and verify soil carbon sequestration. Precision agriculture tools that rely on robotics and remote sensing have the potential to address the issue of permanence in soil carbon sequestration.118 In addition, LCAs of biochar for soil carbon sequestration must prioritize multiple impact categories. Global warming potential is often the impact category of sole interest. A recent review indicates a small but growing body of research that includes other impact categories of import such as abiotic depletion, eco-toxicity, and human toxicity, among others.119 Given the diversity of biochar feedstocks and processes, it is essential that these important categories be included alongside climate impacts when assessing the impacts of soil amendments. Positive benefits of biochar application to soils, including moisture retention and reduced irrigation, are captured in abiotic depletion. Conversely, the negative impacts of incorporating polyaromatic hydrocarbons (PAHs) into soils will be captured in eco-toxicity and human toxicity.120
Wood products
The wood products industry removes significant quantities of atmospheric carbon in the form of long-lived products each year. A summary of products is shown in Fig. 6. In the US, over 100 million tonnes of CO2 are removed and incorporated into wood products each year.121 Thorough assessments have demonstrated that wood products emit considerably less greenhouse gases over their lifetime relative to their non-wood (e.g. metal or plastic) counterparts.122 For example, a standard new house has the potential to sequester 17.5 tonnes of CO2 if lumber, oriented strand board (OSB), and plywood are utilized.122 The use of wood in place of non-wood materials reduces lifecycle emissions by an average of 3.9 tonnes of CO2 for each tonne of dry wood used.123 Many wood product systems models have been developed to track forest carbon accumulation, harvesting, processing, and distribution, but few models accurately account for end-of-life carbon emissions, thereby warranting more research into wood product degradation in different environments.124 The degradation of a variety of wood products, including hardwood, softwood, oriented strand board (OSB), plywood (PW), particleboard (PB), and medium-density fiberboard (MDF), was carried out under landfill conditions over a 2.5 years period, from which exposed holocellulose content was determined to be readily degradable, relative to lignin, thereby highlighting the importance of lignin content for carbon permanence in wood products.125
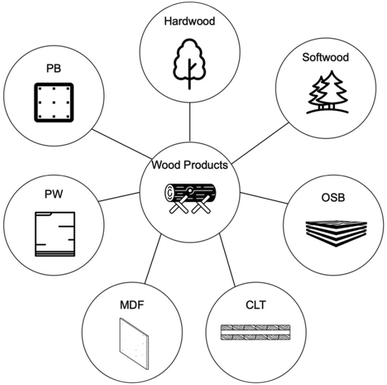 |
| Fig. 6 Harvested wood products for carbon storage. OSB = oriented strand board, CLT = cross-laminated timber, MDF = medium-density fiber board, PW = plywood, PB = particle board. | |
Multiple studies have demonstrated the recalcitrance of lignin in landfills, while the rate and extent of holocellulose decomposition are dependent on the type of wood product and its physical form (e.g. particle size and surface area).126 A detailed assessment of the forest products industry in Portugal using two different carbon accounting methods determined that net negative emissions are possible with long product lifetimes and sufficient landfill management practices.127 However, further research is required to create reliable permanence data for a variety of wood products under various end-of-life conditions, particularly for life cycle modeling where there is significant uncertainty in permanence of carbon in wood products at end-of-life.128 Notably, optimizing forests for long-term carbon storage typically involves harvesting of biomass for wood products. Thus, overall, significant reductions in forest biomass harvesting generally do not maximize carbon storage.129–131 Accumulation of forest residues with minimal removal can lead to negative environmental and social impacts including intensified wildfire severity and probability.132,133 Thus, more effort into the sustainable removal of forest residues for valorization and carbon storage is encouraged.134
Dimensional lumber and OSB have been shown to be significant sinks of carbon over their life cycle when made from forest residues, as opposed to virgin timber.135 Most lumber products have been shown to reliably store 50% of their carbon for at least 100 years, thereby providing significant potential for carbon removal.136 Notably, a ∼10% improvement in wood mill efficiency has been shown to decrease waste and increase the quantity of carbon sequestered in lumber products by ∼7%.137 However, there is considerable variation in the composition and permanence of lumber products, therefore requiring detailed assessments for each product and avoiding generalization. For example, alkaline copper quaternary (ACQ) treated lumber and wood plastic composite (WPC) are two common lumber materials used in decking, but the emissions associated with former over its life cycle are roughly 30% of the emissions of the latter.138 In addition, borate-treated lumber was found to generate 1.8 times lower GHG emissions and use 83 times less water than galvanized steel framing members (the closest non-wood competitor).139 Other emerging engineered wood products with significant carbon storage potential include cross-laminated timber, glulam, laminated veneer lumber, parallel strand lumber, and mass plywood panels.140
Globally, the life cycle of pulp and paper products represent 1.3% of greenhouse gas emissions, and there is considerable potential to reduce emissions with end-of-life carbon management and storage.141 Notably, recent research indicates that increasing the rate of pulp and paper product recycling will not necessarily reduce emissions due to the high quantity of fossil fuel consumption in the recycling pulping process, compared to the chemical pulping of virgin biomass wherein the vast majority of fuels consumed for energy are bio-based.142 Thus, implementing the concept of circularity with heavy reliance on recycling may not reduce emissions in the pulp and paper sector, warranting further research. Landfill practices have been found to be especially important in reducing emissions from the pulp and paper sector, given that approximately 100 tonnes of waste are landfilled per 550 tonnes of pulp produced. There is significant potential for capture and sequestration of the biogenic carbon emitted from paper waste at landfills.143 In addition, there is considerable potential to capture and sequester biogenic CO2 emitted at chemical pulp mills. In the US, the pulp and paper industry is the largest consumer of biomass for stationary heat and power production, emitting ∼115 million metric tons of biogenic CO2 each year. There are intriguing opportunities to integrate CO2 capture into the lime kiln operation and to utilize CO2 for pH adjustment and lignin precipitation. Also, the alkaline chemistry of the kraft pulping process lends itself well for CO2 capture, thereby offering the opportunity for in situ CO2 capture in the recovery boiler.43,144
Emerging biocarbon products
The aforementioned biocarbon products and associated industries are relatively mature and of high technology-readiness-level (TRL > 7). There are several new biocarbon products emerging that have significant potential to sequester carbon, with steel and cement that incorporate biogenic carbon in the production process holding particularly impactful potential. The manufacturing of iron and steel represents ∼7% and cement and concrete ∼6% of global energy system combustion and industrial process CO2 emissions, and traditional low-carbon energy technologies, such as solar and wind, are not suitable for full decarbonization of these industries.145 Traditional manufacturing of virgin steel relies on carbon-rich petroleum- or coal-derived coke for iron ore reduction in the blast furnace. Recently, process modeling has shown the techno-economic feasibility of using pyrolysis-derived biocoke, which is similar to biochar, or gasification-derived biohydrogen as substitutes to fossil coke in the blast furnace.146,147 Preliminary cradle-to-grave life cycle assessments indicate net negative carbon emissions (−0.1 to −0.5 t-CO2 per t-steel) is feasible with capture of the CO2 from the blast furnace and other steel making operations.146 Notably, a small percentage (0.2–2.0 wt%) of the final steel product is carbon from the blast furnace, thereby offering another avenue for carbon sequestration. Carbon is intentionally incorporated into the steel to provide specific strength properties. Although the weight percentage of biocarbon in the final steel product is small, the large quantities of steel produced each year (∼2 billion tonnes of steel) justify its consideration for carbon removal; note that steel produced from virgin iron ore and recycled scrap both require the incorporation of carbon.
Similar to steel, the incorporation of biochar carbon into cement mixes as aggregate and curing agents has been shown to provide desirable strength properties. When 1% weight of the concrete mix is replaced with biochar additive filler, the compressive strength of the concrete improves, the flowability, static elastic modulus, drying shrinkage, and flexural strength are not significantly impacted, and permeability decreases.148,149 Notably, inclusion of <1% biochar to global concrete production has the potential to sequester 0.5 Gt of CO2 per year.149 Prior studies show that biochar dosages of <3 wt% generally do not negatively impact concrete strength properties,150,151 with more recent findings indicating biochar dosages of greater than or equal to 10 wt% may be feasible.152–155 Based on prior and ongoing studies, there is promising potential for biochar-cement composites in various applications, including structural usage.154,155 In addition, the use of biogenic or atmospheric CO2 in the concrete curing has the potential to significantly reduce emissions and achieve negative carbon intensities.156,157
Assessment of drawdown potential
In the second part of this paper, we demonstrate the potential of four representative BiCRS products to facilitate carbon drawdown. Estimating the carbon drawdown potential of BiCRS products necessitates reliable estimates of the magnitude and durability of sequestered carbon. We analyze an advanced BECCS pathway, bio-polyethylene, oriented strand board (OSB), and biochar soil amendment. We first present estimates for the durability of carbon in geologic reservoirs, durable products, and biochar soil amendments over a 10
000-year time horizon, while acknowledging the shortcomings of assessments over such long horizons. We then estimate the life cycle GHG emissions of the four products, including a discussion of the long-term drawdown potential of each pathway. Finally, we discuss the relevance of this analysis to long-term climate mitigation goals, future research directions, and supportive climate policy.
Results part 1: durability of sequestered carbon over time
Biogenic carbon can be sequestered in engineered sinks for climate-relevant timescales. The durability of sequestration can vary from days to millennia. We estimate optimistic, moderate, and pessimistic bounds for sequestration in onshore and offshore geologic reservoirs, polyethylene products, oriented strand board (OSB), and biochar soil amendment. The estimates should not be understood as a statistical likelihood of a given outcome but rather a plausible range of outcomes. The estimates reported here are the result of the synthesis of the best available data. Representative cases are selected for the main text, while the full range of analyzed scenarios is presented in greater detail in the ESI (see S1, ESI† for methods specific to each pathway).
Geologic sequestration
Geologic sequestration of CO2 is the most secure form of sequestration analyzed here, serving as a baseline for comparison to other modes of sequestration. Here, CO2 is compressed into a supercritical fluid and injected into deep geologic formations. Injected CO2 is trapped in porous rock beneath an impermeable cap-rock formation through buoyancy, adhesion, solubility, or mineralization.158 Leakage can occur through structural failure of the caprock or well or from unidentified and improperly abandoned wells proximate to the storage project.158Fig. 7a illustrates the 5th to 95th percentile range for the fraction of carbon remaining in (globally aggregated) on-shore and off-shore reservoirs (adapted from Alcalde et al. (2018)158). In the least optimistic estimate, >67% of the sequestered CO2 remains in storage after 10
000 years. The remaining fraction of sequestered carbon at 100; 1000; and 10
000 years in onshore and offshore reservoirs is estimated to be within the ranges 0.83–0.99 (0.99), 0.79–0.99 (0.99), and 0.67–0.99 (0.92) respectively. The values in parenthesis reflect the representative case of onshore, well-regulated geological sequestration.
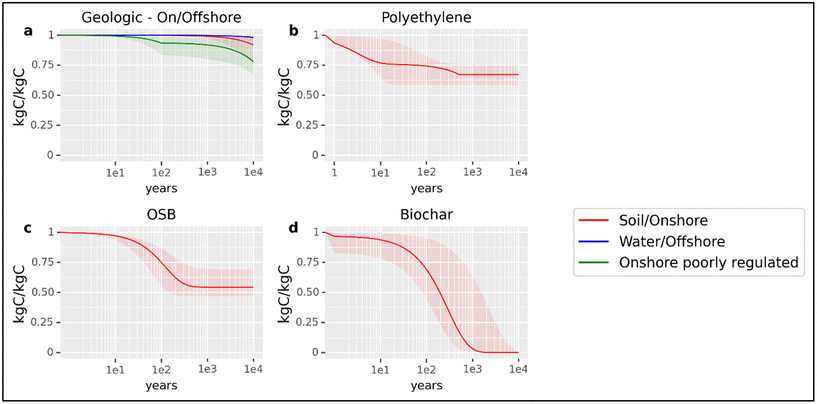 |
| Fig. 7 Estimated carbon sequestration over 10 000 years. This figure illustrates a range of optimistic, pessimistic, and moderate cases for carbon sequestration over time. The dark red line in each panel is the moderate estimate for each analyzed scenario. The dark blue and dark green lines in panel (a) represent the P50 estimate (see S1.1, ESI†) for offshore and onshore poorly-regulated geologic sequestration. The dark red line in panel (a) is the P50 scenario for onshore, well-regulated wells. This is the baseline for geological sequestration in this analysis. The functional form in each case considers a pulse of carbon entering the carbon cycle in the form of a product or sequestration co-product. From the production gate, the function may consider (where appropriate) operational use-life, recycling, secondary use, and sequestration of carbon in the product or biosphere. Panels: (a) Geologic sequestration of industrially captured CO2 in either onshore well-regulated or poorly-regulated or offshore well-regulated reservoirs (b) carbon sequestered in a polyethylene product. Note that the discontinuity and shape of the function results from the interaction of both linear (landfill decay) and exponential (use-life decay) decay assumptions in the function. (c) carbon sequestered in oriented strand board (OSB) construction material (d) carbon sequestered in biochar soil amendment applied to agricultural soil. | |
Carbon storage in polyethylene products
Long-term sequestration of biogenic carbon can also be achieved in thermoplastics such as polyethylene (PE). PE's stable structure and resistance to degradation give it desirable qualities for many commercial uses as well as long-term carbon storage. At the end of its useful life, PE may be recycled, re-used, combusted, landfilled, or discarded. In the U.S. context, most PE is landfilled159 (also see S1.2†), where only a fraction of the degradable carbon will return to the atmosphere. The lifetime of plastics in the environment is not well-understood and estimates vary widely.160 Once in the environment, PE is subject to physical (photodegradation from UV light, thermooxidation, and hydrolysis) as well as biological degradation.161,162 Degradation rates are subject to physical properties such as volume, surface area, and chemical composition as well as environmental factors such as temperature, humidity, pH, and the presence of oxygen.163Fig. 7b shows the optimistic, moderate, and pessimistic estimates for carbon remaining in PE over time based on estimated use-life, recycling and secondary use rates, and a physical decay model for HDPE pipe, HDPE bottles, and LDPE bags in soil. We estimate the fraction of sequestered carbon at 100; 1000; and 10
000 years stored in PE to fall within the ranges 0.58–0.83 (0.74), 0.59–0.76 (0.67), and 0.59–0.75 (0.67) respectively. The values in parentheses reflect the representative case of carbon stored in HDPE bottles.
Carbon storage in oriented strand board (OSB)
There is an extensive literature on the mitigation benefits of storing carbon in long-lived wood products, particularly in buildings.121,123,164 Oriented strand board (OSB) is widely-used as a load-bearing construction material. After its useful life expires, OSB's end-of-life phase may involve recycling, secondary use, and a significant portion may arrive in landfills or open dumps. Fig. 7c shows optimistic, moderate, and pessimistic estimates for carbon sequestered in OSB over time based on estimated use-life, energy reclamation rates, and physical degradation rates for OSB panels in a landfill environment. In all cases, >46% of the OSB carbon is permanently sequestered in landfills. We estimate the fraction of carbon sequestered at 100; 1000; and 10
000 years stored in OSB to be within the ranges 0.56–0.87 (0.75), 0.47–0.70 (0.54), and 0.47–0.70 (0.54) respectively. The values in parenthesis reflect the representative moderate decay rates (use-life and landfill) and degradable organic carbon fraction for OSB.
Carbon storage in biochar soil amendment
Finally, biogenic carbon can be sequestered in biochar as a soil amendment. We rely on published estimates of labile and recalcitrant carbon fractions114 and mineralization rates165 for biochar in soils. Physical characteristics of the biochar and feedstock as well as environmental factors such as precipitation and soil conditions influence biochar stability; as such, there is a large degree of uncertainty in the durability of sequestration.166–168Fig. 2d illustrates optimistic, moderate, and pessimistic estimates for biochar carbon remaining sequestered in soils over time. We estimate the fraction of sequestered carbon at 100; 1000; and 10
000 years stored in biochar in soils to be within the ranges 0.44–0.95 (0.69), 0–0.64 (0.03), and 0–0.01 (0) respectively. The values in parenthesis reflect the representative case.
Results part 2: drawdown in products
The carbon physically stored in bio-based products is only half of the story. Net carbon removal must also account for the full life cycle of production prior to final end-of-life. The four BiCRS pathways explored here are representative but not exhaustive in terms possible production methods. They were selected for near-term viability and to represent a variety of second generation (cellulosic) feedstocks (see S2.2† for details), conversion methods, and end-uses. After estimating cradle-to-gate life cycle emissions, the moderate case 100-year sequestration durability estimates (from Results Part 1, fully derived in the ESI† for each technology) are applied to calculate cradle-to-grave net carbon removals or emissions, as the case may be. We estimate the 1000 and 10
000-year emissions profile for each pathway in the ESI (S2.3.2, S2.4.2, S2.5.2, S2.6.2).† We apply 100-year global warming potentials (GPW) to non-CO2 emissions, expressed as CO2 equivalents or CO2e.169 This decision amplifies the relative climate impact of emissions that occur late in a project's lifetime (e.g. landfill emissions) when considering the 100-year time horizon, causing the estimates of net carbon removal presented here to be conservative within the GWP framework (see S2.1.1, ESI†).170 Dynamic life cycle assessment methods170 can be used to account for these temporal discrepancies, but for the illustrative purposes here, we focus on the physical carbon drawdown rather than assessing the benefits of delayed impacts over a fixed time horizon. The temporal impact considerations are out-of-scope and would only serve to enhance the apparent climate benefits of pathways that delay release of stored carbon (CO2 emissions occurring near year 100 would approach zero impact). This is a distraction from the nominal carbon removal estimate we are after. The life cycle model assumptions for each of the four pathways are described in greater detail in S2.3–S2.6 in the ESI.†
Goal, scope, functional unit, and system boundaries
The goal of this analysis is to quantify the carbon removal potential of various products in the bioeconomy. The scope of the analysis is cradle-to-grave net carbon removal at 100, 1000, and 10
000 years. Carbon durability over millenia is uncertain and speculative, thus here we focus on the 100-year time horizon. We report our estimates for 1000 and 10
000 years in the ESI.† The functional unit for this analysis is “per metric tonne of carbon in biomass feedstock”. This approach allows us to compare the carbon removal efficiency and resulting product outputs of different product categories on a consistent basis.
The system boundary for our analyses includes feedstock production/collection, feedstock transport, production of biomass product, and product end-of-life. Two BiCRS pathways considered here generate co-products alongside the primary product. The corn stover to polyethylene pathway generates excess electricity which is assumed via system expansion to displace average regional electric grid emissions. The oriented strand board pathway also generates a small quantity of wood residues. The quantity is small and thus we made a simplifying assumption that these materials are combusted to support process heat needs. The potential displacement effects of primary product outputs are not considered in the life cycle GHG assessment. Consistent comparison between the variety of products analyzed here would be challenging and perhaps misleading. We instead offer GHG estimates for incumbent products which might be replaced in the sections below without factoring those avoided emissions into the quantitative analysis.
Feedstock selection
The BiCRS pathways analyzed in the sections that follow utilize cellulosic wastes, residues, and purpose-grown energy crops as feedstocks. These feedstocks avoid or at least minimize sustainability challenges associated with food-crop feedstocks (e.g. corn) that compete for land, water, and nutrients with the food sector. In the case of wastes and residues, the upstream life cycle emissions associated with these feedstocks are minimal, limited to the activities of collection and transport because typically environmental impacts associated the production of the primary product are allocated to the primary product rather than wastes and residuals. Purpose-grown energy crops such as switchgrass, poplar, and miscanthus have received attention because they can potentially generate high yields on marginal lands not suitable for agricultural production. Table 2 below describes the feedstocks considered in this analysis.
Table 2 Cellulosic feedstocks considered in this analysis
Feedstock |
Assumed carbon % (dry basis) |
Description |
Switchgrass |
46.6% |
Switchgrass is a fast-growing perennial crop that can generate high yields in diverse environments, including marginal lands unsuitable for conventional agriculture.171 This is especially beneficial since limited land resources and competition for food production are key challenges for scaling up biomass production for carbon drawdown. |
Corn stover |
46.6% |
Corn stover is agricultural residue consisting of leaves, stalks, and cobs left over after harvest. As much as half of corn crop yield consists of stover residues. Agricultural wastes/residues have the advantage of not requiring additional land for cultivation. Most of the resources have already been expended to produce the primary agricultural good. The wastes would otherwise degrade in situ, releasing a significant portion of their carbon back into the atmosphere. |
Forest residues |
50.3% |
Residues consist of the unmerchantable wood left over from logging activities in managed forests. Transport of residues presents logistical challenges.172 When it is not cost-effective to transport or utilize residues, they may be burned onsite or left to decompose. |
Bioelectricity from Switchgrass with CCS
The selected BECCS pathway considers an integrated gasification combined cycle (IGCC) power plant with carbon capture and sequestration (CCS) in geologic storage. Switchgrass cultivation, the IGCC facility, and geologic carbon storage are all assumed to take place in California, USA. There are suitable conditions for switchgrass cultivation throughout the state.173 The feedstock is assumed to travel an average of 100 km by heavy diesel truck to the IGCC facility. At the IGCC facility, gasification of switchgrass generates syngas—a mix of hydrogen, carbon monoxide, and CO2. Carbon monoxide is converted to additional CO2via the water–gas shift reaction.174 CO2 is separated from the syngas mix before the remaining gas is combusted for electricity production. We model pre-combustion capture of CO2 after physical scrubbing with a methanol-based system as described in an analysis of a coal slurry IGCC system.175 There are suitable formations for geologic carbon sequestration throughout California, and it is assumed that the IGCC facility is located proximate such that additional compression outside of the plant boundary is unnecessary to deliver supercritical CO2 to subsurface storage. CSS system operation causes a 22% relative drop in plant efficiency in order to achieve an 85% capture rate. The separation of the high-purity CO2 stream prior to combustion offers a more cost-effective option than conventional biomass combustion with post-combustion capture.176 Further details on our IGCC LCA assumptions can be found in the ESI, S2.3.†
The carbon drawdown potential of the IGCC facility with CCS is substantial. The process sequesters 3113 kgCO2 per tC in geologic storage while generating 3124 kW h (11
249 MJ) of electricity for the grid. Once captured and stored, 92% of the carbon remains sequestered over 10
000 years. The life cycle net drawdown of the process is −2811 kgCO2e per tC or −0.90 kgCO2e per kW per h (−0.25 kgCO2e per MJ). For comparison, a Natural Gas Combined Cycle facility without CCS emits roughly 0.40 kgCO2e per kW per h.177
Taking the moderate case for onshore geological sequestration, at 100; 1000; and 10
000 years, 99.9%, 99% and 91% of the original drawdown benefit remains, respectively (see Fig. S3, ESI†). Net drawdown at 100 years is −2811 kgCO2e per tC (Fig. 8).
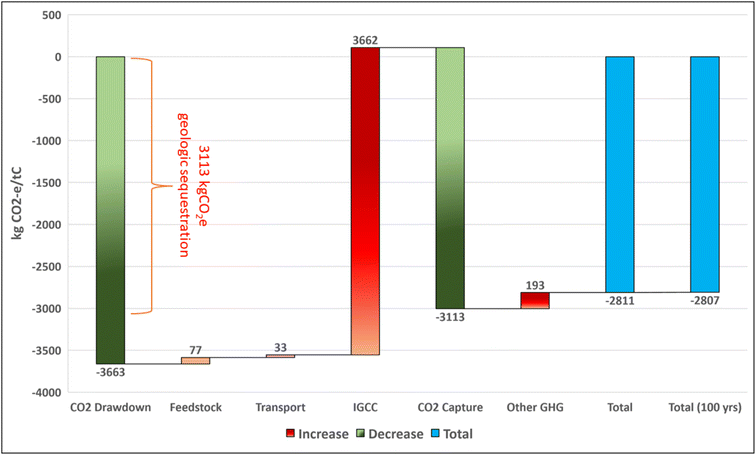 |
| Fig. 8 IGCC-CCS electricity production from switchgrass drawdown over 100 years (moderate case). Note that in the waterfall diagrams, green and red bars represent magnitudes of drawdown and emissions subsequent to the initial drawdown in biomass. The blue bars represent totals. The sum of all red and green bars is equal to the first blue bar. | |
Polyethylene production from corn stover with CCS
Next, we consider conversion of corn stover to polyethylene (PE) with CCS. The process modeled here assumes corn stover is enzymatically treated to make cellulosic sugars available for fermentation. The resulting ethanol is then dehydrated to ethylene intermediate and finally polymerized to PE. Process heat and power for ethanol production is generated via combustion of a fraction of the stover (40%), thus fossil CO2 emissions are avoided at the facility. Subsequent processing into PE is assumed to use regional grid electricity as well as utility natural gas. Collection of corn stover is assumed to take place in Iowa, USA, and the stover travels 2896 km (1800 miles) by diesel rail car to a refinery in California, USA. The fermentation stage generates a high-purity stream of CO2 which can be captured and sequestered geologically at lower cost than combustion streams of CO2.46 As in the IGCC case, the facility is assumed proximate to suitable geologic formations such that additional compression outside of the plant boundary is unnecessary to deliver supercritical CO2 to subsurface storage. Additional biogenic carbon is sequestered in the PE product. Further details on our polyethylene LCA assumptions can be found in the ESI, S2.4.†
The drawdown potential of stover-based PE with CCS is substantial. This pathway sequesters 2159 kgCO2 per tC in engineered sinks while producing 351 kg of polyethylene. 1064 kgCO2 per tC is sequestered in geologic reservoirs while 1102 kgCO2 per tC is sequestered in the PE product as stable carbon. Approximately 92% of the geologic carbon and 67% of the carbon in PE remains sequestered over 10
000 years. The PE carbon losses are emitted by combustion in energy recovery projects or by landfill emissions. The methane in the unflared fraction of landfill emissions is a significant contributor to the reduction of drawdown benefits over time. We estimate the life cycle net drawdown of the process at the gate of the PE resin facility to be −1595 kgCO2e per tC or −4544 kgCO2e per t of PE. Additional emissions arise in our representative case from injection molding to produce HDPE bottles. The net drawdown of the completed product is −1197 kgCO2e per tC or −3410 kgCO2e per t of PE. This compares to an approximate life cycle emission for fossil-based HDPE injection molded products of 2080 kgCO2e per t of PE produced.177
Taking the moderate case which assumes well-managed, flared landfills, at 100; 1000; and 10
000 years, 67%, 53% and 46% of the original drawdown benefit remain, respectively. Assuming no landfill flaring, the benefits fall to 41%, 3.6%, and net emissions at 10
000 years (see Fig. S5 and S6, ESI†). The representative pathway, which assumes methane management at the landfill, retains a net drawdown of −807 kgCO2e per tC at 100 years (Fig. 9).
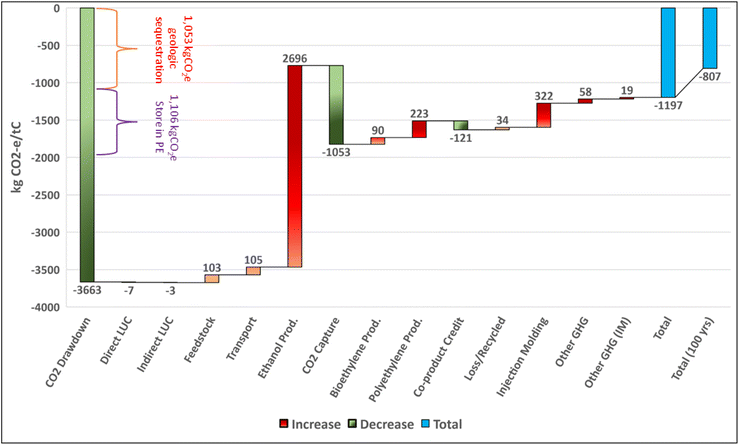 |
| Fig. 9 Polyethylene with CCS drawdown over 100 years (moderate case/flared landfills). Note that in the waterfall diagrams, green and red bars represent magnitudes of drawdown and emissions subsequent to the initial drawdown in biomass. The blue bars represent totals. The sum of all red and green bars is equal to the first blue bar. | |
Biochar from forest residue
We next consider carbon sequestration in biochar as an agricultural soil amendment. Biochar can be a co-product of syngas production (gasification) or bio-oil production (fast pyrolysis), or it can be the primary product (slow pyrolysis). We analyze a simple biochar process using a modified air curtain burner (ACB) to pyrolyze forest management residues. The ACB is a refractory-lined box with a blower that is used for low-emissions incineration but can also be operated to produce biochar (see S2.4 in ESI† for additional details.) External energy requirements include start-up accelerant and fuel for heavy equipment in collection and handling of residues. The portable unit can be set up at remote locations for the management of forest residues which otherwise face logistical challenges to utilization.172 Forest residues and biochar production are assumed to be co-located in Northern California, USA. Biochar is substantially less costly to transport as it is lighter than wood waste and has a higher energy density. It is also preferable to open pile burning from a climate mitigation and emissions perspective.178 Produced biochar is assumed to be transported roughly 129 km (80 miles) by truck from forest site to agricultural soils in the California Central Valley region. Further details on our biochar LCA assumptions can be found in the ESI, S2.5.†
We estimate moderate drawdown potential for biochar on centennial timescales with large uncertainties on millennial timescales. This biochar initially sequesters 1296 kgCO2e per tC in 397 kg of biochar applied to agricultural soils. Over 10
000 years, no carbon remains sequestered in the biochar (see Fig. 7d). We assume all biochar carbon degradation in soils results in CO2 emissions. We do not consider potential biochar sequestration benefits from increased agricultural yields, nor do we consider soil priming impacts. We estimate a net drawdown of −963 kgCO2e per tC or −2426 kgCO2e per t of biochar. Alternatively, the fate of forest residues could be natural decay, open pile burning, or forest fire. Assuming sustainable forest management practices, the alternative fate of onsite combustion would conservatively yield emissions near 0 kgCO2e per tC due to the biogenic nature of the carbon. We do not consider alternative productive uses of forest residues, although there are other possible counterfactuals.
At 100 years, 59% of the original drawdown benefit from biochar application as a soil amendment remains. At 1000 and 10
000 years the process yields net positive emissions (see Fig. S8, ESI†). The pathway retains significant drawdown benefit with life cycle emissions of −564 kgCO2e per tC at 100 years (Fig. 10).
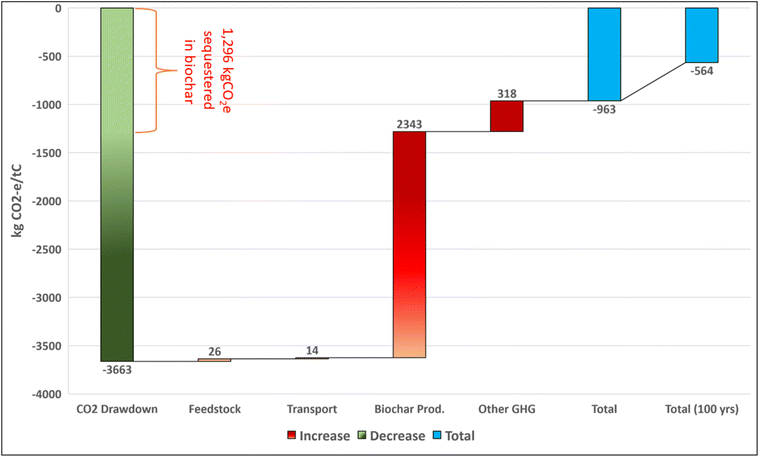 |
| Fig. 10 Biochar soil amendment drawdown over 100 years (moderate case). Note that in the waterfall diagrams, green and red bars represent magnitudes of drawdown and emissions subsequent to the initial drawdown in biomass. The blue bars represent totals. The sum of all red and green bars is equal to the first blue bar. | |
Oriented strand board (OSB) from forest residues
Finally, we assess the conversion of forest residues into oriented strand board (OSB). OSB is a ubiquitous construction material with an estimated North American production volume of 19
885 million ft2 per year as of 2015.179 A standard production unit of OSB is measured at 1000 ft2 at 3/8′′ thickness. One metric tonne of forest residue feedstock will produce roughly 1.3 units (2.58 units per tC) with an estimated mass of 769 kg (1529 kg per tC). We assume forest residue collection in Northern California, USA and transport by heavy diesel truck approximately 145 km (90 miles) to a hypothetical OSB production facility also located in Northern California. Wood strands approximately 2.5 cm × 15 cm are layered at opposing angles and compressed under high temperatures with resin and wax (about 5% by mass)180 to produce a strong construction material. The life cycle of OSB production involves fossil fuels in the collection, handling, and transport of forest residue feedstock. Onsite processes include energy and emissions from flaking, drying/screening, blending, pressing, finishing, and emissions controls. Roughly 90% of the onsite heat requirement comes from wood fuel (about 23% of the feedstock requirement), with the remainder supplied by natural gas, liquified petroleum gas, and fuel oil.181 In addition to wood feedstock, the process uses 25 kg of PF resin, 5 kg of MDI resin, and 11 kg of slack wax per metric tonne of feedstock processed. Further details on our OSB LCA assumptions can be found in the ESI, S2.6.†
The carbon drawdown potential of OSB is substantial. This pathway sequesters 2541 kgCO2e per tC in the OSB product. Approximately 54% of the carbon is eventually permanently sequestered in landfills. The balance of carbon is released when the OSB reaches the end of its functional life, either from combustion in an energy recovery system or as landfill emissions. Methane from the unflared fraction of landfill emissions is a significant contributor to the loss of drawdown benefits over time. We estimate the net drawdown potential to be −1806 kgCO2e per tC or −700 kgCO2e per production unit (−1.18 kgCO2e per kg). A recent meta-analysis estimates an average of 4 tons of CO2e avoided for each ton of dry wood that displaces non-wood materials (assuming similar operation phase emissions), with a middle range of 1.5 to 22.0 tons of CO2 emissions avoided by displacement of non-wood materials per ton of wood carbon employed in building construction (see S2.6.3 for explanation of the reported displacement factors).123
Assuming well-managed landfills that flare emissions, 55% of the original drawdown benefit remains at 100 years (24% if all landfills were unflared), and 11% at 1000 and 10
000 years (net emissions if all landfills are unflared; see Fig. S10 and S11, ESI†). The 100-year net CO2 drawdown of this pathway assuming all landfills are flared is −987 kgCO2e per tC (Fig. 11).
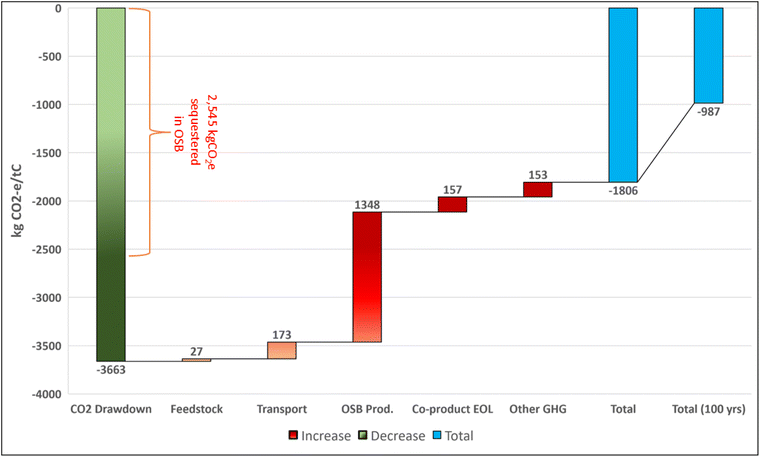 |
| Fig. 11 Oriented strand board drawdown over 100 years (moderate case/flared landfills). Note that in the waterfall diagrams, green and red bars represent magnitudes of drawdown and emissions subsequent to the initial drawdown in biomass. The blue bars represent totals. The sum of all red and green bars is equal to the first blue bar. | |
Pathway comparison
Table 3 provides an overview of pathway analysis results. We include the cradle-to-gate emissions for each pathway, the quantity of atmospheric carbon sequestered (reported in CO2e), and both the initial and 100-year disposition of sequestered CO2, as well as the net CO2 drawdown benefits (negative emissions). Life cycle sequestration durability and net CO2 are reported on both a per tC in feedstock and per-unit-product basis. Non-BECCS pathways achieve 34–64% of the initial drawdown magnitude relative to BECCS and retain 55–67% of the initial drawdown over 100 years (central estimate).
Table 3 Comparison of product pathways
|
Product type |
Sequestration |
Initial sequestration (kgCO2e per t per C)a |
100 year sequestration (kgCO2e per tC)a |
Initial drawdown (kgCO2e per tC) |
100 year drawdown (kgCO2e per tC) |
Initial drawdown (kgCO2e per unit product) |
100 year drawdown (kgCO2e per unit product) |
Product unit |
These quantities reflect the atmospheric carbon sequestered converted into equivalent quantities of atmospheric CO2
The 100-year sequestration values reflect the amount of atmospheric carbon remaining sequestered in CO2e while the 100-year drawdown quantities take into account carbon that has been released as methane. Therefore, the change in drawdown benefit may be larger than the change in carbon sequestered.
|
IGCC w/CCS |
Electricity |
Geologic reservoir |
3113 |
3109 |
−2811 |
−2807 |
−900 |
−899 |
MWh |
Polyethylene w/CCS |
Durable polymer |
Geologic reservoir |
1053 |
∼1053 |
−1197 |
−807b |
−3410 |
−2299b |
t |
Polyethylene/landfill |
1106 |
822b |
Biochar |
Soil amendment |
Agricultural soils |
1296 |
899 |
−963 |
−564 |
−2426 |
−1421 |
t |
OSB |
Durable wood product |
OSB/landfill |
2541 |
1819b |
−1806 |
−987b |
−700 |
−383b |
1000 ft2 of 3/8′′ panel |
The IGCC plant with CCS attains the most drawdown potential per ton of feedstock, with 91% of the initial drawdown benefit expected to persist over millennia. However, the performance of the other pathways is notable. The role of biopower may be limited by the increasing role of other low-carbon energy options. But there are many other sectors of the economy where the carbon removal potential of biomass feedstock can play a significant role. By combining geological sequestration with carbon storage in long-lived products, the PE pathway achieves 29% of the drawdown benefit of the IGCC plant at 100 years and maintains about 46% of the initial drawdown benefit at 10
000 years. The biochar pathway achieves 20% of the IGCC drawdown benefit at 100 years but yields net positive emissions over 10
000 years. The OSB pathway achieves 35% of the drawdown benefit of the IGCC plant at 100 years, with 11% of the initial drawdown benefit persisting over 10
000 years.
Discussion
Our analysis demonstrates a range of opportunities for the bioeconomy to contribute to carbon drawdown. BECCS likely remains a key component of drawdown strategies and serves as a useful baseline for comparing alternatives. Advanced biomass gasification pathways can facilitate access to higher-purity streams of CO2, minimizing the plant efficiency impacts of CO2 separation and capture. Geologic sequestration remains the benchmark given the greater security of long-term sequestration. However, other biomass utilization alternatives may present unique advantages. For instance, alternative markets may present fewer obstacles to scale or offer synergies with existing operations, production externalities (jobs, environmental impacts) may be more regionally beneficial, or processes may be able to utilize a broader range of feedstocks. We discuss our key findings, research needs, and the implications for existing climate mitigation policy.
All of the non-BECCS drawdown pathways analyzed store carbon at climate-relevant timescales. Fermentation pathways are promising because of the potential to produce carbon-negative polymers in addition to low-carbon fuels all while capturing high-purity streams of CO2 for geologic storage. Wood construction materials are a potentially carbon negative alternative to emissions intensive concrete and steel. Markets for polymers and wood products are already mature and do not face the same challenges to scale as more speculative pathways. Biochar can contribute to drawdown efforts while addressing challenges in forest management and improving agricultural yields and soil health.
Furthermore, our analysis highlights the importance of waste management in a comprehensive carbon mitigation system. The drawdown potential of durable goods is blunted by the impact of landfill emissions, namely CH4. Policymakers should take seriously the role of landfills in engineered carbon sequestration. Increased utilization or oxidation of methane across the full life of waste management projects would greatly enhance the potential of bio-based durable goods as negative emissions pathways.182
Our analysis suggests linked mitigation priorities of emissions reduction and carbon drawdown. The performance of drawdown pathways can be enhanced by reducing the energy and emissions intensity of supply chains and conversion, maximizing carbon stored in long-lived goods, and increasing the time which carbon is stored. Policymakers should be mindful that increasing the inflow of atmospheric carbon to engineered sinks relative to the outflow is a sufficient condition to increase the net stock of sequestered carbon. Moreover, magnitude and permanence of drawdown pathways are key policy considerations. Climate change is an intergenerational challenge and analyses should consider the fate of carbon beyond the conventional 100-year horizon.
Our analysis highlights the utility of flow-based accounting in life cycle models. Policymakers need consistent metrics to compare the magnitude, permanence, and temporal evolution of carbon drawdown pathways. Life cycle assessments and models (e.g. GREET) often adopt a “net zero” approach when dealing with biogenic carbon.183 This practice assumes biogenic emissions are accounted for at the point of harvest and is consistent with the stock change approach used in national GHG accounting.184 However, this approach is not suited to track biogenic carbon stored in durable goods, landfills, and soil amendments at the product system level.185 To consistently value carbon stored by biomass products, the magnitude of sequestration must be temporally resolved. Flow-based accounting facilitates that quantification, and the field of dynamic life cycle assessment has developed methods which allow comparison of time-dependent impacts for temporary sequestration.185
Given estimates of magnitude and permanence of carbon drawdown, metrics could be developed to compare the relative value of sequestration in biobased goods. Existing policies such as California's Low Carbon Fuel Standard (LCFS), Section 45Q of the US Tax Code and the proposed Section 45T incentivize geologic sequestration (LCFS and 45Q) and utilization (45Q) of industrial emissions and land management-based sequestration (45T). The policies could offer a framework for policy support of drawdown in biomass-based goods. Moreover, consistent biogenic carbon accounting could support performance-based mechanisms similar to the LCFS to reduce the carbon intensity of other high-volume markets (e.g. polymers, construction materials). For example, biorefineries, which often produce multiple products, could play a larger role in mitigation efforts by producing carbon negative durable goods in addition to the fuels they supply to existing low-carbon fuels markets.
Conclusion
This article provides a qualitative overview of prominent BiCRS technologies from which a set of the most promising technologies are assessed quantitively through life cycle assessment. There are numerous opportunities to incorporate carbon removal and management within the bioeconomy, but the majority of the near-term carbon removal potential exists in four bioproducts: bioenergy, bioplastics, biochar, and wood products.
We analyze the life cycle greenhouse gas emissions and disposition of sequestered carbon over 10
000 years for four bioproducts representative of each broader category: an advanced BECCS pathway, biopolyethylene, oriented strand board, and biochar soil amendment. We find that the BECCS pathway has the greatest magnitude and durability of CO2 storage over all time horizons. However, non-BECCS pathways achieve 34–64% of the initial drawdown magnitude relative to BECCS and retain 55–67% of the initial drawdown over 100 years (central estimate).
We identify three engineering strategies for enhancing carbon drawdown: reducing biomass supply chain emissions, maximizing carbon stored in long-lived products, and extending the term of carbon storage.
Finally, we highlight the need to characterize both the magnitude and permanence of carbon drawdown as a means for policymakers and technology developers to deploy limited biomass resources to maximize mitigation benefits.
A research agenda should begin to think beyond BECCS and take a holistic view of the potential role of biomass in carbon drawdown. Within the broader bioeconomy, carbon drawdown is an opportunity to create economic value, support working lands, and achieve climate benefits with an innovative systems approach to carbon management through biomass.
Conflicts of interest
There are no conflicts to declare.
Author contributions
W.J.S. conceived the literature review. W.J.S. and E.W. conducted the literature review. W.J.S. wrote the literature review with input from J.D. and D.L.S. D.L.S. and A.J.S. conceived the analysis in part 2. J.D. carried out data collection, modeling, and analysis is part 2, with assistance from H.G. J.D. wrote part 2 of the paper and the ESI with input from all co-authors.
Acknowledgements
This material is based upon work supported by the National Science Foundation Graduate Research Fellowship under Grant No. DGE 1752814. The authors would also like to thank Jeannette Lynn Yusko for work on the design of Fig. 1. This work was performed under the auspices of the US Department of Energy by Lawrence Livermore National Laboratory under contract DE-AC52-07NA27344. This document was prepared as an account of work sponsored by an agency of the US government. Neither the US government nor Lawrence Livermore National Security, LLC, nor any of their employees makes any warranty, expressed or implied, or assumes any legal liability or responsibility for the accuracy, completeness, or usefulness of any information, apparatus, product, or process disclosed, or represents that its use would not infringe privately owned rights. Reference herein to any specific commercial product, process, or service by trade name, trademark, manufacturer, or otherwise does not necessarily constitute or imply its endorsement, recommendation, or favoring by the US government or Lawrence Livermore National Security, LLC. The views and opinions of authors expressed herein do not necessarily state or reflect those of the US government or Lawrence Livermore National Security, LLC, and shall not be used for advertising or product endorsement purposes. Document release number: LLNL-TR-836682. This work was supported, in part, by the US Department of Energy funded project (award number DE-EE0009413), Integrating Carbon Capture, Utilization, & Sequestration into Chemical Pulp Mills.
References
-
European Commission, What is the Bioeconomy – Research – European Commission. 2021 [cited 2022 May 29]. Available from: https://ec.europa.eu/research/bioeconomy/policy/bioeconomy_en.htm.
- A. Aguilar, R. Wohlgemuth and T. Twardowski, Perspectives on bioeconomy, N Biotechnol., 2018, 40(Pt A), 181–184 CrossRef CAS PubMed.
- J. Jones, B. Verma, R. Mohtar and M. Matlock, Transforming food and agriculture to circular systems: a perspective for 2050, Resource Magazine, 2021, 28(2), 7 Search PubMed.
-
P. Forster, T. Storelvmo, K. Armour, W. Collins, J. L. Dufresne and D. Frame, et al., The Earth's Energy Budget, Climate Feedbacks, and Climate Sensitivity, in Climate Change 2021: The Physical Science Basis Contribution of Working Group I to the Sixth Assessment Report of the Intergovernmental Panel on Climate Change, ed. V. Masson-Delmotte, P. Zhai, A. Pirani, S. L. Connors, C. Péan and S. Berger, et al., Cambridge University Press, Cambridge, United Kingdom and New York, NY, USA, 2021. pp. 923–1054 Search PubMed.
-
U.S. Department of Energy, 2016 billion-ton report: Advancing domestic resources for a thriving bioeconomy, Volume 1: Economic Availability of Feedstocks. 2016.
- H. K. Jeswani, A. Chilvers and A. Azapagic, Environmental sustainability of biofuels: a review, Proc. R. Soc. A, 2020, 476(2243), 21 CrossRef PubMed.
-
J. Rogelj, D. Shindell, K. Jiang, S. Fifita, P. Forster and V. Ginzburg, et al., Mitigation pathways compatible with 1.5 C in the context of sustainable development, in Global Warming of 15 °C An IPCC Special Report on the impacts of global warming of 15 °C above pre-industrial levels and related global greenhouse gas emission pathways, in the context of strengthening the global response to the threat of climate change, ed. V. Masson-Delmotte, P. Zhai, H. O. Pörtner, D. Roberts, J. Skea and P. R. Shukla, et al., 2018. pp. 93–174 Search PubMed.
- D. Y. C. Leung, G. Caramanna and M. M. Maroto-Valer, An overview of current status of carbon dioxide capture and storage technologies, Renewable Sustainable Energy Rev., 2014, 39, 426–443 CrossRef CAS.
-
D. Sandalow, R. Aines, J. Friedmann, C. McCormick and D. L. Sanchez Biomass Carbon Removal and Storage (BiRCS) Roadmap. 2021 Jan 1.
-
W. J. Sagues and A. Woodley, Building a Biotechnology Innovation Ecosystem to Mitigate Climate Change. 2022 Search PubMed.
- M. He, Z. Xu, D. Hou, B. Gao, X. Cao and Y. S. Ok,
et al., Waste-derived biochar for water pollution control and sustainable development, Nat. Rev. Earth Environ., 2022, 3(7), 444–460 CrossRef CAS.
-
U.S. Code
, Credit for carbon oxide sequestration.
-
California Low Carbon Fuel Standard
, California Code of Regulations 2009.
- M. Honegger and D. Reiner, The political economy of negative emissions technologies: consequences for international policy design, Clim. Policy, 2018, 18(3), 306–321 CrossRef.
- S. Fuss, W. F. Lamb, M. W. Callaghan, J. Hilaire, F. Creutzig and T. Amann,
et al., Negative emissions—Part 2: Costs, potentials and side effects, Environ. Res. Lett., 2018, 13(6), 063002 CrossRef.
- J. C. Minx, W. F. Lamb, M. W. Callaghan, S. Fuss, J. Hilaire and F. Creutzig,
et al., Negative emissions—Part 1: Research landscape and synthesis, Environ. Res. Lett., 2018, 13(6), 063001 CrossRef.
-
S. E. Tanzer and A. Ramírez, When are negative emissions negative emissions?, in Vol. 12, Energy and Environmental Science, 2019, pp. 1210–1218 Search PubMed.
- A. Al-Mamoori, A. Krishnamurthy, A. A. Rownaghi and F. Rezaei, Carbon Capture and Utilization Update, Energy Technol., 2017, 5(6), 834–849 CrossRef.
- R. M. Cuéllar-Franca and A. Azapagic, Carbon capture, storage and utilisation technologies: A critical analysis and comparison of their life cycle environmental impacts, J. CO2 Util., 2015, 9, 82–102 CrossRef.
- T. Terlouw, C. Bauer, L. Rosa and M. Mazzotti, Life cycle assessment of carbon dioxide removal technologies: a critical review, Energy Environ. Sci., 2021, 14(4), 1701–1721 RSC.
- F. Creutzig, C. Breyer, J. Hilaire, J. Minx, G. P. Peters and R. Socolow, The mutual dependence of negative emission technologies and energy systems, Energy Environ. Sci., 2019, 12(6), 1805–1817 RSC.
- A. Babin, C. Vaneeckhaute and M. C. Iliuta, Potential and challenges of bioenergy with carbon capture and storage as a carbon-negative energy source: A review, Biomass Bioenergy, 2021, 146, 1–21 CrossRef.
- V. Stavrakas, N. A. Spyridaki and A. Flamos, Striving towards the Deployment of Bio-Energy with Carbon Capture and Storage (BECCS): A Review of Research Priorities and Assessment Needs, Sustainability, 2018, 10(7), 2206 CrossRef CAS.
- Y. Cao, M. He, S. Dutta, G. Luo, S. Zhang and D. C. W. Tsang, Hydrothermal carbonization and liquefaction for sustainable production of hydrochar and aromatics, Renewable Sustainable Energy Rev., 2021, 152, 111722 CrossRef CAS.
-
L. J. Snowden-Swan, S. Li, Y. Jiang, M. R. Thorson, A. J. Schmidt, T. E. Seiple, et al., Wet Waste Hydrothermal Liquefaction and Biocrude Upgrading to Hydrocarbon Fuels: 2021 State of Technology.
- R. C. Brown, The Role of Pyrolysis and Gasification in a Carbon Negative Economy, Processes, 2021, 9(5), 882 CrossRef CAS.
- V. S. Sikarwar, M. Zhao, P. Clough, J. Yao, X. Zhong and M. Z. Memon,
et al., An overview of advances in biomass gasification, Energy Environ. Sci., 2016, 9(10), 2939–2977 RSC.
-
R. M. Swanson, J. A. Satrio, R. C. Brown, A. Platon and D. D. Hsu, Techno-Economic Analysis of Biofuels Production Based on Gasification, 2010.
- D. L. Sanchez and D. S. Callaway, Optimal scale of carbon-negative energy facilities, Appl. Energy, 2016, 170, 437–444 CrossRef.
-
A. Dutta, K. Iisa, C. Mukarakate, M. Griffin, E. C. D. Tan, J. Schaidle, et al., Ex Situ Catalytic Fast Pyrolysis of Lignocellulosic Biomass to Hydrocarbon Fuels: 2018 State of Technology and Future Research., 2018.
- A. Bhave, R. H. S. Taylor, P. Fennell, W. R. Livingston, N. Shah and N. M. Dowell,
et al., Screening and techno-economic assessment of biomass-based power generation with CCS technologies to meet 2050 CO2 targets, Appl. Energy, 2017, 190, 481–489 CrossRef CAS.
- H. P. Schmidt, A. Anca-Couce, N. Hagemann, C. Werner, D. Gerten and W. Lucht,
et al., Pyrogenic carbon capture and storage, GCB Bioenergy, 2019, 11(4), 573–591 CrossRef CAS.
- J. Hetland, P. Yowargana, S. Leduc and F. Kraxner, Carbon-negative emissions: Systemic impacts of biomass conversion. A case study on CO2 capture and storage options, Int. J. Greenhouse Gas Control, 2016, 49, 330–342 CrossRef CAS.
- M. Langholtz, I. Busch, A. Kasturi, M. R. Hilliard, J. McFarlane and C. Tsouris,
et al., The Economic Accessibility of CO2 Sequestration through Bioenergy with Carbon Capture and Storage (BECCS) in the US, Land, 2020, 9(9), 299 CrossRef.
- D. L. Sanchez and D. M. Kammen, A commercialization strategy for carbon-negative energy, Nat. Energy, 2016, 1(1), 1–4 Search PubMed.
- J. Full, S. Merseburg, R. Miehe and A. Sauer, A New Perspective for Climate Change Mitigation—Introducing Carbon-Negative Hydrogen Production from Biomass with Carbon Capture and Storage (HyBECCS), Sustainability, 2021, 13(7), 4026 CrossRef CAS.
-
S. E. Baker, G. Peridas, J. K. Stolaroff, H. M. Goldstein, S. H. Pang and F. R. Lucci, et al., Getting to Neutral: Options for Negative Carbon Emissions in California, 2019 Search PubMed.
-
Energy Transitions Commission, Making the Hydrogen Economy Possible : accelerating clean hydrogen in an electrified economy. Making Mission Possible Series. 2021 [cited 2022 Sep 27]. Available from: https://www.energy-transitions.org/publications/making-clean-hydrogen-possible/.
- C. Arnaiz del Pozo, S. Cloete and Á. Jiménez Álvaro, Carbon-negative hydrogen: Exploring the techno-economic potential of biomass co-gasification with CO2 capture, Energy Convers. Manage., 2021, 247, 114712 CrossRef CAS.
- M. Fajardy and N. Mac Dowell, Can BECCS deliver sustainable and resource efficient negative emissions?, Energy Environ. Sci., 2017, 10(6), 1389–1426 RSC.
- M. He, Z. Xu, Y. Sun, P. S. Chan, I. Lui and D. C. W. Tsang, Critical impacts of pyrolysis conditions and activation methods on application-oriented production of wood waste-derived biochar, Bioresour. Technol., 2021, 341, 125811 CrossRef CAS PubMed.
- W. J. Sagues, S. Park, H. Jameel and D. L. Sanchez, Enhanced carbon dioxide removal from coupled direct air capture–bioenergy systems, Sustainable Energy Fuels, 2019, 3(11), 3135–3146 RSC.
- W. J. Sagues, H. Jameel, D. L. Sanchez and S. Park, Prospects for bioenergy with carbon capture & storage (BECCS) in the United States pulp and paper industry, Energy Environ. Sci., 2020, 13(8), 2243–2261 RSC.
-
Encyclopedia Britannica. Arrhenius equation | Definition & Facts | Britannica. Encyclopdedia Britannica. 2022 [cited 2022 Sep 29]. Available from: https://www.britannica.com/science/Arrhenius-equation.
- D. Kardaś, P. Hercel, I. Wardach-Święcicka and S. Polesek-Karczewska, On the kinetic rate of biomass particle decomposition - Experimental and numerical analysis, Energy, 2021, 219, 119575 CrossRef.
- D. L. Sanchez, N. Johnson, S. T. McCoy, P. A. Turner and K. J. Mach, Near-term deployment of carbon capture and sequestration from biorefineries in the United States, Proc. Natl. Acad. Sci. U. S. A., 2018, 115(19), 4875–4880 CrossRef CAS PubMed.
-
U.S. EPA, LMOP Landfill and Project Database | US EPA. [cited 2022 May 13]. Available from: https://www.epa.gov/lmop/lmop-landfill-and-project-database#access.
-
EPA, Livestock Anaerobic Digester Database. AgSTAR Livestock Anaerobic Digester Database. 2016 [cited 2022 May 13]. p. 3–4. Available from: https://www.epa.gov/agstar/livestock-anaerobic-digester-database.
-
JBEI, BioSiting Tool: Select Map. [cited 2022 May 13]. Available from: https://biositing.jbei.org/.
- J. R. Moreira, V. Romeiro, S. Fuss, F. Kraxner and S. A. Pacca, BECCS potential in Brazil: Achieving negative emissions in ethanol and electricity production based on sugar cane bagasse and other residues, Appl. Energy, 2016, 179, 55–63 CrossRef CAS.
- P. C. Psarras, S. Comello, P. Bains, P. Charoensawadpong, S. Reichelstein and J. Wilcox, Carbon Capture and Utilization in the Industrial Sector, Environ. Sci. Technol., 2017, 51(19), 11440–11449 CrossRef CAS PubMed.
- Y. Xu, L. Isom and M. A. Hanna, Adding value to carbon dioxide from ethanol fermentations, Bioresour. Technol., 2010, 101(10), 3311–3319 CrossRef CAS PubMed.
- P. M. Berger, L. Yoksoulian, J. T. Freiburg, S. K. Butler and W. R. Roy, Carbon sequestration at the Illinois Basin-Decatur Project: experimental results and geochemical simulations of storage, Environ. Earth Sci., 2019, 78(22), 1–10 CAS.
- J. N. Rosenberg, A. Mathias, K. Korth, M. J. Betenbaugh and G. A. Oyler, Microalgal biomass production and carbon dioxide sequestration from an integrated ethanol biorefinery in Iowa: A technical appraisal and economic feasibility evaluation, Biomass Bioenergy, 2011, 35(9), 3865–3876 CrossRef CAS.
- J. L. Field, T. L. Richard, E. A. H. Smithwick, H. Cai, M. S. Laser and D. S. LeBauer,
et al., Robust paths to net greenhouse gas mitigation and negative emissions via advanced biofuels, Proc. Natl. Acad. Sci. U. S. A., 2020, 117(36), 21968–21977 CrossRef CAS PubMed.
- S. Kim, X. Zhang, A. D. Reddy, B. E. Dale, K. D. Thelen and C. D. Jones,
et al., Carbon-Negative Biofuel Production, Environ. Sci. Technol., 2020, 54(17), 10797–10807 CrossRef CAS PubMed.
- H. Li, Y. Tan, M. Ditaranto, J. Yan and Z. Yu, Capturing CO2 from Biogas Plants, Energy Procedia, 2017, 114, 6030–6035 CrossRef CAS.
- K. Rajendran and G. S. Murthy, Techno-economic and life cycle assessments of anaerobic digestion – A review, Biocatal. Agric. Biotechnol., 2019, 20, 101207 CrossRef.
- W. J. Nock, M. Walker, R. Kapoor and S. Heaven, Modeling the water scrubbing process and energy requirements for CO 2 capture to upgrade biogas to biomethane, Ind. Eng. Chem. Res., 2014, 53(32), 12783–12792 CrossRef CAS.
- G. Shah, E. Ahmad, K. K. Pant and V. K. Vijay, Comprehending the contemporary state of art in biogas enrichment and CO2 capture technologies via swing adsorption, Int. J. Hydrogen Energy, 2021, 46(9), 6588–6612 CrossRef CAS.
- M. S. Ivan, K. M. Vítězová, M. Struk, I. Kushkevych and V. Ám, Biogas upgrading methods: recent advancements and emerging technologies Pressure swing adsorption PSB Purple sulphur bacteria VSA Vacuum swing adsorption, Rev. Environ. Sci. Biotechnol., 2020, 19, 651–671 CrossRef.
- W. J. Sagues, C. A. Assis, P. Hah, D. L. Sanchez, Z. Johnson and M. Acharya,
et al., Decarbonizing agriculture through the conversion of animal manure to dietary protein and ammonia fertilizer, Bioresour. Technol., 2020, 297, 122493 CrossRef CAS PubMed.
-
S. Wang, T. Zhang, M. Bao, H. Su and P. XuMicrobial Production of Hydrogen by Mixed Culture Technologies: A Review. https://www.biotechnology-journal.com. 2019.
- P. A. Turner, K. J. Mach, D. B. Lobell, S. M. Benson, E. Baik and D. L. Sanchez,
et al., The global overlap of bioenergy and carbon sequestration potential, Clim. Change, 2018, 148(1–2), 1–10 CrossRef CAS.
- E. Baik, D. L. Sanchez, P. A. Turner, K. J. Mach, C. B. Field and S. M. Benson, Geospatial analysis of near-term potential for carbon-negative bioenergy in the United States, Proc. Natl. Acad. Sci. U. S. A., 2018, 115(13), 3290–3295 CrossRef CAS PubMed.
- R. Meys, A. Kätelhön, M. Bachmann, B. Winter, C. Zibunas and S. Suh,
et al., Achieving net-zero greenhouse gas emission plastics by a circular carbon economy, Science, 2021, 374(6563), 71–76 CrossRef CAS PubMed.
- C. Smeaton, Augmentation of global marine sedimentary carbon storage in the age of plastic, Limnol. Oceanogr. Lett., 2021, 6(3), 113–118 CrossRef CAS.
-
United Nations Environment Programme, Visual Feature | Beat Plastic Pollution. UNEP. 2022 [cited 2022 May 13]. Available from: https://www.unep.org/interactives/beat-plastic-pollution/.
-
L. Parker, A Whopping 91 Percent of Plastic Isn't Recycled | National Geographic Society. National Geographic Societyo. 2019 [cited 2022 May 13]. Available from: https://www.nationalgeographic.org/article/whopping-91-percent-plastic-isnt-recycled/.
- J. P. Dees, M. Ateia and D. L. Sanchez, Microplastics and Their Degradation Products in Surface Waters: A Missing Piece of the Global Carbon Cycle Puzzle, ACS ES&T Water, 2021, 1(2), 214–216 Search PubMed.
-
Department of Ecology State of Washington. Focus on Bioplastics: “Biobased”, “Biodegradable”, and “Compostable”. 2014.
-
European Bioplastics, Bioplastics market data. european-bioplastics.org. 2021 [cited 2022 May 13]. Available from: https://www.european-bioplastics.org/market/#iLightbox[gallery_image_1]/-1.
- R. Mülhaupt, Green polymer chemistry and bio-based plastics: Dreams and reality, Macromol. Chem. Phys., 2013, 214(2), 159–174 CrossRef.
- T. A. Hottle, M. M. Bilec and A. E. Landis, Biopolymer production and end of life comparisons using life cycle assessment, Resour., Conserv. Recycl., 2017, 122, 295–306 CrossRef.
- F. Gironi and V. Piemonte, Bioplastics Disposal: How To Manage It, WIT Trans. Ecol. Environ., 2010, 140, 261–271 Search PubMed.
- G. Vinci, R. Ruggieri, A. Billi, C. Pagnozzi, M. V. Di Loreto and M. Ruggeri, Sustainable management of organic waste and recycling for bioplastics: A lca approach for the italian case study, Sustainability, 2021, 13(11), 10–17 CrossRef.
- E. T. H. Vink, K. R. Rábago, D. A. Glassner and P. R. Gruber, Applications of life cycle assessment to NatureWorks™ polylactide (PLA) production, Polym. Degrad. Stab., 2003, 80(3), 403–419 CrossRef CAS.
- R. Geyer, J. R. Jambeck and K. L. Law, Production, use, and fate of all plastics ever made, Sci. Adv., 2017, 3(7), 4 Search PubMed.
- T. Leejarkpai, T. Mungcharoen and U. Suwanmanee, Comparative assessment of global warming impact and eco-efficiency of PS (polystyrene), PET (polyethylene terephthalate) and PLA (polylactic acid) boxes, J. Cleaner Prod., 2016, 125, 95–107 CrossRef CAS.
- A. Morschbacker, Bio-ethanol based ethylene, Polym. Rev., 2009, 49(2), 79–84 CrossRef CAS.
- N. Fackler, B. D. Heijstra, B. J. Rasor, H. Brown, J. Martin and Z. Ni,
et al., Stepping on the Gas to a Circular Economy: Accelerating Development of Carbon-Negative Chemical Production from Gas Fermentation, Annu. Rev. Chem. Biomol. Eng., 2021, 12, 439–470 CrossRef PubMed.
- Y. Ni, G. M. Richter, O. N. Mwabonje, A. Qi, M. K. Patel and J. Woods, Novel integrated agricultural land management approach provides sustainable biomass feedstocks for bioplastics and supports the UK's ‘net-zero’ target, Environ. Res. Lett., 2020, 16(1), 014023 CrossRef.
- D. Tonini, D. Schrijvers, S. Nessi, P. Garcia-Gutierrez and J. Giuntoli, Carbon footprint of plastic from biomass and recycled feedstock: methodological insights, Int. J. Life Cycle Assess., 2021, 26(2), 221–237 CrossRef.
- Y. Zhu, C. Romain and C. K. Williams, Sustainable polymers from renewable resources, Nature, 2016, 540(7633), 354–362 CrossRef CAS PubMed.
- D. Zhang, E. A. del Rio-Chanona, J. L. Wagner and N. Shah, Life cycle assessments of bio-based sustainable polylimonene carbonate production processes, Sustainable Prod. Consum., 2018, 14, 152–160 CrossRef.
- A. Rehman, F. Saleem, F. Javed, A. Ikhlaq, S. W. Ahmad and A. Harvey, Recent advances in the synthesis of cyclic carbonates via CO2 cycloaddition to epoxides, J. Environ. Chem. Eng., 2021, 9(2), 105113 CrossRef CAS.
- O. Hauenstein, M. Reiter, S. Agarwal, B. Rieger and A. Greiner, Bio-based polycarbonate from limonene oxide and CO2 with high molecular weight, excellent thermal resistance, hardness and transparency, Green Chem., 2016, 18(3), 760–770 RSC.
- O. Hauenstein, S. Agarwal and A. Greiner, Bio-based polycarbonate as synthetic toolbox, Nat. Commun., 2016, 7(1), 1–7 Search PubMed.
- Z. Li, J. Yang and X. J. Loh, Polyhydroxyalkanoates: opening doors for a sustainable future, NPG Asia Mater., 2016, 8(4), e265–e265 CrossRef CAS.
- A. Z. Naser, I. Deiab and B. M. Darras, Poly(lactic acid) (PLA) and polyhydroxyalkanoates (PHAs), green alternatives to petroleum-based plastics: a review, RSC Adv., 2021, 11(28), 17151–17196 RSC.
- J. Maroušek, M. Vochozka, J. Plachý and J. Žák, Glory and misery of biochar, Clean Technol. Environ. Policy, 2016, 19(2), 311–317 CrossRef.
- B. Wang, B. Gao and J. Fang, Recent advances in engineered biochar productions and applications, Crit. Rev. Environ. Sci. Technol., 2017, 47(22), 2158–2207 CrossRef CAS.
- X. Zhu, D. C. W. Tsang, L. Wang, Z. Su, D. Hou and L. Li,
et al., Machine learning exploration of the
critical factors for CO2 adsorption capacity on porous carbon materials at different pressures, J. Cleaner Prod., 2020, 273, 122915 CrossRef CAS.
- A. A. Abd, M. R. Othman and J. Kim, A review on application of activated carbons for carbon dioxide capture: present performance, preparation, and surface modification for further improvement, Environ. Sci. Pollut. Res., 2021, 28(32), 43329–43364 CrossRef CAS PubMed.
- S. Jung, Y. K. Park and E. E. Kwon, Strategic use of biochar for CO2 capture and sequestration, J. CO2 Util., 2019, 32, 128–139 CrossRef CAS.
- Q. Yin, B. Zhang, R. Wang and Z. Zhao, Biochar as an adsorbent for inorganic nitrogen and phosphorus removal from water: a review, Environ. Sci. Pollut. Res. Int., 2017, 24(34), 26297–26309 CrossRef CAS PubMed.
- W. Zhao, H. Yang, S. He, Q. Zhao and L. Wei, A review of biochar in anaerobic digestion to improve biogas production: Performances, mechanisms and economic assessments, Bioresour. Technol., 2021, 341, 125797 CrossRef CAS PubMed.
-
M. Burton and E. Van Der WaltElectric-Car Revolution Shakes Up the Biggest Metals Markets. Bloomberg. 2017 [cited 2022 May 13]. Available from: https://www.bloomberg.com/news/articles/2017-08-02/electric-car-revolution-is-shaking-up-the-biggest-metals-markets.
-
A. Mayyas, Are there enough materials to cover li-ion batteries?. Joint Institute for Strategic Energy Analysis [NREL]. 2018 [cited 2022 May 13]. Available from: https://www.jisea.org/20180815.html.
- W. J. Sagues, J. Yang, N. Monroe, S. D. Han, T. Vinzant and M. Yung,
et al., A simple method for producing bio-based anode materials for lithium-ion batteries, Green Chem., 2020, 22(20), 7093–7108 RSC.
- L. Zhao, X. Zhao, L. T. Burke, J. C. Bennett, R. A. Dunlap and M. N. Obrovac, Voronoi-Tessellated Graphite Produced by Low-Temperature Catalytic Graphitization from Renewable Resources, ChemSusChem, 2017, 10(17), 3409–3418 CrossRef CAS PubMed.
- J. Yang and S. Zuo, Facile synthesis of graphitic mesoporous carbon materials from sucrose, Diamond Relat. Mater., 2019, 95, 1–4 CrossRef CAS.
- M. N. Obrovac, X. Zhao, L. T. Burke and R. A. Dunlap, Reversible lithium insertion in catalytically graphitized sugar carbon, Electrochem. Commun., 2015, 60, 221–224 CrossRef CAS.
- S. Xia, N. Cai, W. Lu, H. Zhou, H. Xiao and X. Chen,
et al., Reaction kinetics, mechanism, and product analysis of the iron catalytic graphitization of cellulose, J. Cleaner Prod., 2021, 329, 129735 CrossRef CAS.
- E. Thompson, A. E. Danks, L. Bourgeois and Z. Schnepp, Iron-catalyzed graphitization of biomass, Green Chem., 2014, 17(1), 551–556 RSC.
- N. A. Banek, D. T. Abele, K. R. McKenzie and M. J. Wagner, Sustainable Conversion of Lignocellulose to High-Purity, Highly Crystalline Flake Potato Graphite, ACS Sustainable Chem. Eng., 2018, 6(10), 13199–13207 CrossRef CAS.
- H. G. Chae, B. A. Newcomb, P. V. Gulgunje, Y. Liu, K. K. Gupta and M. G. Kamath,
et al., High strength and high modulus carbon fibers, Carbon, 2015, 93, 81–87 CrossRef CAS.
- F. Souto, V. Calado and N. Pereira, Lignin-based carbon fiber: a current overview, Mater. Res. Express, 2018, 5(7), 072001 CrossRef.
- W. J. Sagues, A. Jain, D. Brown, S. Aggarwal, A. Suarez and M. Kollman,
et al., Are lignin-derived carbon fibers graphitic enough?, Green Chem., 2019, 21(16), 4253–4265 RSC.
- E. M. Karp, T. R. Eaton, V. SànchezNogué, V. Vorotnikov, M. J. Biddy and E. C. D. Tan,
et al., Renewable acrylonitrile production, Science, 2017, 358(6368), 1307–1310 CrossRef CAS PubMed.
- M. Al Aiti, D. Jehnichen, D. Fischer, H. Brünig and G. Heinrich, On the morphology and structure formation of carbon fibers from polymer precursor systems, Prog. Mater. Sci., 2018, 98, 477–551 CrossRef CAS.
- L. A. Biederman and W. Stanley Harpole, Biochar and its effects on plant productivity and nutrient cycling: a meta-analysis, GCB Bioenergy, 2013, 5(2), 202–214 CrossRef CAS.
- C. Zhang, G. Zeng, D. Huang, C. Lai, M. Chen and M. Cheng,
et al., Biochar for environmental management: Mitigating greenhouse gas emissions, contaminant treatment, and potential negative impacts, Chem. Eng. J., 2019, 373, 902–922 CrossRef CAS.
- J. Wang, Z. Xiong and Y. Kuzyakov, Biochar stability in soil: meta–analysis of decomposition and priming effects, GCB Bioenergy, 2016, 8(3), 512–523 CrossRef CAS.
- P. Brassard, S. Godbout and V. Raghavan, Soil biochar amendment as a climate change mitigation tool: Key parameters and mechanisms involved, J. Environ. Manage., 2016, 181, 484–497 CrossRef CAS PubMed.
- L. Leng and H. Huang, An overview of the effect of pyrolysis process parameters on biochar stability, Bioresour. Technol., 2018, 270, 627–642 CrossRef CAS PubMed.
- A. El-Naggar, A. H. El-Naggar, S. M. Shaheen, B. Sarkar, S. X. Chang and D. C. W. Tsang,
et al., Biochar composition-dependent impacts on soil nutrient release, carbon mineralization, and potential environmental risk: A review, J. Environ. Manage., 2019, 241, 458–467 CrossRef CAS PubMed.
- R. R. Tan, Data challenges in optimizing biochar-based carbon sequestration, Renewable Sustainable Energy Rev., 2019, 104, 174–177 CrossRef.
- X. Zhu, C. Labianca, M. He, Z. Luo, C. Wu and S. You,
et al., Life-cycle assessment of pyrolysis processes for sustainable production of biochar from agro-residues, Bioresour. Technol., 2022, 360, 127601 CrossRef CAS PubMed.
- J. Matuštík, T. Hnátková and V. Kočí, Life cycle assessment of biochar-to-soil systems: A review, J. Cleaner Prod., 2020, 259, 120998 CrossRef.
- K. E. Skog, Sequestration of carbon in harvested wood products for the United States, For. Prod. J., 2008, 58(6), 56–72 CAS.
- R. Bergman, M. Puettmann, A. Taylor and K. E. Skog, The Carbon Impacts of Wood Products, For. Prod. J., 2014, 64(7–8), 220–231 Search PubMed.
- R. Sathre and J. O'Connor, Meta-analysis of greenhouse gas displacement factors of wood product substitution, Environ. Sci. Policy, 2010, 13(2), 104–114 CrossRef CAS.
- P. Brunet-Navarro, H. Jochheim and B. Muys, Modelling carbon stocks and fluxes in the wood product sector: a comparative review, Global Change Biol., 2016, 22(7), 2555–2569 CrossRef PubMed.
- X. Wang, J. M. Padgett, J. S. Powell and M. A. Barlaz, Decomposition of forest products buried in landfills, Waste Manage., 2013, 2267–2276 CrossRef CAS PubMed.
- F. B. De la Cruz, J. P. Chanton and M. A. Barlaz, Measurement of carbon storage in landfills from the biogenic carbon content of excavated waste samples, Waste Manage., 2013, 33(10), 2001–2005 CrossRef CAS PubMed.
- L. Arroja, A. C. Dias and I. Capela, The Role of Eucalyptus Globulus Forest and Products in Carbon Sequestration, Clim. Change, 2006, 74(1), 123–140 CrossRef CAS.
- J. H. Arehart, J. Hart, F. Pomponi and B. D'Amico, Carbon sequestration and storage in the built environment, Sustainable Prod. Consum., 2021, 27, 1047–1063 CrossRef.
- M. A. Carle, S. D'Amours, R. Azouzi and M. Rönnqvist, A Strategic Forest Management Model for Optimizing Timber Yield and Carbon Sequestration, For. Sci., 2021, 67(2), 205–218 CrossRef.
- T. J. Fahey, P. B. Woodbury, J. J. Battles, C. L. Goodale, S. P. Hamburg and S. V. Ollinger,
et al., Forest carbon storage: ecology, management, and policy, Front. Ecol.
Environ., 2010, 8(5), 245–252 CrossRef.
- A. Favero, A. Daigneault and B. Sohngen, Forests: Carbon sequestration, biomass energy, or both?, Sci. Adv., 2020, 6(13), 1–13 Search PubMed.
- E. L. Kalies and L. L. Yocom Kent, Tamm Review: Are fuel treatments effective at achieving ecological and social objectives?, A systematic review, For. Ecol. Manage., 2016, 375, 84–95 CrossRef.
- S. L. Stephens, A. L. R. Westerling, M. D. Hurteau, M. Z. Peery, C. A. Schultz and S. Thompson, Fire and climate change: conserving seasonally dry forests is still possible, Front. Ecol. Environ., 2020, 18(6), 354–360 CrossRef.
- R. W. Malmsheimer, J. L. Bowyer, J. S. Fried, E. Gee, R. L. Izlar and R. A. Miner,
et al., Managing forests because carbon matters: Integrating energy, products, and land management policy, SAF, 2011, 109(7), S9–S12 Search PubMed.
- M. K. White, S. T. Gower and D. E. Ahl, Life cycle inventories of roundwood production in northern Wisconsin: Inputs into an industrial forest carbon budget, For. Ecol. Manage., 2005, 219(1), 13–28 CrossRef.
- P. Saud, J. Wang, B. D. Sharma and W. Liu, Carbon impacts of hardwood lumber processing in the northeastern United States, Can. J. For. Res., 2015, 45(12), 1699–1710 CrossRef CAS.
- W. E. Schlosser, J. H. Bassman, F. G. Wagner and P. R. Wandschneider, Increasing long-term storage of carbon sequestered in Russian softwood logs through enhanced lumber recovery, For. Prod. J., 2002, 52(9), 51–59 Search PubMed.
- C. A. Bolin and S. Smith, Life cycle assessment of ACQ-treated lumber with comparison to wood plastic composite decking, J. Cleaner Prod., 2011, 19(6–7), 620–629 CrossRef CAS.
- C. A. Bolin and S. T. Smith, Life cycle assessment of borate-treated lumber with comparison to galvanized steel framing, J. Cleaner Prod., 2011, 19(6–7), 630–639 CrossRef CAS.
-
D. L. Sanchez, T. Zimring, C. Mater and K. HarrellLiterature Review and Evaluation of Research Gaps to Support Wood Products Innovatation. 2020 Search PubMed.
- S. van Ewijk, J. A. Stegemann and P. Ekins, Limited climate benefits of global recycling of pulp and paper, Nat. Sustain., 2021, 4(2), 180–187 CrossRef.
- S. van Ewijk, J. A. Stegemann and P. Ekins, Limited climate benefits of global recycling of pulp and paper, Nat. Sustain., 2020, 4(2), 180–187 CrossRef.
- A. Haile, G. G. Gelebo, T. Tesfaye, W. Mengie, M. A. Mebrate and A. Abuhay,
et al., Pulp and paper mill wastes: utilizations and prospects for high value-added biomaterials, Bioresour Bioprocess, 2021, 8(1), 1–22 CrossRef.
- B. P. McGrail, C. J. Freeman, C. F. Brown, E. C. Sullivan, S. K. White and S. Reddy,
et al., Overcoming business model uncertainty in a carbon dioxide capture and sequestration project: Case study at the Boise White Paper Mill, Int. J. Greenhouse Gas Control, 2012, 9, 91–102 CrossRef.
-
C. Bataille, Low and zero emissions in the steel and cement industries, 2020 Search PubMed.
- S. E. Tanzer, K. Blok and A. Ramírez, Can bioenergy with carbon capture and storage result in carbon negative steel?, Int. J. Greenhouse Gas Control, 2020, 100, 103104 CrossRef CAS.
- A. T. Ubando, W. H. Chen, R. R. Tan and S. R. Naqvi, Optimal integration of a biomass-based polygeneration system in an iron production plant for negative carbon emissions, Int. J. Energy Res., 2020, 44(12), 9350–9366 CrossRef CAS.
- S. Gupta, H. W. Kua and S. D. Pang, Biochar-mortar composite: Manufacturing, evaluation of physical properties and economic viability, Constr. Build. Mater., 2018, 167, 874–889 CrossRef.
- B. A. Akinyemi and A. Adesina, Recent advancements in the use of biochar for cementitious applications: A review, J. Build. Eng., 2020, 32, 101705 CrossRef.
- D. Cuthbertson, U. Berardi, C. Briens and F. Berruti, Biochar from residual biomass as a concrete filler for improved thermal and acoustic properties, Biomass Bioenergy, 2019, 120, 77–83 CrossRef CAS.
- S. Praneeth, R. Guo, T. Wang, B. K. Dubey and A. K. Sarmah, Accelerated carbonation of biochar reinforced cement-fly ash composites: Enhancing and sequestering CO2 in building materials, Constr. Build. Mater., 2020, 244, 118363 CrossRef CAS.
- X. Yang and X. Y. Wang, Hydration-strength-durability-workability of biochar-cement binary blends, J. Build. Eng., 2021, 42, 103064 CrossRef.
- Z. Asadi Zeidabadi, S. Bakhtiari, H. Abbaslou and A. R. Ghanizadeh, Synthesis, characterization and evaluation of biochar from agricultural waste biomass for use in building materials, Constr. Build. Mater., 2018, 181, 301–308 CrossRef CAS.
-
Y. Zhang, M. He, L. Wang, J. Yan, B. Ma and X. Zhu, et al., Biochar as construction materials for achieving carbon neutrality. Vol. 4, Biochar. Springer, 2022 Search PubMed.
- L. Chen, Y. Zhang, L. Wang, S. Ruan, J. Chen and H. Li,
et al., Biochar-augmented carbon-negative concrete, Chem. Eng. J., 2022, 431, 133946 CrossRef CAS.
- L. Wang, L. Chen, D. C. W. Tsang, B. Guo, J. Yang and Z. Shen,
et al., Biochar as green additives in cement-based composites with carbon dioxide curing, J. Cleaner Prod., 2020, 258, 120678 CrossRef CAS.
- M. U. Hossain, L. Wang, I. K. M. Yu, D. C. W. Tsang and C. S. Poon, Environmental and technical feasibility study of upcycling wood waste into cement-bonded particleboard, Constr. Build. Mater., 2018, 173, 474–480 CrossRef.
- J. Alcalde, S. Flude, M. Wilkinson, G. Johnson, K. Edlmann and C. E. Bond,
et al., Estimating geological CO2 storage security to deliver on climate mitigation, Nat. Commun., 2018, 9(1), 1–9 CrossRef CAS PubMed.
-
U.S. Environmental, Protection Agency. Plastics: Material-Specific Data. Facts and Figures about Materials, Waste and Recycling. 2020 [cited 2020 Jul 10]. Available from: https://www.epa.gov/facts-and-figures-about-materials-waste-and-recycling/plastics-material-specific-data.
- C. P. Ward and C. M. Reddy, We need better data about the environmental persistence of plastic goods, Proc. Natl. Acad. Sci. U. S. A., 2020, 117(26), 14618–14621 CrossRef CAS PubMed.
- S. Ghatge, Y. Yang, J. H. Ahn and H. G. Hur, Biodegradation of polyethylene: a brief review, Appl. Biol. Chem., 2020, 63, 27 CrossRef CAS.
- A. A. Shah, F. Hasan, A. Hameed and S. Ahmed, Biological degradation of plastics: A comprehensive review, Biotechnol. Adv., 2008, 26(3), 246–265 CrossRef CAS PubMed.
- A. Chamas, H. Moon, J. Zheng, Y. Qiu, T. Tabassum and J. H. Jang,
et al., Degradation Rates of Plastics in the Environment, ACS Sustainable Chem. Eng., 2020, 8(9), 3494–3511 CrossRef CAS.
- W. C. Stewart and G. M. Nakamura, Documenting the full climate benefits of harvested wood products in northern california: Linking harvests to the us greenhouse gas inventory, For. Prod. J., 2012, 62(5), 340–353 Search PubMed.
- F. Santos, M. S. Torn and J. A. Bird, Biological degradation of pyrogenic organic matter in temperate forest soils, Soil Biol. Biochem., 2012, 51, 115–124 CrossRef CAS.
-
N. P. Gurwick, L. A. Moore, C. Kelly and P. Elias, A Systematic Review of Biochar Research, with a Focus on Its Stability in situ and Its Promise as a Climate Mitigation Strategy, ed. Q. Sun, PLoS One, 2013, vol. 8(9), p. e75932 Search PubMed.
- B. P. Singh, A. L. Cowie and R. J. Smernik, Biochar carbon stability in a clayey soil as a function of feedstock and pyrolysis temperature, Environ. Sci. Technol., 2012, 46(21), 11770–11778 CrossRef CAS PubMed.
- A. Enders, K. Hanley, T. Whitman, S. Joseph and J. Lehmann, Characterization of biochars to evaluate recalcitrance and agronomic performance, Bioresour. Technol., 2012, 114, 644–653 CrossRef CAS PubMed.
-
IPCC, Climate Change 2013: The Physical Science Basis. Contribution of Working Group I to the Fifth Assessment Report of the Intergovernmental Panel on Climate Change, ed. T. F. Stocker, D. Qin, G.-K. Plattner, M. Tignor, S. K. Allen, J. Boschung, A. Nauels, Y. Xia, V. Bex and P. M. Midgley, Cambridge University Press, Cambridge, United Kingdom and New York, NY, USA, 2013, pp 1535, https://www.ipcc.ch/report/ar5/wg1/.
- A. Levasseur, P. Lesage, M. Margni, L. Deschěnes and R. Samson, Considering time in LCA: Dynamic LCA and its application to global warming impact assessments, Environ. Sci. Technol., 2010, 44(8), 3169–3174 CrossRef CAS PubMed.
- M. A. Sanderson, P. R. Adler, A. A. Boateng, M. D. Casler and G. Sarath, Switchgrass as a biofuels feedstock in the USA, Can. J. Plant Sci., 2006, 86(5), 1315–1325 CrossRef.
- T. D. Montgomery, H. S. Han and A. R. Kizha, Modeling work plan logistics for centralized biomass recovery operations in mountainous terrain, Biomass Bioenergy, 2016, 85, 262–270 CrossRef.
- G. M. Pedroso, C. De Ben, R. B. Hutmacher, S. Orloff, D. Putnam and J. Six,
et al., Switchgrass is a promising, high-yielding crop for California biofuel, Calif. Agric., 2011, 65(3), 168–173 CrossRef.
- D. S. Newsome, The Water-Gas Shift Reaction, Catal. Rev., 1980, 21(2), 275–318 CrossRef CAS.
- M. Kanniche, R. Gros-Bonnivard, P. Jaud, J. Valle-Marcos, J. M. Amann and C. Bouallou, Pre-combustion, post-combustion and oxy-combustion in thermal power plant for CO2 capture, Appl. Therm. Eng., 2010, 30(1), 53–62 CrossRef CAS.
- C. Kunze and H. Spliethoff, Assessment of oxy-fuel, pre- and post-combustion-based carbon capture for future IGCC plants, Appl. Energy, 2012, 94, 109–116 CrossRef CAS.
-
M. Wang, A. Elgowainy, Z. Lu, P. T. Benavides, A. Burnham, H. Cai, et al., Greenhouse gases, Regulated Emissions, and Energy use in Transportation Model ® (2018 .Net). 2018.
- S. K. Thengane, K. Kung, R. York, S. Sokhansanj, C. J. Lim and D. L. Sanchez, Technoeconomic and emissions evaluation of mobile in-woods biochar production, Energy Convers. Manage., 2020, 223, 113305 CrossRef CAS.
- J. Jin, S. Chen and R. Wellwood, Oriented Strand Board: Opportunities and Potential Products in China, Bioresources, 2016, 11(4), 10585–10603 Search PubMed.
-
M. Puettmann, E. Oniel, E. Kline and L. Johnson Cradle to Gate Life Cycle Assessment of Oriented Strandboard Production from the Southeast, 2012.
- D. E. Kline, Gate-to-gate life-cycle inventory of oriented strandboard production, Wood Fiber Sci., 2005, 37, 74–84 CAS.
-
National Academies of Science Engineering and Medicine. Negative Emissions Technologies and Reliable Sequestration. Negative Emissions Technologies and Reliable Sequestration: A Research Agenda. Washington D.C.; 2019.
- T. D. Searchinger, S. P. Hamburg, J. Melilo, W. L. Chameides, P. Havlik and D. M. Kammen,
et al., Fixing a critical climate accounting error, Science, 2009, 326(5952), 527–528 CrossRef CAS PubMed.
-
J. Goodwin, M. Gillenwater, D. Romano and K. Radunsky, Chapter 1 Introduction To National Ghg Inventories, in Refinement to the 2006 IPCC Guidelines for National Greenhouse Gas Inventories, 2019, vol. 1, pp. 1–22 Search PubMed.
- A. Levasseur, P. Lesage, M. Margni and R. Samson, Biogenic Carbon and Temporary Storage Addressed with Dynamic Life Cycle Assessment, J. Ind. Ecol., 2013, 17(1), 117–128 CrossRef CAS.
|
This journal is © The Royal Society of Chemistry 2023 |