DOI:
10.1039/D3FO02460A
(Paper)
Food Funct., 2023,
14, 8987-8999
Bioactive compounds in pomace olive oil modulate the inflammatory response elicited by postprandial triglyceride-rich lipoproteins in BV-2 cells
Received
19th June 2023
, Accepted 30th August 2023
First published on 4th September 2023
Abstract
Modulation of microglial response could be a target to reduce neuroinflammation associated with Alzheimer's disease. In this study, we propose that lipophilic bioactive molecules present in pomace olive oil (POO), transported in triglyceride-rich lipoproteins (TRLs), are able to modulate microglial high-oleic sunflower oil (HOSO, points) or pomace olive oil (POO, stripes). In order to prove this hypothesis, a randomized crossover postprandial trial was performed in 18 healthy young women. POO was assayed in opposition to high-oleic sunflower oil (HOSO), a common dietary oil which shares with POO an almost identical fatty acid composition but lacks certain biomolecules with recognized antioxidant and anti-inflammatory activities. TRLs were isolated from blood at the baseline and 2 and 4 hours postprandially and used to treat BV-2 cells to assess their ability to modulate the microglial function. We found that the intake of POO leads to the constitution of postprandial TRLs that are able to modulate the inflammatory response in microglia compared to HOSO. TRL-derived POO reduced the release of pro-inflammatory cytokines (tumor necrosis factor-α, and interleukins 1β and 6) and nitric oxide and downregulated genes codifying for these cytokines and inducible nitric oxide synthase (iNOS) in BV-2 cells. Moreover, the ingestion of POO by healthy women slightly improved glycemic control and TRL clearance throughout the postprandial phase compared to HOSO. In conclusion, we demonstrated that consuming POO results in postprandial TRLs containing lipophilic bioactive compounds capable of regulating the inflammatory response prompted by microglial activation.
1. Introduction
At the moment, there is no cure for Alzheimer's disease (AD), one of the most disabling neurodegenerative diseases, even though its prevalence is constantly increasing.1 Among the pathological processes to which the etiology of the disease has been attributed, misfolding of proteins and formation of protein aggregates in the form of extracellular accumulation of amyloid beta (Aβ) and hyperphosphorylation of tau protein are the most known hallmarks of AD.2 In addition, mitochondrial dysfunction, oxidative stress, autophagy impairment, metal ion metabolism disorder, dysfunction of lipid metabolism, inflammation and neurogenesis impairment are also on the list.3–5 Accumulating evidence suggests that certain dietary lipids could lead to some of these processes, including dyslipidaemia, endothelial dysfunction, inflammation and oxidative stress.6
A significant part of plasma Aβ is associated with postprandial triglyceride-rich lipoproteins (TRLs), which also transport dietary fats.7 Besides, it has been shown that the type of dietary fat has an impact on the postprandial response, the secretion of TRL-Aβ8 and blood brain barrier (BBB) integrity.9 In this regard, chronic intake of diets rich in saturated fatty acids significantly increases Aβ synthesis, increasing its content in TRLs.10 Thus, repeated postprandial excursions may lead to extravasation of TRLs (among other molecules and toxins) into the brain.11 In fact, immunoreactivity studies have showed that plasma-derived apolipoprotein (Apo) B, which is a constituent protein of TRLs, colocalizes with Aβ plaques in the brain of APP/PS1 transgenic mice, an experimental model of AD.12
It has been suggested that this situation may be detected as an insult by the microglia, which represents the first line of defense of the central nervous system (CNS).13 Microglia are highly plastic cells, which can exist as distinct functional phenotypes depending on the environment. In the case of insult, the synthesis of pro-inflammatory cytokines and other inflammatory mediators is rapidly induced. This is a physiological process addressed to eliminate the origin of the damage and restore homeostasis, but it gets pathological when it turns chronic.14 Indeed, chronic inflammation in the brain is common in aging and contributes to neurodegenerative disease development.15
Bearing in mind that sustained hypertriglyceridemia can increase the susceptibility to diseases related to neuroinflammatory processes, and that microglia act as the primary cellular mediator in neuroinflammation, we hypothesized that modulation of microglia could be a target to reduce inflammatory processes associated with the postprandial period. In addition, we have proposed that lipophilic bioactive compounds with anti-inflammatory and antioxidant properties could be delivered to the CNS encapsulated in TRLs.
In this regard, we have previously reported that lab-made TRLs carrying lipophilic bioactive compounds present in pomace olive oil (POO), namely oleanolic acid (OA), β-sitosterol (BS) and α-tocopherol (AT), yielded a reduction in the expression and release of inflammatory mediators in activated microglial BV-2 cells.16 POO, obtained by chemical processes as a by-product of the virgin olive oil industry, is characterized by high levels of these bioactive components, all of them with proven anti-inflammatory and/or antioxidant properties both in vitro and in vivo.17–19
As the health benefits of olive oil gained recognition, the food industry responded by using high-oleic sunflower oil (HOSO), which was engineered through selection or genetic modification, mirroring oleic acid concentration in olive oil but differing in lipophilic bioactive minor components. In fact, the differences observed in the biological effects of POO and HOSO have been attributed to these bioactive components.20
In order to test whether these differences affect the ability to modulate the microglial inflammatory response, TRLs obtained from the serum of healthy young women after the intake of POO and HOSO were used to treat BV-2 cells.
2. Materials and methods
2.1. Design overview, settings and participants
The study was designed as a randomized crossover, double-blinded, controlled trial in the postprandial phase, performed from June to July 2020 in the Virgen del Rocío University Hospital (HUVR; Seville, Spain). Eligible participants were community-residing healthy women (aged 18–30 years) with normal-weight (BMI 19.0–24.0 kg m−2), who had fasting plasma triglyceride concentration in the normal range, and did not suffer from any digestive or metabolic disorders, as verified from their electronic medical record. Pregnant women and those within 3 months postpartum or breastfeeding were not included. Subjects with alcohol dependence, drug addiction and physical or intellectual limitations were also excluded. All participants signed their consent after being informed of the objectives and methodology of the trial.
2.2. Recruitment, intervention and follow-up
In June 2020, volunteers from the University environment were examined, identifying 18 women who fulfilled the requirements to be included in the trial. The participants were encouraged to avoid the consumption of alcoholic beverages and tobacco during the day prior to the execution of the trials. On test days, and after 12 hours of overnight fasting, blood samples were drawn from the cubital vein to determine general, liver, and kidney biochemical parameters. These determinations were carried out in the Clinical Biochemistry and Analysis Laboratory of the Virgen del Rocío University Hospital. Subsequently, the participants were randomly distributed into two groups of nine individuals. One group received 50 g of POO as part of an experimental breakfast that included 208 g of skimmed milk, 71 g of whole meal bread, and 20 g of mashed tomato, providing 2806 kJ of energy. Those assigned to the other group received the same amount of HOSO within the experimental meal. The POO was kindly donated by ORIVA, whereas the HOSO was purchased from the local market.
After eating the respective breakfasts, blood samples were drawn from the participants every hour, during a postprandial period of 6 hours. During this time, free access to water intake was allowed. After a first test session, a washout period of four weeks was established, and a second session was carried out, in which each study group ingested the other type of oil. With this study design, each participant is her own control.
2.3. Anthropometric measures of the participants
Anthropometric evaluations were individually conducted based on the guidelines from the International Society for the Advancement of Kinanthropometry (ISAK).21 For body measurements, a TANITA® BC-418MA body composition analyzer (West Drayton, UK) was utilized. Height was measured twice using a Seca 214* portable stadiometer, accurate to 5 mm. The average of these two measurements was used for the analysis. The calculation of body mass index (BMI) was done by dividing the weight in kilograms by the square of the height in meters. Waist circumference (WC) was measured by placing a Seca automatic roll-up measuring tape (with a precision of 1 mm) at the midpoint between the lowest rib and the upper edge of the iliac crest, at the conclusion of a normal exhalation. Blood pressure (BP) readings were obtained using a calibrated aneroid sphygmomanometer along with a Littmann® stethoscope (from Saint Paul, MN, USA), following internationally recognized recommendations.22
2.4. Clinical biochemistry of the participants
In order to provide a comprehensive understanding of the participants’ metabolic profile in the postprandial state, serum glucose, cholesterol, triglycerides, HDL cholesterol, LDL cholesterol, and Apo B were analyzed. Glucose, total cholesterol and triglycerides were determined by enzymatic colorimetric methods on a Roche/Hitachi System analyzer (Roche Diagnostics, Mannheim, Germany). Serum HDL cholesterol was measured by a direct enzymatic method (HDL-C-plus 2nd generation, Roche Diagnostics, Mannheim, Germany) on a Roche/Hitachi System analyzer (Roche Diagnostics) and LDL cholesterol was estimated by the Friedewald formula.23 Total lipoprotein Apo B (Apo B48 + Apo B100) was determined by automated immunoturbidimetric assays (Tinaquant; Roche Diagnostics, Mannheim, Germany). Fasting serum insulin was determined using an ELISA kit (Diaclone, Besançon, France) according to the manufacturer's instructions.
2.5. Chemical composition of POO and HOSO
The chemical composition of the experimental oils was analyzed to compare their distinct molecular properties, nutritional components, and bioactive compounds, aiming to understand how these variations might contribute to the observed effects.
2.5.1. Fatty acid composition.
The FA composition of the oils was determined according to the analytical method described in the European Union Commission Regulation 2568/91,24,25 after preparation of fatty acid methyl esters (FAME). FAME were injected into an Agilent 5890 series II gas chromatograph equipped with an automatic split injector and flame ionization detector (FID). A capillary silica column Supelcowax 10 (60 m × 0.25 mm I.D.; 0.25 μm stationary phase thickness) (Sulpelco, Co., Bellefonte, USA) with hydrogen as a carrier gas was used. The temperatures of the injector and detector were held at 250 °C. The oven was programmed at 180 °C (10 min), and then to increase at 2 °C min−1 rate until 250 °C. The injection volume was 1 μL. Compound identification was based on matching performed peak retention times with those of the standards FAME. The amount of an individual FAME was expressed as a percentage of the total.
2.5.2. Minor components’ analysis.
Tocopherols were determined according to the IUPAC Standard Method 2432
26 by HPLC using a Si-column (250 mm × 4 mm I.D.; 4 μm particle size). The elution solvent was a mixture of hexane
:
2-propanol (99
:
1, v/v) at a flow rate of 1 ml min−1. Detection was performed by fluorescence analysis (RF-10AXL Shimadzu fluorescence detector, Shimadzu, Columbia, MD, USA), setting excitation and emission at 290 and 330 nm wavelengths, respectively. For quantitative determinations, standards of tocopherols in hexane at concentrations of 1–6 μg ml−1 were prepared and injected.
Phytosterols contained in the oils were also determined according to the method published by the International Olive Oil Council.27 After separation from the unsaponifiable matter by thin layer chromatography on a silica gel plate, this fraction was analyzed by GC-FID, using an Agilent 5890 series II gas chromatograph, equipped with an automatic split injector and FID. An Agilent DB-5 capillary column (30 m × 0.25 mm ID; 0.25 μm stationary phase thickness) was used. The temperature was maintained at 260 °C, and that of the injector and the detector at 300 °C, hydrogen was used as carrier gas with a flow rate of 2 ml min−1, and the volume of the sample injected was 1 microliter. Individual sterol (including erythrodiol and uvaol) values were reported in percentage and total content in milligrams per 100 g of the oil.
For the determination of squalene, the procedure described by Lanzón et al.28 was used. The chromatographic conditions were those followed in the determination of FA. For quantification, using an external standard, a calibration curve was generated with standard squalene supplied by Sigma Chemical Co. (Saint Louis, MO, USA).
For the analysis of oleanolic acid, the procedure described by Pérez-Camino and Cert29 was used. The triterpenoid fraction of the oil was recovered by fractionated solid-phase extraction using a bonded aminopropyl phase cartridge.
For the analysis of the OA content, the fraction was silylated with hexamethyldisilazane
:
trimethylchlorosilane
:
anhydrous pyridine (3
:
1
:
9 v/v) and subjected to GC-FID analysis on an Agilent 6890N GC (Agilent Technologies, CA), equipped with a FID and a Rtx-65TG Crossbond capillary column (30 m × 0.25 mm I.D.; 0.1 mm film thickness) coated with 35% dimethyl-65% diphenyl polysiloxane as the stationary phase (Restek, Co., Bellefonte, PA). The injection was realized in split mode (1
:
5 split ratio), and hydrogen was used as a carrier gas (pressure at column head: 140 kPa). The oven temperature was initially established at 260 °C for 9 min and programmed to increase up to 280 °C at a rate of 10 °C min−1 and maintained at this value for 8 min. The injector and detector temperatures were isothermally established at 300 °C.
2.6. Isolation and characterization of human triglyceride-rich lipoproteins (TRLs)
Since bioactive lipophilic compounds may be transported in the postprandial phase as TRLs, we isolated these particles from blood aliquots drawn at 0, 2 and 4 hours of the postprandial period by ultracentrifugation, as described by Cabello-Moruno et al.8 Briefly, TRLs were isolated from 4 mL of serum by salt-gradient ultracentrifugation with 6 mL of a NaCl solution (density: 1006 g ml−1) at 356
000g (18 h at 15 °C). Ultracentrifugation was performed using a SW 41 Ti rotor in a Beckman L8-70M preparative ultracentrifuge (Beckman Instruments, Palo Alto, CA, USA).
2.7. BV-2 cells and TRL treatment
BV-2 brain microglial cells (available online: https://bioinformatics.hsanmartino.it/cldb/cl7130.html), kindly provided by Dr Alberto Pascual (Institute of Biomedicine of Seville, IBIS, Seville, Spain), were used as a microglial cell model. BV-2 (CVCL_0182) is a type of microglial cell derived from C57/BL6 mice that have been immortalized by v-raf/v-myc carrying J2 retrovirus. They have morphological, phenotypic and functional markers of macrophages. The cells were cultured in DMEM (Biowest, Nuaillé, France) supplemented with 10% heat-inactivated fetal bovine serum (FBS) (Biowest, Nuaillé, France) and antibiotics (100 U mL−1 penicillin and 100 U mL−1 streptomycin) (Biowest, Nuaillé, France), under a 100% humidified atmosphere of air + 5% CO2 at 37 °C. Cells were passaged every 2–3 days to maintain growth.
BV-2 cells were treated with postprandial TRLs, which were added according to their triglyceride concentration (0.15 μmol mL−1 culture medium). Buffered saline (0.9% w/w, pH 7.4) was used as a blank treatment, and 100 ng mL−1 LPS as the positive control. The assays were carried out in cultures with viability greater than 95%. Characterization of the microglial phenotype and gene expression were carried out in a medium reduced to 5% of FBS.
2.8. Inflammatory cytokine production
BV-2 cells were seeded at 5 × 105 cells per mL in 6-well plates and treated with postprandial TRLs (10 μM) for 24 h at 37 °C to examine the impact of POO and HOSO on the production of inflammatory cytokines. The culture media were collected, and IL-6, IL-1β and TNF-α production was measured using ELISA kits (Diaclone, Besancon, France) according to the manufacturer's instructions. Absorbance was measured at 450 nm using a scanning multiwell spectrophotometer (Multiskan spectrum, Thermo Fisher Scientific, Waltham, MA, USA).
2.9. Nitric oxide (NO) assay
NO serves as a key signaling molecule and inflammatory mediator within the CNS and is associated with its activation. Therefore, NO was determined in BV-2 cells that were seeded in a 96-well plate at a density of 5 × 105 cells per well and incubated overnight. Then, cells were treated with postprandial TRLs for 24 h at 37 °C. After the treatments, the culture supernatants were collected, and the NO concentration was measured using a Griess reagent assay kit (R&D Systems, Inc., Minneapolis, MN, USA), which measures the levels of accumulated nitrite, a NO metabolite. Absorbance at 540 nm was determined using a Multiskan spectrum spectrophotometer.
2.10. Glutathione assay
Since the measurement of glutathione is essential for understanding cellular antioxidant capacity and oxidative stress, we assessed the total glutathione level in the culture supernatants after TRL treatments, using a modification of the glutathione reductase recycling assay.30 In this assay, reduced glutathione (GSH) is oxidized by 5,50-dithiobis-(2-nitrobenzoic acid) (DTNB), resulting in the formation of oxidized glutathione (GSSG) and 5-thio-2-nitrobenzoic acid (TNB). GSSG is then reduced to GSH by glutathione reductase (GR) using reducing equivalents provided by NADPH. The rate of TNB formation is proportional to the sum of GSH and GSSG present in the cell culture sample and was determined at 412 nm with the Multiskan multiwell scanning spectrophotometer.
2.11. Gene expression
In order to provide insights into the underlying cellular mechanisms of the observed effects, gene expression of cytokines and NO was measured by reverse transcription-quantitative polymerase chain reaction (RT-qPCR) by means of a CFX96 Real-Time PCR system (Bio-Rad, Hercules, CA, USA). BV-2 cells were seeded at 5 × 105 cells per mL in six-well plates and treated with postprandial TRLs for 24 h at 37 °C. Total RNA was isolated using Trisure (Bioline, Meridian Life Science, Inc. Memphis, TN, USA) and reverse transcribed to cDNA using a synthesis kit (NZYTech, Lisboa, Portugal). Quantitative PCR was performed by means of a Precision Plus Mastermix kit containing SYBR Green (Primerdesign Ltd, Chandler's Ford, UK), according to the manufacturer's instructions and the following conditions: denaturation at 95 °C for 2 min, followed by 40 cycles at 95 °C for 15 s and at 60 °C for 60 s. The relative gene expression was quantified according to the 2−ΔΔCT method, and the results were expressed as fold difference with the control (TRLs) after normalization with hypoxanthine phosphoribosyltransferase (HPRT).
The following mouse primer sequences were used:
IL-1β,
forward GACCTTCCAGGATGAGGACA,
reverse AGCTCATATGGGTCCGACAG;
IL-6,
forward AGTTGCCTTCTTGGGACTGA,
reverse TCCACGATTTCCCAGAGAAC;
TNF-α,
forward AGTCCGGGCAGGTCTACTTT,
reverse GAGTTGGACCCTGAGCCATA;
iNOS,
forward CTCACTGGGACAGCACAGAA,
reverse GGTCAAACTCTTGGGGTTCA;
HPRT,
forward TGCTCGAGATGTCATGAAGG,
reverse TATGTCCCCCGTTGACTGAT.
2.12. Statistical analysis
All experiments were performed in triplicate, and the data were expressed as mean ± SD, unless otherwise stated. Statistical analyses were conducted using IBM SPSS Statistics 23.0 (IBM Corp., Armonk, NY, USA) software. Statistical significance was assessed by one- or two-way ANOVA, followed by Bonferroni's multiple-comparison tests or the Kruskal–Wallis test or Student's t-test, when applicable. Normality was assessed using the Kolmogorov–Smirnov test. Differences were considered statistically significant at p < 0.05.
3. Results
3.1. Baseline characteristics of the participants
In June 2020, a number of female volunteers were examined from the university academic environment, identifying 18 women who fulfilled the requirements to be included in the trial. The group of volunteers was well balanced with respect to all the variables considered (Table 1), including clinical characteristics.
Table 1 Anthropometric characteristics of the participants
|
Mean |
SD |
Weight (kg) |
57.2 |
6.1 |
Body fat (%) |
26.3 |
4.7 |
Height (cm) |
162.3 |
5.2 |
Waist circumference (cm) |
68.6 |
5.0 |
Hip circumference (cm) |
94.4 |
4.2 |
Fat mass (kg) |
15.4 |
3.2 |
Fat-free mass (kg) |
41.6 |
3.7 |
Total water (kg) |
30.4 |
2.7 |
Visceral fat (kg) |
1.47 |
0.61 |
BMI (kg m−2) |
21.4 |
2.1 |
Waist to hip ratio |
0.73 |
0.04 |
Waist to height ratio |
0.42 |
0.03 |
3.2. Chemical composition of POO and HOSO
The fatty acid (FA) composition of the experimental oils used in the study is shown in Table 2. Both oils were characterized by a similar FA profile, mainly composed of oleic acid (18:1 n-9), accompanied by significant contents of linoleic (18:2 n-6), palmitic (16:0) and stearic (18:0) acids. HOSO exhibited a three-fold higher level of palmitic acid than POO. Both oils showed similar concentrations of tocopherols. On the other hand, POO exhibited higher levels of phytosterols and, in contrast to HOSO, included high concentrations of squalene, oleanolic acid, uvaol and erythrodiol, which were absent in HOSO.
Table 2 Fatty acid composition (%) and minor components (mg kg−1) of the experimental oils
Fatty acid |
POO |
HOSO |
16:0 |
11.6 |
4.1 |
16:1 n-7 |
1.0 |
1.3 |
18:0 |
2.8 |
4.0 |
18:1 n-9 |
71.3 |
77.4 |
18:2 n-6 |
11.5 |
14.3 |
18:3 n-6 |
0.8 |
0.3 |
20:0 |
0.5 |
0.1 |
20:1 n-9 |
0.3 |
0.1 |
22:0 |
0.2 |
0.9 |
Minor components
|
Tocopherols |
389 |
393.2 |
Sterols |
2839 |
1497 |
Squalene |
799 |
— |
Oleanolic acid |
187 |
— |
Erythrodiol + uvaol |
638.5 |
— |
3.3. Effects of the experimental meals on the serum biochemistry of the participants during the postprandial phase
Glycemia remained stable throughout the postprandial period, with a relative maximum reaching 1 hour after the experimental breakfast intake (Fig. 1A). However, no statistically significant differences were found between both types of oils.
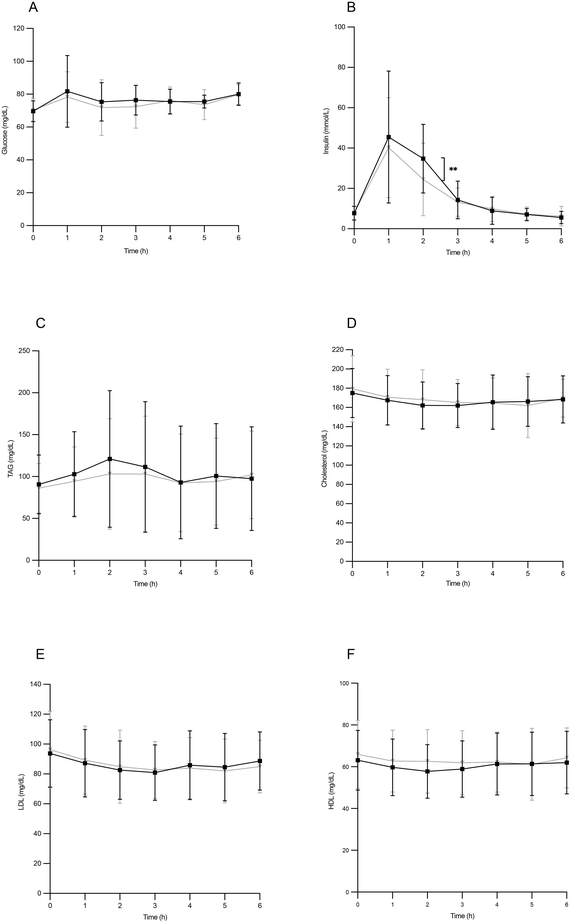 |
| Fig. 1 Postprandial glucose (A), insulin (B), triglycerides (C, TAG), cholesterol (D), LDL-cholesterol (E) and HDL-cholesterol (F) serum concentrations after the ingestion of the breakfast with HOSO (yellow) or POO (green). Values are expressed as mean ± SD. | |
The insulin peak concentration corresponded to the highest glycemic level, also exhibiting a maximum value 1 hour after ingestion. In this phase of the postprandial period, circulating insulin values were higher in individuals who ingested HOSO, showing significant differences at 2 hours (Fig. 1B). Serum triglycerides reached a relative maximum at two hours of the postprandial period, without reaching statistical significance between individuals ingesting HOSO or POO (Fig. 1C). Regarding plasma concentrations of total cholesterol, LDL-cholesterol and HDL-cholesterol, no significant changes were found throughout the entire postprandial period after the intake of either HOSO or POO (Fig. 1D–F). This result was expected, since it is known that cholesterol concentrations do not vary significantly in the postprandial phase.31
TRLs were isolated from the blood samples obtained before and after the intake of the meals, and their content in total apolipoprotein B (Apo B), which serves as an indicator of the TRL particle abundance, was assessed. Fig. 2 shows the total Apo B concentration before the meal ingestion and at two and four hours of the postprandial period. A steady decline of Apo B was observed throughout the postprandial phase, revealing the gradual TRL clearance. At all time points, the Apo B content in TRLs seemed to be slightly higher after the consumption of HOSO (TRL–HOSO), in comparison to that recovered after the POO (TRL–POO) intake, but differences were not significant.
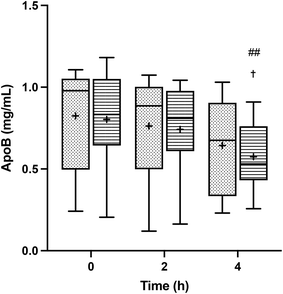 |
| Fig. 2 Postprandial ApoB serum concentrations after the ingestion of the breakfast with high-oleic sunflower oil (HOSO, points) or pomace olive oil (POO, stripes). Values are expressed as mean ± SD. ##p < 0.01 vs. 2 h; †p < 0.05 vs. 0 h. | |
3.4. Release of inflammatory cytokines by BV-2 cells treated with postprandial TRLs
The treatment of BV-2 cells with postprandial TRLs caused cell activation and elicited their inflammatory response. An increase in the release of IL-1β throughout the postprandial phase was observed with both oil types when compared to the saline treatment (negative control) (Fig. 3A). In addition, differences among the ingested oils were remarkable, with particles formed after HOSO intake (TRL–HOSO) producing a significantly higher IL-1β release in comparison with TRL–POO at the fourth hour of the postprandial period. At this time, the TRL–HOSO treatment overwhelms the response obtained by the exposure of BV-2 cells to 100 ng mL−1 LPS. These responses were associated well with the evolution of the IL-1β gene expression, which was differentially overstimulated by the treatment with TRL–HOSO (Fig. 4A).
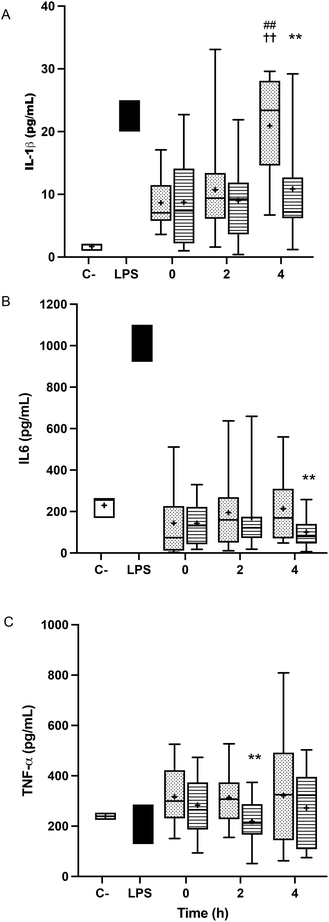 |
| Fig. 3 Cytokine release in BV-2 cells stimulated by lipopolysaccharides (LPS, positive control, black) or TRLs obtained at 0 h, 2 h and 4 h after the intake of high-oleic sunflower oil (HOSO, points) or pomace olive oil (POO, stripes) for 24 h: (A) interleukin-1 β (IL-1β); (B) interleukin-6 (IL-6); and (C) tumor necrosis factor-α (TNF-α); saline (0.9%, pH 7.4) was used as the negative control (white box). Values are expressed as mean ± SD of 18 independent experiments. **p < 0.01 vs. HOSO; ##p < 0.01 vs. 2 h; ††p < 0.01 vs. 0 h. | |
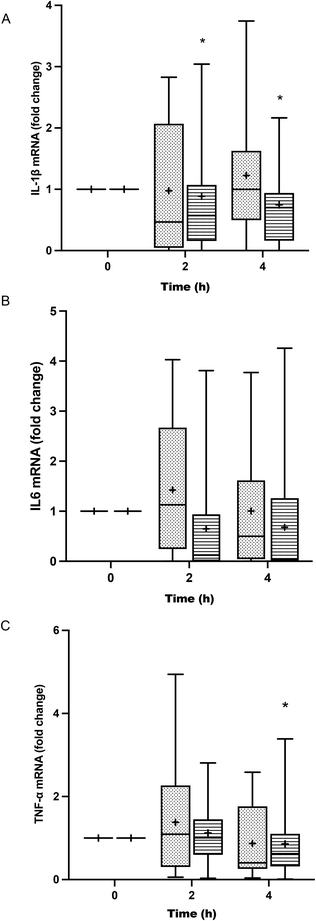 |
| Fig. 4 Cytokine RNA expression of cytokines in BV-2 cells stimulated by TRLs obtained at 0 h, 2 h and 4 h after the intake of high-oleic sunflower oil (HOSO, points) or pomace olive oil (POO, stripes) for 24 h: (A) interleukin-1 β (IL-1β); (B) interleukin-6 (IL-6); and (C) tumor necrosis factor-α (TNF-α). TRLs obtained at 0 h were used as reference. Values are expressed as mean ± SD of 18 independent experiments. *p < 0.05 vs. HOSO. | |
TRL treatment did not result in increased IL-6 release from BV-2 cells. In all cases, the levels of IL-6 in the culture medium remained similar to those generated by incubating the cells with saline solution (Fig. 3B). However, with TRLs isolated from plasma collected 4 h after ingestion of the experimental meal, significant differences were detected between both types of oils, with TRL–POO causing lower IL-6 production when compared with TRL–HOSO. The quantification of IL-6 gene expression levels in BV-2 cells treated with these TRLs, on the other hand, did not show differences among the oil types during the postprandial period (Fig. 4B).
Results for TNF-α were somehow similar to those obtained for IL-6 with concentrations at the level of the negative control or slightly higher (Fig. 3C). We found differences between TRL–POO and TRL–HOSO in the production of TNF-α by BV-2 treated cells. Treatment with TRL–POO showed lower concentrations of this cytokine in the medium at 2 hours postprandially. These results paralleled those of the gene expression of TNF-α (Fig. 4C).
3.5. Gene expression and release of nitric oxide in TRL-treated BV-2 cells
Fig. 5A shows that the treatment of BV-2 cells with postprandial TRLs gave rise to an increase in the release of NO by the cells, compared to the negative control, but that it was not as high as that of the aggression with LPS. NO production in TRL–POO treated cells was lower compared to TRL–HOSO, the difference being significant at 4 h. However, this effect could not be associated with iNOS gene expression (Fig. 5B), since, although a slight increase in the postprandial times studied was observed, no significant differences were found.
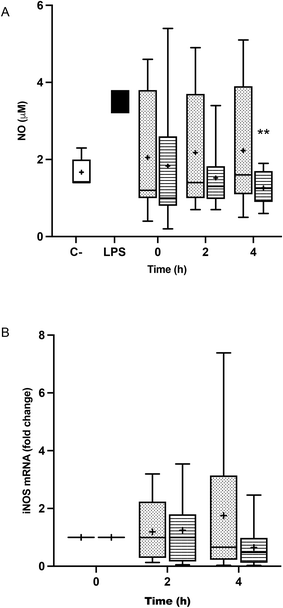 |
| Fig. 5 Nitrite production (A) and inducible nitric oxide synthase (B) (iNOS) gene expression in BV-2 cells stimulated by lipopolysaccharides (LPS, positive control, black) or TRLs obtained at 0 h, 2 h and 4 h after the intake of high-oleic sunflower oil (HOSO, points) or pomace olive oil (POO, stripes) for 24 h. Saline (0.9%, pH 7.4) was used as the negative control (white) in nitrite production experiments, and TRLs obtained at 0 h were used as reference in the gene expression experiments. Values are expressed as mean ± SD of 18 independent experiments. **p < 0.01 vs. POO. | |
3.6. Effect of the TRL treatment on the glutathione pool of BV-2 cells
Incubation with fasting and postprandial TRLs caused the decline of the glutathione pool of BV-2 cells, when compared with the level exhibited in the saline treatment. In fact, the treatment with the TRLs obtained from the plasma samples corresponding to the 2 and 4 hours of the postprandial period exceeded the decline in the glutathione level that is achieved when activating microglial cells with LPS. No significant differences between the two TRL types were observed (Fig. 6).
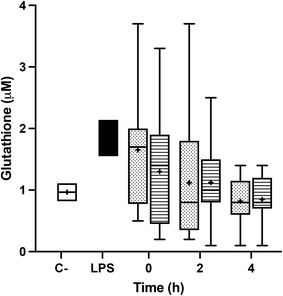 |
| Fig. 6 Intracellular concentrations of total glutathione (GSH + GSSG) in BV-2 cells stimulated by lipopolysaccharides (LPS, positive control, black) or TRLs obtained at 0 h, 2 h and 4 h after the intake of high-oleic sunflower oil (HOSO, points) or pomace olive oil (POO, stripes) for 24 h. Saline (0.9%, pH 7.4) was used as the negative control (white). Values are expressed as mean ± SD of 18 independent experiments. | |
4. Discussion
As a continuation of our previous research,16,32 we designed a crossover, randomized and controlled trial in the postprandial phase, with healthy young women, to assess the impact of the POO intake compared with that of HOSO. These oils share an almost identical FA content, but different minor components’ compositions (Table 2). We investigated the influence of TRLs isolated from the participants’ serum on the transactivation of BV-2 cells, a model of microglia.
Diet, and particularly the type of dietary fat, plays a crucial role in postprandial lipidemia.33,34 Certainly, a previous research study of our group demonstrated that the intake of oils with a similar FA content but different minor components influences differentially the TRL uptake rates by the liver35 and their clearance from blood,36 affecting both the composition37 and the size of the postprandial TRL particles in humans.8 The ratio TG/ApoB has been strongly correlated with the size of the TRL particles,38 which could be associated with their ability to penetrate into the arterial wall and activate monocytes/macrophages in atherosclerosis.39 It has also been proposed that an equivalent situation could be extrapolated to the extravasation of TRLs into the brain through the BBB,40 where they could play a critical role in the etiopathogenesis of certain neurodegenerative disorders.
Mamo et al.7 reported that the majority of plasma β-amyloids were associated with TRLs, encompassing chylomicrons, VLDL and IDL, and pointed out that plasma β-amyloids can compromise the blood–brain barrier, contributing to cerebrovascular alterations and amyloid angiopathy in AD. In this context, it has also been shown that the presence of TRLs in the brain, assessed as Apo B, colocalizes with Aβ.12 Nowadays, humans spend most of their time living in the postprandial state, which leads to a continuous and exaggerated exposure of the BBB to Aβ-containing TRLs.41,42 This might result in an elevated Aβ deposit in the brain and neuroinflammation. Aβ accumulation is toxic to neurons and exerts trophic effects on glial cells. Much evidence suggests that Aβ deposits trigger the inflammatory response.
In the present study, we report that human postprandial TRLs are able to activate BV-2 cells, which react, increasing the expression and release of inflammatory cytokines and NO. Our results show increased levels of IL-1β, IL-6 and TNF-α by these cells after the treatment with either TRL–POO or TRL–HOSO, in comparison with saline buffer as the negative control. IL-1β is considered a master regulator of the inflammatory process in the brain due to its ability to elicit the expression of other cytokine genes, such as TNF-α and IL-6, through the activation of the p38 MAPK/NF-κB axis.43,44 Furthermore, it has been shown that an increased TNF-α release by microglia can boost the activation of neighboring microglial cells in a positive feedback mechanism.45
In addition, we found that TRL particles obtained after the ingestion of POO yielded a lower release of these cytokines by microglial cells, when compared to those TRLs derived from the ingestion of HOSO. The differences in the IL-1β and IL-6 production became significant at 4 hours after the meal intake, and correlated well with the lower expression of the corresponding genes. This result suggests that bioactive compounds present in POO, but absent in HOSO, have the ability to modulate the pro-inflammatory response triggered by the microglial activation. The release of IL-1β was particularly high when cells were treated with TRLs originated after the intake of HOSO at time point 4 h, compared with POO, but also with those obtained at 2 h and at baseline. While the exact cause of this phenomenon remains uncertain, we could speculate that hydrolyzed TRLs produced after HOSO consumption could activate nuclear transcription factor-kappa B (NF-κB), triggering notable IL-1β production. TRL hydrolysis via lipoprotein lipase in the bloodstream is less specific for linoleic acid than for oleic acid (ref). Given the slightly higher presence of linoleic acid in HOSO, it is plausible that 4 h TRLs become substantially enriched with this fatty acid. Notably, previous work highlights the influence of postprandial time on TRL–cell interactions46 and that oleic acid and linoleic acid-enriched TRLs affect macrophages differently.47 In this context, existing evidence points to the potential of linoleic acid to amplify IL-1beta production in monocytes.48,49 Furthermore, Alzoghaibi et al.50 demonstrated that linoleic acid augmented IL-8 production in human intestinal smooth muscle cells from Crohn's disease patients, in contrast to oleic acid. These effects could be attributed to the ability of linoleic acid to enhance NFκB activation, which participates in IL-1β release.51
In a previous work,32 we demonstrated that OA, a natural component of POO, modulated the inflammatory response in LPS-activated BV-2 cells, reducing in a dose-dependent manner the IL-1β, IL-6 and TNF-α production. Additionally, in other earlier research studies,52 we treated THP-1 macrophages with human postprandial TRLs obtained after the intake of an OA-enriched olive oil, and found that the treatment reduced the IL-6 production. More recently, we also observed a moderate reduction in the expression and release of IL-6 in BV-2 cells treated with lab-made OA-containing TRLs.16 In agreement with these data, Han et al.53 proved that OA repressed the pro-inflammatory cytokine release in LPS-activated RAW 264.7 macrophages, suggesting the inhibition of the NF-κB transactivation by the triterpene as the underlying mechanism.
Other bioactive lipophilic compounds present in POO, such as AT and BS, have also demonstrated anti-inflammatory activity. In this regard, Akeson et al.54 observed an 80% reduction in the IL-1β release in PMA-activated THP-1 macrophages after incubation with AT. Furthermore, a trial performed by Devaraj and Jialal55 with diabetic patients revealed that supplementation with 1200 IU day−1 RRR-AT for 3 months resulted in a significant reduction of IL-1β and TNF-α levels (ca. 82%) in LPS-activated monocytes isolated from fasting blood from patients. Similar results were obtained by Van Tits et al.56 in LPS-activated PBMCs obtained from blood of both hypertriglyceridemic and normolipidemic subjects who received 600 IU day−1 AT for 6 weeks.
Moreover, Sun et al.57 described that BS decreased the IL-6 and TNF-α production by repressing their gene expression in LPS-activated BV-2 cells and LPS-activated primary microglia, proposing that this action was associated with the inhibition of the ERK, p38, and NF-κB pathways. Likewise, Choi et al.19 reported the decrease of the IL-1β, IL-6 and TNF-α production in LPS-activated RAW 264.7 macrophages pre-treated with BS. Also, Kyung-Ah et al.58 reported that treatment with BS modulated high-fat diet-induced colon inflammation in C57BL/6J mice, showing that phytosterols were able to reduce the release of pro-inflammatory cytokines via the inhibition of the NF-κB pathway.
NO is a signaling molecule involved in many physiological and pathological processes. NO can act as a vascular endothelium relaxing agent, a neurotransmitter and as an inhibitor of platelet aggregation, but is also generated as a stress factor during immune and inflammatory responses, being a toxic agent towards exogenous substances or particles.59 In the present research, the incubation of BV-2 cells with fasting TRLs caused a surge in NO release, which remained equally high in all the TRL–HOSO treatments. However, the treatment of BV-2 cells with the TRLs obtained after the ingestion of POO attenuated NO overproduction, bringing it down to the levels measured with saline (negative control). This effect of TRL–POO does not seem to be caused through the downregulation of iNOS expression, but likely via a post-translational process, since the iNOS gene was overexpressed when compared with saline in all the experiments involving either TRL–POO or TRL–HOSO.
In a previous study,32 we observed a reduced release of NO in LPS-activated BV-2 cells when they were pre-treated with 0.5–10.0 μM OA solutions in DMSO. This effect was accompanied by the down-regulation of the iNOS gene. In another recent study,16 we observed a reduction of 45% in NO production by BV-2 cells when synthetic TRLs contained 10 μM OA and 80% when the lipoprotein contained 10 μM BS.
In line with our results, Li et al.60 showed that OA reduced iNOS transcription and NO release in LPS-stimulated BV-2 cells. Likewise, Han et al.53 found that pre-incubation with OA and maslinic acid (MA) reduced NO secretion in LPS-activated RAW 264.7 macrophages. This was correlated with the decline of iNOS expression by the triterpene treatments. In murine macrophages, Márquez-Martínez et al.61 demonstrated that MA also attenuated NO production in a dose-dependent manner after stimulation with LPS, and concluded that the effect was not associated with direct inhibition of iNOS activity, but with gene downregulation.
Similar results were also provided by Namki Cho et al.,62 who assayed different oleanan type tritepenes from Tetrapanax papyrifer, displaying a reduction in NO synthesis in LPS-activated BV-2 cells. On the other hand, Sun et al.57 showed that BS induced a reduction of NO and iNOS in LPS-stimulated BV-2 cells and LPS-stimulated primary microglia.
GSH is the main non-protein thiol involved in antioxidant defense and redox homeostasis in all cells in vivo, including neurons.63 In the present study, we observed that the treatments with fasting and postprandial TRLs generate oxidative stress and decrease the GSH pool in BV-2 cells. Moreover, we found no significant differences between the two dietary oils introduced in the experimental meal. This finding was contrary to those of our earlier studies,16,32 in which we found that pre-treatment with OA or its inclusion, together with AT and BS, in the lipid core of lab-made TRLs restored the GSH levels to those determined in non-activated microglial cells. These discrepancies could be due to the fact that concentrations of these bioactive compounds within postprandial TRLs did not reach the threshold levels to exert significant effects on the GSH status. In this sense, it has been alleged that OA protected PC12 rat pheochromocytoma cells from H2O2-induced oxidative injury, enhancing the GSH content and SOD activity.64 Similar results were obtained by Vivancos and Moreno,65 who demonstrated that BS reverted GSH and GSSG levels, restoring the GSH/total glutathione ratio in PMA-stimulated RAW 264.7 macrophages. This action was associated with an enhancement of the SOD, GPx and CAT activities.
Previous studies had proposed that, compared to HOSO, POO enhances vascular function, glucose tolerance and obesity progression. González-Rámila et al.66 found that regular POO consumption reduced total cholesterol and LDL cholesterol levels, as well as waist circumference, in healthy and hypercholesterolemic subjects. In addition, we previously found that POO intake affects the size and composition of postprandial TRLs, which might have a relevant impact on their atherogenicity.8 This effect was related to the increased VLDL-receptor (VLDLr) gene expression in macrophages, which was associated with triggering inflammation after TRL uptake and foam cell formation.67 The differences were attributed to dissimilarities in triterpenic acid, squalene, and phytosterol levels, as well as the superior presence of oleic acid and antioxidant polyphenols in POO. These findings are in agreement with the results presented here.
In our study, we found no significant differences in postprandial glycemia between the oils. However, serum glucose increase was somehow lower than expected. This might be likely due to the low carbohydrate content of the meals. In addition, fiber in wholemeal bread might also influence postprandial glycemia, although recent studies suggest minimal effects.68
Both POO and HOSO are used in similar culinary and industrial applications, primarily in frying processes.69 Consequently, the slight differences in technological applications of these oils and in the quantity produced and consumed are unlikely to exert a substantial influence on the daily encounters of consumers.
The present work was designed as a crossover, double-blinded controlled trial in healthy women, based on an experimental meal with two dietary oils which only differ in their minor components. The high homogeneity of the studied female population and the double-blinded and crossover design should be considered as the strengths of this study. However, this study also has certain limitations that should be addressed. First, we did not analyze the minor component composition of TRLs. Therefore, we cannot confirm their presence in the lipoprotein particles. However, since we have compared two dietary oils with well-known compositions, we may assume that the different effects of these oils should be attributed to the differential presence of certain bioactive compounds. Secondly, the study included both in vivo and in vitro assays. While human research is based on the isolation of postprandial TRLs, the assessment of their antioxidant and anti-inflammatory activities were performed in microglial cell cultures, hence the effects of TRL–POO should not be strictly extrapolated to humans. In consequence, further dietary intervention trials with full human-based protocols are needed.
5. Conclusions
In conclusion, here we show for the first time that the intake of POO leads to the constitution of postprandial TRLs carrying lipophilic bioactive compounds that are able to modulate the inflammatory response elicited by microglial activation. We report that postprandial TRL–POO reduced the release of pro-inflammatory cytokines and NO and downregulated genes codifying for cytokines in BV-2 microglial cells. Moreover, the ingestion of POO by healthy women slightly improved glycemic control and TRL clearance throughout the postprandial phase. We have assayed POO effects in opposition to HOSO, a common dietary oil which shares with POO an almost identical FA composition but lacks certain biomolecules with recognized antioxidant and anti-inflammatory activities.
Author contributions
Conceptualization: J. S. P.; methodology: J. S. P. and J. M. C.; formal analysis and investigation: J. M. E., A. Q.-F., N. C., E. M., A. R.-R., J. M. C. and J. S. P.; resources: J. S. P. and J. M. C.; writing—original draft preparation: J. S. P., J. M. C. and J. M. E.; writing—review and editing: J. M. C., J. M. E. and J. S. P.; funding acquisition: J. S. P.; supervision: J. S. P. and J. M. C. All authors have read and agreed to the published version of the manuscript.
Conflicts of interest
JSP has received a speaker honorarium from Organización Interprofesional del Aceite de Orujo de Oliva. No other conflicts of interest are disclosed.
Acknowledgements
We are greatly thankful to Alberto Pascual (Institute of Biomedicine of Seville) for the donation of BV-2 microglial cells. This research was funded by 'Organización Interprofesional del Aceite de Orujo de Oliva' (ORIVA); Grant number 20191185 ORIVA.
References
-
2021 Alzheimer’s disease facts and figures, Alzheimer’s Dementia, 2021, 173, 327–406, DOI:10.1002/alz.12328.
- A. Serrano-Pozo, M. P. Frosch, E. Masliah and B. T. Hyman, Neuropathological alterations in Alzheimer disease, Cold Spring Harb. Perspect Med., 2011, 1(1), 1–24 CrossRef PubMed
.
- R. M. Ransohoff, How neuroinflammation contributes to neurodegeneration, Science, 2016, 353(6301), 168–175 CrossRef PubMed
.
- J. M. Perez Ortiz and R. H. Swerdlow, Mitochondrial dysfunction in Alzheimer's disease: Role in pathogenesis and novel therapeutic opportunities, Br. J. Pharmacol., 2019, 176(18), 3489–3507 CrossRef CAS PubMed
.
- E. Tönnies and E. Trushina, Oxidative Stress, Synaptic Dysfunction, and, Alzheimer's Disease, J. Alzheimer's Dis., 2017, 57(4), 1105–1121 Search PubMed
.
- V. Solfrizzi, C. Custodero, M. Lozupone, B. P. Imbimbo, V. Valiani and P. Agosti,
et al., Relationships of Dietary Patterns, Foods, and Micro- and Macronutrients with Alzheimer's Disease and Late-Life Cognitive Disorders: A Systematic Review, J. Alzheimer's Dis., 2017, 59(3), 815–849 CAS
.
- J. C. L. Mamo, L. Jian, A. P. James, L. Flicker, H. Esselmann and J. Wiltfang, Plasma lipoprotein β-amyloid in subjects with Alzheimer's disease or mild cognitive impairment, Ann. Clin. Biochem., 2008, 45(4), 395–403 CrossRef CAS PubMed
.
- R. Cabello-Moruno, J. S. Perona, J. Osada, M. Garcia and V. Ruiz-Gutierrez, Modifications in postprandial triglyceride-rich lipoprotein composition and size after the intake of pomace olive oil, J. Am. Coll. Nutr., 2007, 26(1), 24–31 CrossRef CAS PubMed
.
- T. M. Hsu and S. E. Kanoski, Blood-brain barrier disruption: Mechanistic links between western diet consumption and dementia, Front. Aging Neurosci., 2014, 6, 1–6 Search PubMed
.
- S. Galloway, R. Takechi, M. Nesbit, M. M. Pallebage-Gamarallage, V. Lam and J. C. L. Mamo, The differential effects of fatty acids on enterocytic abundance of amyloid-beta, Lipids Health Dis., 2019, 18(1), 1–6 CrossRef PubMed
.
- R. Takechi, S. Galloway, M. M. S. Pallebage-Gamarallage, V. Lam and J. C. L. Mamo, Dietary fats, cerebrovasculature integrity and Alzheimer's disease risk, Prog. Lipid Res., 2010, 49(2), 159–170 CrossRef CAS PubMed
.
- R. Takechi, S. Galloway, M. Pallebage-Gamarallage, C. Wellington, R. Johnsen and J. C. Mamo, Three-dimensional colocalization analysis of plasma-derived apolipoprotein B with amyloid plaques in APP/PS1 transgenic mice, Histochem. Cell Biol., 2009, 131(5), 661–666 CrossRef CAS PubMed
.
- E. Solito and M. Sastre, Microglia function in Alzheimer's disease, Front. Pharmacol., 2012, 3, 1–10 Search PubMed
.
- V. H. Perry, J. A. R. Nicoll and C. Holmes, Microglia in neurodegenerative disease, Nat. Rev. Neurol., 2010, 6(4), 193–201 CrossRef PubMed
.
- G. Sergio, Exploring the complex relations between inflammation and aging (inflamm-aging): Anti-inflamm-aging remodelling of inflamm- aging, from robustness to frailty, Inflammation Res., 2008, 57(12), 558–563 CrossRef CAS PubMed
.
- J. M. Espinosa, J. M. Castellano, S. Garcia-Rodriguez, A. Quintero-Flórez, N. Carrasquilla and J. S. Perona, Lipophilic Bioactive Compounds Transported in Triglyceride-Rich Lipoproteins Modulate Microglial Inflammatory Response, Int. J. Mol. Sci., 2022, 23(14), 7706 CrossRef CAS PubMed
.
- A. Marquez-Martin, R. D. La Puerta, A. Fernandez-Arche, V. Ruiz-Gutierrez and P. Yaqoob, Modulation of cytokine secretion by pentacyclic triterpenes from olive pomace oil in human mononuclear cells, Cytokine, 2006, 36(5–6), 211–217 CrossRef CAS PubMed
.
- M. Alcalá, I. Sánchez-Vera, J. Sevillano, L. Herrero, D. Serra and M. P. Ramos,
et al., Vitamin E reduces adipose tissue fibrosis, inflammation, and oxidative stress and improves metabolic profile in obesity, Obesity, 2015, 23(8), 1598–1606 CrossRef PubMed
.
- J. N. Choi, Y. H. Choi, J. M. Lee, I. C. Noh, J. W. Park and W. S. Choi,
et al., Anti-inflammatory effects of β-sitosterol-β-D-glucoside from Trachelospermum jasminoides (Apocynaceae) in lipopolysaccharide-stimulated RAW 264.7 murine macrophages, Nat. Prod. Res., 2012, 26(24), 2340–2343 CrossRef CAS PubMed
.
- J. S. Perona, C. Arcemis, V. Ruiz-Gutierrez and A. Catalá, Effect of dietary high-oleic-acid oils that are rich in antioxidants on microsomal lipid peroxidation in rats, J. Agric. Food Chem., 2005, 53(3), 730–735 CrossRef CAS PubMed
.
-
Arthur International Society for Advancement of Kinanthropometry, M. Marfell-Jones, T. Olds and H. De Ridder, International standards for anthropometric assessment 2011, International Society for the Advancement of Kinanthropometry, Lower Hutt, New Zealand, 3rd edn, 2011, p. 0620362073 Search PubMed
.
- T. G. Pickering, J. E. Hall, L. J. Appel, B. E. Falkner, J. W. Graves, M. N. Hill, D.W. Jones, T. Kurtz, S. G. Sheps and E. J. Roccella, Recommendations for Blood Pressure Measurement in Humans and Experimental Animals: Part 1: Blood Pressure Measurement in Humans: A Statement for Professionals from the Subcommittee of Professional and Public Education of the American Heart Association Council on High Blood Pressure Research, Hypertension, 2005, 45, 142–161 CrossRef CAS PubMed
.
- W. T. Friedewald, R. I. Levy and D. S. Fredrickson, Estimation of the concentration of low-density lipoprotein cholesterol in plasma, without use of the preparative ultracentrifuge, Clin. Chem., 1972, 18(6), 499–502 CrossRef CAS
.
- (EU) European Union, Commission Delegated Regulation (EU) 2022/2104 of 29 July 2022 supplementing Regulation (EU) No 1308/2013 of the European Parliament and of the Council as regards marketing standards for olive oil, and repealing Commission Regulation (EEC) No 2568/91 and Commission Implementing Regulation (EU) No 29/2012, Off. J. Eur. Union, 2022, 284/1(1234), L284/1–L284/22 Search PubMed
.
- Commission THEE, Commission implementing regulation (EU) 2022/2105 of 29 July 2022, Off. J. Eur. Union, 2022, 284/23(1234), 23–48 Search PubMed
.
-
Chemistry IU of P and A, Standard Methods for the Analysis of Oils, Fats and Derivatives, Blackwell Sci Publ., Oxford, 1987, 1st supple Search PubMed
.
- C.O.I. International Olive Council, Determination of the composition and content of sterols, triterpenic dialcohols and aliphatic alcohols by capillary column gas chromatography, Int. Olive Counc., 2020, Doc. No 26/Rev. 4, 1–31 Search PubMed
.
- A. Lanzón, Á. Guinda-Garín, T. Albi and C. de la Osa, Rapid method for squalene determination in vegetable oils (Spanish), Grasas Aceites, 1995, 46(4–5), 276–278 CrossRef
.
- M. C. Pérez-Camino and A. Cert, Quantitative determination of hydroxy pentacyclic triterpene acids in vegetable oils, J. Agric. Food Chem., 1999, 47(4), 1558–1562 CrossRef PubMed
.
- D. Giustarini, I. Dalle-Donne, A. Milzani, P. Fanti and R. Rossi, Analysis of GSH and GSSG after derivatization with N-ethylmaleimide, Nat. Protoc., 2013, 8(9), 1660–1669 CrossRef CAS PubMed
.
- J. S. Perona, J. Martínez-González, J. M. Sanchez-Domínguez, L. Badimon and V. Ruiz-Gutierrez, The Unsaponifiable Fraction of Virgin Olive Oil in Chylomicrons from Men Improves the Balance between Vasoprotective and Prothrombotic Factors Released by Endothelial Cells, J. Nutr., 2004, 134(12), 3284–3289 CrossRef CAS PubMed
.
- J. M. Castellano, S. Garcia-Rodriguez, J. M. Espinosa, M. C. Millan-Linares, M. Rada and J. S. Perona, Oleanolic acid exerts a neuroprotective effect against microglial cell activation by modulating cytokine release and antioxidant defense systems, Biomolecules, 2019, 9, 683 CrossRef CAS PubMed
.
- R. Cabello-Moruno, E. Martinez-Force, E. Montero and J. S. Perona, Minor components of olive oil facilitate the triglyceride clearance from postprandial lipoproteins in a polarity-dependent manner in healthy men, Nutr. Res., 2014, 34(1), 40–47 CrossRef CAS PubMed
.
- K. M. Rathnayake, M. Weech, K. G. Jackson and J. A. Lovegrove, Impact of meal fatty acid composition on postprandial lipaemia, vascular function and blood pressure in postmenopausal women, Nutr. Res. Rev., 2018, 31(2), 193–203 CrossRef CAS PubMed
.
- J. S. Perona, M. Avella, K. M. Botham and V. Ruiz-Gutierrez, Uptake of triacylglycerol-rich lipoproteins of differing triacylglycerol molecular species and unsaponifiable content by liver cells, Br. J. Nutr., 2006, 95(5), 889–897 CrossRef CAS PubMed
.
- R. Abia, J. S. Perona, Y. M. Pacheco, E. Montero, F. J. G. Muriana and V. Ruiz-Gutiérrez, Postprandial triacylglycerols from dietary virgin olive oil are selectively cleared in humans, J. Nutr., 1999, 129(12), 2184–2191 CrossRef CAS PubMed
.
- V. Ruiz-Gutierrez, J. S. Perona, Y. M. Pacheco, F. J. G. Muriana and J. Villar, Incorporation of dietary triacylglycerols from olive oil and high-oleic sunflower oil into VLDL triacylglycerols of hypertensive patients, Eur. J. Clin. Nutr., 1999, 53(9), 687–693 CrossRef CAS PubMed
.
- M. Amigo-Benavent, L. Sinausia, E. Montero and J. S. Perona, Brief Communication: Discordant ability of the triglyceride to apolipoprotein B ratio to predict triglyceride-rich lipoprotein particle size in normal-weight and obese men, Exp. Biol. Med., 2016, 241(16), 1772–1775 CrossRef CAS PubMed
.
- R. Carmena, P. Duriez and J. C. Fruchart, Atherogenic lipoprotein particles in atherosclerosis, Circulation, 2004, 109(23 SUPPL.), 2–7 Search PubMed
.
- M. M. S. Pallebage-Gamarallage, R. Takechi, V. Lam, S. Galloway, S. Dhaliwal and J. C. L. Mamo, Post-prandial lipid metabolism, lipid-modulating agents and cerebrovascular integrity: Implications for dementia risk, Atheroscler. Suppl., 2010, 11(1), 49–54 CrossRef CAS PubMed
.
- C. Potter, R. L. Griggs, J. M. Brunstrom and P. J. Rogers, Breaking the fast: Meal patterns and beliefs about healthy eating style are associated with adherence to intermittent fasting diets, Appetite, 2019, 133, 32–39 CrossRef PubMed
.
- M. P. Mattson, D. B. Allison, L. Fontana, M. Harvie, V. D. Longo and W. J. Malaisse,
et al., Meal frequency and timing in health and disease, Proc. Natl. Acad. Sci. U. S. A., 2014, 111(47), 16647–16653 CrossRef CAS PubMed
.
- A. Basu, J. K. Krady and S. W. Levison, Interleukin-1: A master regulator of neuroinflammation, J. Neurosci. Res., 2004, 78(2), 151–156 CrossRef CAS PubMed
.
- X. Liu, F. Ye, H. Xiong, D. N. Hu, G. A. Limb and T. Xie,
et al., IL-1β Induces IL-6 production in retinal Müller cells predominantly through the activation of P38 MAPK/NF-κB signaling pathway, Exp. Cell Res., 2015, 331(1), 223–231 CrossRef CAS PubMed
.
- J. P. Brás, J. Bravo, J. Freitas, M. A. Barbosa, S. Gomes-Santos, T. Summavielle and M.I. Almeida, TNF-alpha-induced microglia activation requires miR-342: impact on NF-kB signaling and neurotoxicity, Cell Death Dis., 2020, 11, 415 CrossRef PubMed
.
- K. Sato, Y. Takahashi, T. Takahashi, N. Katoh and Y. Akiba, Identification of factors regulating lipoprotein lipase catalyzed hydrolysis in rats with the aid of monoacid-rich lipoprotein preparations, J. Nutr. Biochem., 2002, 13(9), 528–538 CrossRef CAS PubMed
.
- A. Quintero-Flórez, L. Sinausia Nieva, A. Sánchez-Ortíz, G. Beltrán and J. S. Perona, The Fatty Acid Composition of Virgin Olive Oil from Different Cultivars Is Determinant for Foam Cell Formation by Macrophages, J. Agric. Food Chem., 2015, 63(30), 6731–6738 CrossRef PubMed
.
- D. Rothman, H. Allen, L. Herzog, A. Pilapil, C. M. Seiler and R. B. Zurier, Effects of unsaturated fatty acids on interleukin-1beta Production by human monocytes, Cytokine, 1997, 9(12), 1008–1012 CrossRef CAS PubMed
.
- C. A. Dinarello, Interleukin-1β and the Autoinflammatory Diseases, N. Engl. J. Med., 2009, 360(23), 2467–2470 CrossRef CAS PubMed
.
- M. A. Alzoghaibi, S. W. Walsh, A. Willey, I. A. Fowler and M. F. Graham, Linoleic acid, but not oleic acid, upregulates the production of interleukin-8 by human intestinal smooth muscle cells isolated from patients with Crohn's disease, Clin. Nutr., 2003, 22(6), 529–535 CrossRef CAS PubMed
.
-
B. Arneth, Tumor microenvironment, Medicina, Lithuania, 2020, vol. 56 Search PubMed
.
- Á. Fernández-Aparicio, J. S. Perona, J. M. Castellano, M. Correa-Rodríguez, J. Schmidt-Riovalle and E. González-Jiménez, Oleanolic acid-enriched olive oil alleviates the interleukin-6 overproduction induced by postprandial triglyceride-rich lipoproteins in thp-1 macrophages, Nutrients, 2021, 13(10), 1–17 CrossRef PubMed
.
- Y. Han, C. Yuan, X. Zhou, Y. Han, Y. He, J. Ouyang, W. Zhou, Z. Wang, H. Wang and G. Li, Anti-inflammatory activity of three triterpene from hippophae rhamnoides l. In lipopolysaccharide-stimulated raw264.7 cells, Int. J. Mol. Sci., 2021, 22, 12009 CrossRef CAS PubMed
.
- A. L. Akeson, C. W. Woods, L. B. Mosher, C. E. Thomas and R. L. Jackson, Inhibition of IL-1β expression in THP-1 cells by probucol and tocopherol, Atherosclerosis, 1991, 86(2–3), 261–270 CrossRef CAS PubMed
.
- S. Devaraj and I. Jialal, Low-density lipoprotein postsecretory modification, monocyte function, and circulating adhesion molecules in type 2 diabetic patients with and without macrovascular complications: the effect of alpha-tocopherol supplementation, Circulation, 2000, 102(2), 191–196 CrossRef CAS PubMed
.
- L. J. Van Tits, P. N. Demacker, J. De Graaf, H. L. Hak-Lemmers and A. F. Stalenhoef, α-Tocopherol supplementation decreases production of superoxide and cytokines by leukocytes ex vivo in both normolipidemic and hypertriglyceridemic individuals, Am. J. Clin. Nutr., 2000, 71(2), 458–464 CrossRef CAS PubMed
.
- Y. Sun, Y. Sun, Y. Sun, L. Gao, L. Gao and L. Gao,
et al., β-Sitosterol Alleviates Inflammatory Response via Inhibiting the Activation of ERK/p38 and NF-κ B Pathways in LPS-Exposed BV2 Cells, BioMed Res. Int., 2020, 2020, 7532306 Search PubMed
.
- K. A. Kim, I. A. Lee, W. Gu, S. R. Hyam and D. H. Kim, β-Sitosterol attenuates high-fat diet-induced intestinal inflammation in mice by inhibiting the binding of lipopolysaccharide to toll-like receptor 4 in the NF-κB pathway, Mol. Nutr. Food Res., 2014, 58(5), 963–972 CrossRef CAS PubMed
.
- S. Moncada, R. M. Palmer and E. A. Higgs, Nitric oxide: physiology, pathophysiology, and pharmacology, Pharmacol. Rev., 1991, 43(2), 109–142 CAS
.
- X. Li, G. Wu, M. Li and Z. Zhang, Oleanolic acid administration alleviates neuropathic pain after a peripheral nerve injury by regulating microglia polarization-mediated neuroinflammation, RSC Adv., 2020, 10(22), 12920–12928 RSC
.
- A. Márquez Martín, R. de la Puerta Vázquez, A. Fernández-Arche and V. Ruiz-Gutiérrez, Supressive effect of maslinic acid from pomace olive oil on oxidative stress and cytokine production in stimulated murine macrophages, Free Radical Res., 2006, 40(3), 295–302 CrossRef PubMed
.
- N. Cho, E. H. Moon, H. W. Kim, J. Hong, J. A. Beutler and S. H. Sung, Inhibition of Nitric Oxide Production in BV2 Microglial Cells by Triterpenes from Tetrapanax papyriferus, Molecules, 2016, 21, 459 CrossRef PubMed
.
- A. Pastore, G. Federici, E. Bertini and F. Piemonte, Analysis of glutathione: Implication in redox and detoxification, Clin. Chim. Acta, 2003, 333(1–2), 19–39 CrossRef CAS PubMed
.
- Z. T. Rong, X. J. Gong, H. B. Sun, Y. M. Li and H. Ji, Protective effects of oleanolic acid on cerebral ischemic damage in vivo and H2O2-induced injury in vitro, Pharm. Biol., 2011, 49(1), 78–85 CrossRef CAS PubMed
.
- M. Vivancos and J. J. Moreno, β-Sitosterol modulates antioxidant enzyme response in RAW 264.7 macrophages, Free Radicals Biol. Med., 2005, 39(1), 91–97 CrossRef CAS PubMed
.
- S. González-Rámila, R. Mateos, J. García-Cordero, M. A. Seguido, L. Bravo-Clemente and B. Sarriá, Olive Pomace Oil versus High Oleic Sunflower Oil and Sunflower Oil: A Comparative Study in Healthy and Cardiovascular Risk Humans, Foods, 2022, 11, 2186 CrossRef PubMed
.
- R. Cabello-Moruno, L. Sinausia, E. Montero, K. M. Botham, M. Avella and J. S. Perona, Minor components of pomace olive oil enhance VLDL-receptor expression in macrophages when treated with postprandial triglyceride-rich lipoproteins, Grasas Aceites, 2015, 66, e096 CrossRef
.
- A. Colombo, Y. Xu and H. Dong, Postprandial glycaemic response to white and wholemeal bread consumption between normal weight and overweight/obese healthy adults, Proc. Nutr. Soc., 2023, 82(OCE1), 100103 CrossRef
.
- F. Holgado, M. V. Ruiz-Méndez, J. Velasco and G. Márquez-Ruiz, Performance of olive-pomace oils in discontinuous and continuous frying. Comparative behavior with sunflower oils and high-oleic sunflower oils, Foods, 2021, 10, 3081 CrossRef CAS PubMed
.
|
This journal is © The Royal Society of Chemistry 2023 |
Click here to see how this site uses Cookies. View our privacy policy here.