DOI:
10.1039/D3FO01436C
(Paper)
Food Funct., 2023,
14, 6226-6235
Efficacy of pectins with different degrees of methyl-esterification and of blockiness in preventing gut epithelial cell barrier disruption and the impact on sodium–glucose co-transporter expression under low and high glucose conditions†
Received
11th April 2023
, Accepted 7th June 2023
First published on 9th June 2023
Abstract
Pectins support intestinal barrier function and have anti-diabetic effects, and can differ in the degree of methyl-esterification (DM) and the distribution of non-esterified galacturonic acid residues (DB). The mechanisms and effects of pectin type at different glucose levels are unknown. Pectins with different DM/DB on T84 cells were tested in the presence and absence of the barrier disruptor A23187 at 5 mM and 20 mM glucose. DM19 and DM43 pectins with high DB do rescue the intestinal barrier from disruption. Their effects were as strong as those of the barrier-rescuing anti-diabetic drug metformin, but effects with metformin were restricted to high glucose levels while pectins had effects at both low and high glucose levels. At high glucose levels, DM43HB pectin, which enhanced trans-epithelial electrical resistance, also increased the expressions of claudin1, occludin, and ZO-1. Low and high DM pectins decrease the apical expression of the sodium–glucose co-transporter (SGLT-1) and thereby influence glucose transport, explaining the anti-diabetogenic effect of pectin. Higher DB pectins had the strongest effect. Their impact on SGLT-1 was stronger than that of metformin. Pectin's rescuing effect on barrier disruption and its impact on glucose transportation and anti-diabetogenic effects depend on both the DB and the DM of pectins.
1. Introduction
Dietary fiber intake has a profound impact on human health. Numerous studies have shown that enhancing the intake of dietary fiber has beneficial effects on lowering the frequency of obesity, inflammatory bowel disease, and auto-immune diseases as well as e.g., on blood glucose levels in patients with type 2 diabetes mellitus.1–3 Many mechanisms have been proposed to explain the benefits of dietary fiber. Improved gut barrier function is one of these dietary fiber-induced health benefits. This enhanced barrier function can contribute to a reduced entry of pathogenic organisms and lower the passage of toxic molecules, and it can even modulate immune function.4
The intestinal barrier is considered the gatekeeper of the human body. It consists of an epithelial cell layer connected by tight junctions.5,6 By regulating the permeability of the epithelial layer, immune processes can be tuned by allowing or preventing the passage of antigens. Also, the regulation of tight junctions can modify nutrient absorption and prevent the transport of harmful components to the bloodstream.7 Nutrients may also have an impact on gut barrier function. It has for example been shown that prolonged exposure to too high glucose levels might induce intestinal barrier dysfunction through alteration of tight junctions’ integrity, which might result in the influx of harmful microbial components.8 Chronic hyperglycemia occurring in diabetic patients may lead to prolonged barrier disruption with enhanced inflammation and dysbiosis of the microbiome and associated health issues as a consequence.9 Prevention of barrier disruption may therefore be an essential focus for preventing gastrointestinal issues in patients with diabetes.
Pectin is a type of dietary fiber recognized for its many health benefits including effects on gut barrier function.10 Pectins are mostly extracted from plant peels and consist of homo-galacturonan, rhamnogalacturonan I and rhamnogalacturonan II. Homogalacturonan consists of 1,4-D-galacturonic acid where the ester groups can be methyl-esterified. The proportion of esters in the GalA backbone is expressed as DM.11 We have shown that the degree of methyl esterification has a strong impact on the health benefits of pectins in which lower DM pectins have a stronger effect on several health parameters than higher DM pectins. DB refers to the distribution of non-esterified GalA residues in the pectin backbone. High DB pectins have a more blockwise distribution of non-esterified GalA, while low DB pectins have a more random distribution of non-esterified GalA. Both DM and DB influence immune health parameters by attenuating TLR2-1 dependent inflammatory responses.12
Pectins have been shown to have many beneficial features, such as supporting gut barrier function, immunity, and metabolism, and reducing obesity.13,14 Also, pectins have been suggested to have anti-diabetogenic effects, but the mechanisms remain to be identified.15 Recently, we have shown that lower DM pectins can enhance the viability of insulin-producing cells by enhancing and stimulating mitochondrial health.16 However, another mechanism that has not been explored yet is that pectin might impact gut epithelial barrier function. It has been reported that the intestinal epithelium is a sensor that can sense luminal nutrients and regulate entry accordingly.17 We hypothesize that regulation of this process by pectins might be a mechanism for its anti-diabetogenic effects, and insight into how pectin structures are responsible for such an effect might contribute to the design of pectin-based strategies to lower hyperglycemia in type-2 diabetic subjects.
In the current study, we investigated and compared the effects of pectins with different DM and DB on the protection of gut barrier function disturbed by A23187. Metformin, which is applied to regulate glucose uptake in type 2 diabetic patients, is known to impact gut barrier function.18 It was therefore used to compare the effects of pectins on gut barrier function. The experiments were performed at low (5 mM) and high (20 mM) glucose concentrations to decipher the impact of hyperglycemia and the efficacy of pectins under these different conditions. First, six lemon pectins, i.e., DM18LB, DM19HB, DM49LB, DM43HB, DM84LB and DM88HB were studied with trans-epithelial electrical resistance (TEER) on the barrier formed by T84 cells in the presence or absence of the barrier disruptor A23187. Next, we studied the gene expression of tight junctions claudin1, claudin3, occludin, and ZO-1 in T84 cells and we determined whether the pectins impacted glucose transporters in T84 cells.
2. Materials and methods
2.1 Cell culture
T84 human colon carcinoma cells (ATCC, USA) were cultured in glucose-free DMEM (Merck, Germany) with 10% heat-deactivated fetal bovine serum (Merck, Germany), 50 mg mL−1 gentamycin (Lonza, Belgium), 5 mM glucose and 15 mM HEPES (Gibco, the Netherlands). T84 cells were cultured at 37 °C under a 5% CO2 condition and the medium was refreshed every other day. T84 cells used in this experiment were from passages 19–30. T84 cells were cultured for 21 days until confluence was reached and used in the experiments.
2.2 Structure determination of the pectin samples
Pectins of lemon origin were purchased from CPKelco (Lille Skensved, Denmark). All pectin samples were tested for contamination with endotoxins by applying a Limulus amebocyte assay (PYROGENT-5000, Lonza, the Netherlands), which was performed according to the manufacturer's protocol. Pectin samples were only used when the endotoxin levels were lower than the detection level of 0.1 μg L−1. Pectins were filtered through a charcoal filter to remove possible endotoxins in case they were found. The chemical structure of the pectins was determined as reported.12 In short, pectins were pre-hydrolyzed with 72% (w/w) H2SO4 at 30 °C for 1 h followed by treatment with 1 M H2SO4 at 100 °C for 3 h for the neutral sugar composition analysis. Pectin lyase (EC 4.2.2.10, CPKelco, Denmark) and endopolygalacturonase (CPKelco, Denmark) from Kluyveromyces fragilis were used for the galacturonic acid backbone hydrolysis. The pectin digests were analyzed using an ICS5000 high-performance anion exchange chromatography (HPAEC) system with a pulsed amperometric detector (PAD, ICS5000 ED) (Dionex Corporation, Sunnyvale, CA, USA) equipped with a CarboPac PA-1 column (250 mm × 2 mm i.d.) and a CarboPac PA guard column (25 mm × 2 mm i.d.). Each pectin was saponified using 1 ml of 0.1 M NaOH at 4 °C for 1 h and kept overnight at room temperature. After that DM was determined with the head-space gas chromatography method. The amount of mono-, di- and triGalA of pectins was determined by HPAEC-PAD and the degree of blockiness was calculated as the number of mono-, di- and tri-GalA residues related to the total amount of GalA residues. The monosaccharide composition was determined by gas–liquid chromatography.19 The molecular weight of the pectin samples was measured using high-pressure size exclusion chromatography. The composition of the pectin samples is shown in Table 2.
To support the data obtained with the analytical methods, liquid-state NMR analysis was performed. The pectin samples (19 mg) were suspended in 0.75 mL of D2O and 10 μL of acetone. 1H-NMR and 1H–13C-HSQC spectra were recorded on a Bruker spectrometer (500, 125 MHz) in a 5 mm tube at 80 °C using D2O as solvent. 1H and 13C chemical shifts are reported with HDO (δ4.23 ppm at 80 °C) and acetone (δ 2.22 for 1H and δ 30.89, δ 215.94 for 13C) as an internal reference.
2.3 Transepithelial electrical resistance measurements
Before seeding the T84 cells, electric cell–substrate impedance sensing (ECIS) plates (Applied BioPhysics, USA) were coated with 2 mg ml−1L-cystine (Merck, Germany) for half an hour and then washed with DMEM (Merck, Germany) twice. Subsequently, the plate was coated with 0.1% bovine serum albumin (Sigma, the Netherlands) and 1% PureCol collagen (Nutacon, Australia) in DMEM at room temperature for 24 hours. After that, T84 cells were seeded in the plate at a cell density of 104 per well. The cells were then cultured at 37 °C with 5% CO2 and the culture medium was changed every other day. Before the start of the experiment, the ECIS plate was refreshed with a medium containing either 5 mM or 20 mM glucose and then placed in the ECIS machine (Applied BioPhysics, USA) for at least 5 hours for stabilization. After that, the plate was incubated with the six different pectin samples at 2 mg ml−1 or 1 mM metformin (Merck, the Netherlands) for 24 hours. Afterward, the cells were treated with 6 μM gut epithelial cell barrier disruptor A23187 (Merck, Germany) for another 24 hours. The resistance at 400 Hz was measured during the whole experiment for analysis.
2.4 Immunofluorescence staining
T84 cells were seeded on 16 mm coverslips (VWR, the Netherlands) in a 12 well plate at a density of 105 cells per well. The medium was refreshed every other day and cultured for 3 weeks until the cells were confluent. Before the start of the experiment, the medium was refreshed with either 5 mM glucose or 20 mM glucose medium. Then, the T84 cells were pre-incubated with either of the six pectins or metformin for 24 hours. After that, the cells were exposed to the gut epithelial cell barrier disruptor A23187 for 24 hours. Subsequently, the coverslips were collected for immunofluorescence staining. The coverslips were washed 3 times with PBS for 5 minutes. The cells were then fixed in 4% paraformaldehyde at room temperature for 10 minutes, after which the cells were again washed with PBS 3 times. The cells were then incubated with 0.1% Triton-X100 (Sigma, the Netherlands) at 37 °C for 15 minutes and subsequently blocked with 1% bovine serum albumin at room temperature for 1 hour. Afterward, the cells were incubated overnight with a primary antibody against the sodium–glucose cotransporter-1 (SGLT1, 1
:
500, Merck, the Netherlands) at 4 °C. The cells were washed three times and then incubated with the secondary antibody Alex555 (1
:
500, Thermo Fisher, the Netherlands). After washing three times, the cells were stained with 4′,6-diamidino-2-phenylindole (DAPI, Merck, the Netherlands) for 10 minutes. After washing 3 times with PBS, the coverslips were mounted with Citifluor (Electron Microscopy Sciences, USA). Then, the slides were scanned using a Leica SP8 confocal microscope (Leica, Germany) with a 40×/1.3 oil differential interference contrast objective. The Z stack size was 1 μm for every step and 5 images per group were collected for analysis.
2.5 RNA isolation, reverse transcription, and expression
Total RNA isolation was performed according to the manufacturer's protocol. RevertAid reverse transcriptase (Thermo Fisher, Lithuania) was used for cDNA synthesis. ZO-1, claudin 1, claudin 3, and occludin expression were analyzed. cDNA samples were mixed with the primers shown in Table 1 and used with SYBR® Green real-time PCR master mix (Roche, Germany) to quantify the expression. PCR plates (384-well, Thermo Scientific, UK) and the ViiA7 real-time PCR system (Applied Biosystems, USA) were used in this experiment. Housekeeping gene GADPH was used to normalize the expression levels. The 2−ΔΔCt method was used for analyzing the fold changes in gene expression.
Table 1 The primers of tight junction genes
Gene |
Forward (5′–3′) |
Reverse (5′–3′) |
ZO-1 |
TGCCTCTGAGAGAGACGACA |
TCTCTACTCCGGAGACTGCC |
Claudin1 |
GATGAGGTGCAGAAGATGAG |
GGACAGGAACAGCAAAGTAG |
Claudin3 |
CTGCTCTGCTGCTCGTGTC |
CGTAGTCCTTGCGGTCGTAG |
Occludin |
CTATAAATCCACGCCGGTTC |
TATTCCTGTAGGCCAGTGTC |
GADPH |
AGCCACATCGCTCAGACAC |
GCCCAATACGACCAAATCC |
2.6 Statistical analysis
The results were processed with GraphPad Prism. Normal distribution was confirmed using the Kolmogorov–Smirnov test. Data that were not normally distributed were log-transformed before analysis. One-way ANOVA was used for statistical comparisons and post-testing was performed with Tukey's test to test statistical differences between groups (*p < 0.05 was considered statistically significant; *p < 0.05, **p < 0.01, ***p < 0.001, ****p < 0.0001).
3. Results
3.1 Chemical characterization of the pectins
All the lemon pectins were characterized for the DB, DM, sugar composition, and molecular weight (Mw). The chemical characteristics of the pectins are shown in Table 2 and Fig. 1. The pectins all revealed a similar ratio of monosaccharide units and showed a molecular weight distribution between 70 and 114 kDa. The DM ranged from DM18 to DM88 and the DB of pectins varied from DB33 to DB94. The pectins DM19 and DM18, DM43 and DM49, and DM84 and DM88 have similar DM but different DB.
Table 2 Composition of tested pectins. Data were also used in ref. 12
Name |
DB (%) |
M
w (kDa) |
Sugar composition (mol%) |
Carbohydrate content (w/w%) |
Rha |
Ara |
Gal |
Glc |
UA |
DM18LB |
86 |
78 |
1 |
0 |
2 |
0 |
97 |
62 |
DM19HB |
94 |
75 |
1 |
1 |
3 |
0 |
95 |
63 |
DM49LB |
33 |
114 |
0 |
1 |
2 |
0 |
96 |
73 |
DM43HB |
60 |
79 |
0 |
0 |
0 |
0 |
99 |
77 |
DM84LB |
71 |
113 |
1 |
5 |
6 |
1 |
87 |
65 |
DM88HB |
91 |
91 |
1 |
3 |
5 |
0 |
91 |
67 |
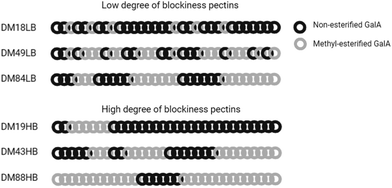 |
| Fig. 1 Schematic structure of pectins. | |
3.2 Determination of methylated GalA by NMR analysis
The peaks for the α-linked galacturonic acid were easily identified in the NMR spectra, and the 1H-NMR spectrum of DM19HB was used to assign the peaks of α-GalA (H-1 at 5.07, H-2 at 3.73, H-3 at 3.97, H-4 at 4.41 and H-5 at 4.71 ppm). In addition, 13C-NMR values were deduced from the HSQC measurement (C-1 at 99.8, C-2 at 69.1, C-3 at 69.5, C-4 at 78.9 and C-5 at 71.9 ppm). The NMR analysis corroborated the presence of α-linked galacturonic acid (GalA) as the major carbohydrate residue in the pectin samples; the NMR spectroscopy results are shown in the ESI Fig. 2.† The GalA methyl ester (OCH3) signals were observed at 3.79 ppm (1H) and 53.4 ppm (13C) (Table 3). When the amount of methyl ester was compared to the anomeric UA signal (Table 3), an increasing ratio was observed, indicating that the degree of methylation was in accordance with the values determined by HPAEC-PAD (Section 3.1).
Table 3 The ratio of H-1-GalA and methyl ester, as determined by 1H-NMR analysis
Pectin |
Area H-1-GalA peak |
Area methyl-ester peak |
Ratio GalA: methyl-esters |
DM18LB |
0.91 |
0.62 |
1 : 0.7 |
DM19HB |
0.91 |
0.60 |
1 : 0.7 |
DM49LB |
0.91 |
1.70 |
1 : 1.5 |
DM43HB |
0.91 |
1.00 |
1 : 1.1 |
DM84LB |
0.91 |
2.43 |
1 : 2.7 |
DM88HB |
0.91 |
2.55 |
1 : 2.8 |
3.3 Glucose concentration dependent protective effects of metformin and pectins on A23187 induced barrier disruption
Lower DM pectins have been shown to protect gut epithelial cells against barrier disruption,20 but the effects of the distribution of methyl groups are unknown. It is also unknown whether pectins have similar efficacy in protecting against barrier disruption compared to pharmacological agents that are known to protect against epithelial barrier disruption such as metformin.18 Metformin is an anti-diabetogenic drug that might work differently under low and high glucose circumstances.21 Therefore, all tests and comparisons on the efficacy of pectins and metformin in protecting against barrier disruption were performed under 5 mM glucose and 20 mM glucose circumstances.
Fig. 2A and B show the rescuing effects of metformin and pectins on A23187-induced epithelial barrier disruption. To visualize and compare the effects, the areas under the curve of TEER were calculated and are compared in Fig. 2C and D. Pectins as such had no effect on gut epithelial barrier integrity in unstressed T84 epithelial cells. This was the same under culture conditions with low (5 mM) and high (20 mM) glucose levels (Fig. 2C and D, left). However, this was different when the cells were treated with the barrier disruptor A23187. The barrier disruptor had a much stronger effect under 5 mM glucose conditions compared to 20 mM glucose conditions. Under 5 mM glucose culture conditions and exposure to A23187, the protective effects of metformin were absent but they were pronounced with pectins. There was not a very strong effect of the DM of pectins at 5 mM glucose as the lower DM18LB and DM19HB, as well as the intermediate DM49LB and DM43HB and higher DM84LB, had similar protective effects and AUCs of 69.8 ± 9.4% (p < 0.0001), 52.0 ± 5.9% (p < 0.01), 57.0 ± 8.4% (p < 0.001), 70.2 ± 7.6% (p < 0.0001), and 63.4 ± 6.6% (p < 0.0001), respectively. There was also an effect of DB as DM43HB had a 70.2 ± 7.6% AUC (p < 0.0001), which was higher than that of DM49LB which was 57.0 ± 8.5% (p < 0.001). However, no DB-dependent difference in barrier protective effects was observed with pectins with a low DM (DM19) or high DM (DM86).
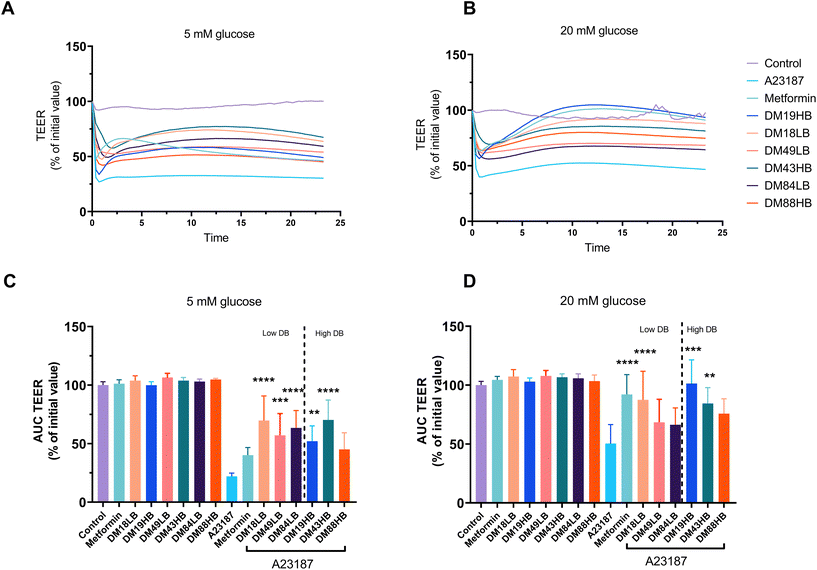 |
| Fig. 2 Protective effect of metformin and pectins with different DM and DB on the TEER in the presence and absence of the gut epithelial cell barrier disruptor A23187 in T84 cells. The TEER of T84 cells with different treatments under low (5 mm (n = 5)) or high (20 mm glucose (n = 6)) conditions (a and b). The areas under the curve (AUC) of (a) and (b) were calculated and used for the analysis. Data are expressed as mean ± SEM in the AUC. Groups were compared with A23187 and presented as %. Statistical differences were processed with one-way ANOVA analysis with Tukey's post hoc test (*p < 0.05, **p < 0.01, ***p < 0.001, and ****p < 0.0001). | |
The rescuing effects of pectins on barrier disruption were very different under hyperglycemic (20 mM) conditions. Metformin had a strong protective effect, as the AUC was 92.1 ± 6.9% of its original TEER value (p < 0.0001). This effect is DM and DB dependent as both low DM and intermediate pectins with high DB (DM19HB and DM43HB) rescued the barrier disruption from A23187, which was 101.4 ± 8.2% (p < 0.0001) and 84.4 ± 5.5% (p < 0.01), respectively. This indicates that pectins with a high level or blockwise distribution of non-esterified GalA residues play a role in the rescuing effect of barrier disruption.
3.4 Pectin pretreatment protects epithelial barrier function by enhancing gene expression of claudin1 and ZO-1 in a DB and DM dependent way
As clear protective effects were observed for metformin and specific pectins on A23187-induced gut epithelial barrier disruption, we next investigated whether this could be explained by an effect on the tight junctions between the cells. We determined this for cells cultured under low (5 mM) and high (20 mM) glucose conditions. To this end, we investigated the gene expression of the tight junctions claudin1, claudin3, occludin, and ZO-1 by real-time qPCR.
Gene expression of claudin-1 was 3.15 ± 0.08 times (p < 0.0001) enhanced by the addition of A23187 in cells exposed to 5 mM glucose (Fig. 3A). However, this was not influenced by metformin or by the pectins tested at 5 mM glucose. At high (20 mM) glucose, the results were different (Fig. 3E). Claudin-1 was not enhanced by A23187 and not by pre-exposure to metformin, but pectins did enhance and regulate claudin-1. Lower DM18LB and intermediate DM49LB pectins enhanced claudin-1 expression 4.14 ± 0.22 times (p < 0.05) and 4.11 ± 0.34 times (p < 0.05), respectively. Also, higher DB pectins DM43HB and DM88HB increased claudin-1 expression 4.82 ± 0.40 times (p < 0.001) and 4.13 ± 0.17 times (p < 0.05), respectively.
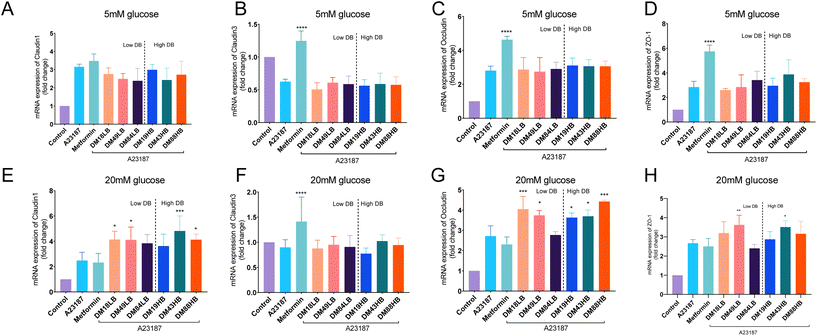 |
| Fig. 3 The mRNA expression of tight junctions in T84 cells exposed to the barrier disruptor A23187 in the presence or absence of metformin or pectins and under low (5 mm) or high (20 mm) glucose conditions. The expression of tight junctions in T84 cells is shown as a fold change. The mRNA expressions of claudin1 (a and e), claudin3 (b and f), occludin (c and g), and ZO-1 (d and h) were studied (n = 3). Data are expressed as mean ± SEM. Statistical differences were processed with one-way ANOVA analysis with Tukey's post hoc test (*p < 0.05, **p < 0.01, ***p < 0.001, and ****p < 0.0001). | |
Gene expression of claudin-3 was downregulated at 5 mM glucose 0.37 ± 0.19 times (p < 0.001) when exposed to A23187 (Fig. 3B). This could only be prevented by metformin, but not by any of the pectins tested. At 20 mM glucose (Fig. 3F), we only observed a 0.10 ± 0.05 times (p ≥ 0.05) reduction in A23187 induced reduction in claudin-3 expression, which was again not observed in pectin-exposed cells. However, metformin induced a 0.41 ± 0.15 times (p < 0.0001) increase of claudin-3 expression.
Occludin was enhanced 2.8 ± 0.12 times by A23187 (p < 0.0001) and was increased 4.6 ± 0.09 times (p < 0.0001) by metformin in cells exposed to 5 mM glucose but not by the tested pectins (Fig. 3C). At 20 mM glucose (Fig. 3G), occludin expression was increased 2.7 ± 0.29 times (p < 0.0001) by A23187. Moreover, DM18LB and DM49LB increased occludin expression by 4.1 ± 0.28 times (p < 0.001) and 3.7 ± 0.12 times (p < 0.05), respectively. However, pectins with high DB such as DM19HB, DM43HB and DM88HB enhanced occludin expression by 3.6 ± 0.10 times (p < 0.05), 3.7 ± 0.15 times (p < 0.05) and 4.4 ± 0.05 times (p < 0.001), respectively.
ZO-1 was 2.8 ± 0.21 times (p < 0.01) enhanced by the addition of A23187 and 5.7 ± 0.23 times (p < 0.0001) enhanced by the treatment of metformin in cells exposed to 5 mM glucose (Fig. 3D). However, ZO-1 was not regulated by the pectins tested at 5 mM glucose. At high (20 mM) glucose levels, occludin was 2.7 ± 0.07 times (Fig. 3H, p < 0.0001) enhanced by A23187 compared with the control group.
Occludin was not enhanced by pre-exposure to metformin, but intermediate DM pectins DM43HB and DM49LB increased ZO-1 expression by 3.5 ± 0.12 times (p < 0.05) and 3.6 ± 0.19 times (p < 0.01), respectively.
3.5 Pectins reverse the translocation of the SGLT1 induced by the barrier disruptor A23187
As metformin is known to impact glucose transporters22 and therewith the influx of glucose, we questioned whether pectins might also have such an effect in gut epithelial cells. We therefore first studied the expression of glucose transporter 2 (GLUT-2), SGTL-1, and sodium–glucose cotransporter-2 (SGLT-2) on T84 gut epithelial cells. GLUT-2 and SGLT-2 were only weakly expressed and not regulated by metformin or pectin, but SGTL-1 was. We therefore focused on the expression and dynamics of SGLT-1 in response to pectins of different structural compositions. SGLT-1 is a principal luminal glucose transporter responsible for the luminal transport of glucose to the basolateral site and bloodstream. We studied the protein expression of SGLT-1 and quantified the expression apical and basolaterally (Fig. 3). Fig. 4A and B shows the immune-fluorescence and translocation of SGLT1 of pectins with a confirmed effect on barrier function, while the others are shown in the ESI Fig. 1.† At 5 mM glucose, we found that A23187 did not enhance the apical expression of SGLT-1 but did so basolaterally (19.8 ± 1.9%, p < 0.001). This effect was partially reduced by metformin, but pectins had a stronger effect. Lower DM pectins DM18LB, DM19HB, DM43HB, and DM49LB showed a lower fluorescence intensity of SGLT1 at the basolateral side, which was 12.7 ± 1.8% (p < 0.05), 10.7 ± 2.0% (p < 0.001), 8.6 ± 1.7% (p < 0.001) and 11.5 ± 1.3% (p < 0.05), respectively. We also observed that the higher DM pectin DM88HB decreased the expression of SGLT1 at the basolateral side by 8.2 ± 1.2% (p < 0.001).
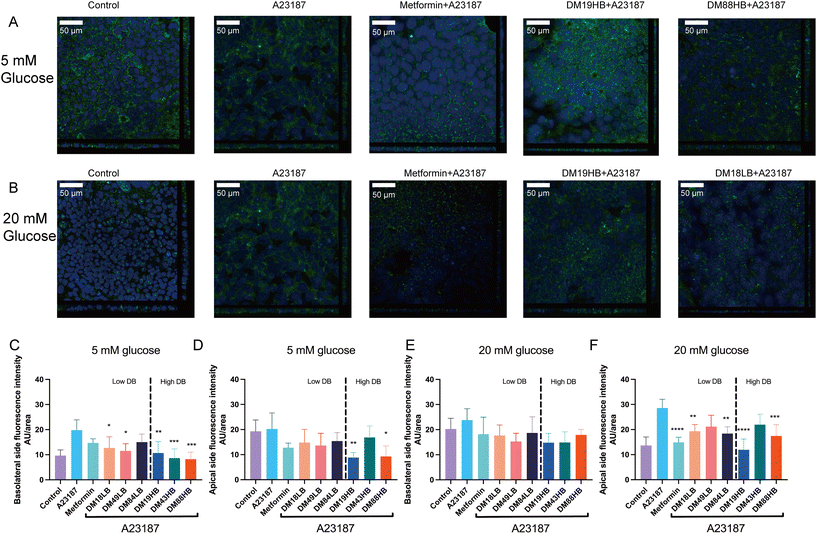 |
| Fig. 4 Confocal laser scanning microscopy and quantification of the apical or basolateral expression of the sodium–glucose co-transporter1 (SGLT1) in T84 cells with two-dimensional fluorescence detection and quantification. The SGLT1 fluorescence of T84 cells in controls, A23187, metformin, DM19HB and DM18LB under low (5 mm) or high (20 mm) glucose conditions (a and b). The fluorescence intensity of SGLT1 in T84 cells was quantified (c–f). Data are expressed as mean ± SEM of n = 3. Statistical differences were processed with one-way ANOVA analysis with Tukey's post hoc test (*p < 0.05, **p < 0.01, ***p < 0.001, and ****p < 0.0001). | |
Interestingly, at 5 mM glucose (Fig. 4A), the higher DB pectins DM19HB and DM88HB significantly lowered the apical expression of SGLT-1 with 8.9 ± 0.85% (p < 0.01) and 9.3 ± 1.9% (p < 0.05), respectively. At 20 mM glucose (Fig. 4B), we found that A23187 did not enhance the basolateral expression of SGLT1 but did so apically (28.6 ± 1.6%, p < 0.0001). This effect was reduced the most by metformin (14.9 ± 0.92%, p < 0.0001) and by low DM pectin with high DB DM19HB (12.0 ± 1.9%, p < 0.0001). Also, pectin DM18LB, DM84LB, and DM88HB induced a lowering of expression of SGLT1 on the apical side, with 19.3 ± 1.2% (p < 0.01), 18.4 ± 1.2% (p < 0.01) and 17.5 ± 2.0% (p < 0.001), respectively.
4. Discussion
Here, we show that specific types of pectins have a protective effect on gut barrier disruption. This effect is both DM and DB dependent under 20 mM glucose conditions. In comparison with the well-known barrier protective effects of metformin,23 we show that pectins are not only effective in the prevention of barrier disruption in gut epithelial cells at low (5 mM) glucose concentrations but also at higher (20 mM) glucose concentrations. As the gut epithelium is known to be the sensor for luminal glucose and as it is known that some pectins have anti-diabetogenic effects, we also studied the expression of the glucose transporters GLUT-2, SGLT-1, and SGLT-2 on T84 cells. Only SGLT-1 was dynamically expressed and, as reported before, was considered to be responsible for sensing glucose concentrations on the apical side of the lumen24 in the intestine.
In mice, it was shown that hyperglycemia leads to disruption of the intestinal epithelial barrier and enhanced transepithelial flux of harmful microbial components.8 This glucose induced barrier dysfunction is also considered to be a health issue in diabetes as it has been shown in humans that serum levels of intestinal fatty acid-binding protein, which is a biomarker of intestinal barrier dysfunction, became higher with the progression of the disease.25 Some studies associate diabetes with higher chances of the development of inflammatory bowel disease,26 which is also associated with barrier disruption.27 Frequent perturbations of barrier function led to lower numbers of secretory cells, tight junction dysfunction, and enhanced permeability.28 Many diseases such as type 1 diabetes, inflammatory bowel diseases (IBD), and acute inflammation-related diseases are associated with frequent barrier dysfunction.29 Preventing disruption of gut barrier function might therefore be an effective strategy to prevent other diseases from developing in diabetic patients, such as IBD.30
In this study, we show that DM19HB, DM18LB, DM43HB, DM49LB and DM84LB pectins at 5 mM glucose had a rescuing effect on gut barrier function while metformin was virtually ineffective at this glucose concentration. Metformin was only effective at high glucose levels. This might partly be explained by the stronger drop in TEER in response to the disruptor A23187 under 5 mM glucose than 20 mM glucose conditions. However, this effectiveness at higher glucose levels of metformin on the barrier function of cells was also observed in studies on blood–brain barrier function and artery endothelial barrier function.31,32 Some studies explain this by an effect of metformin on Jun N-terminal kinase (JNK) signaling activation via adenosine monophosphate-activated protein kinase (AMPK) α1-dependent inhibition.18 However, pectins have been shown to stabilize the cell membrane of A23187 exposed cells via a different mechanism. A23187 is a divalent ion carrier that can bind ions such as calcium and magnesium and facilitates their transport into cells.33 The influx of calcium has been related to tight junction biogenesis and the mediation of protein kinase c (PKC) signaling.34,35 As pectin is negatively charged, we hypothesize that it enables cross-links between positively charged ions such as divalent calcium ions, which prevents a too high calcium influx. This process is glucose independent and this can explain its efficacy at both low and high glucose concentrations.
The efficacy of pectins in preventing gut barrier disruption at high glucose levels was DM and DB dependent. Under high glucose conditions, we found that both low DM pectins (DM18 and DM19) and the intermediate DM pectin (DM43) with higher DB have a stronger rescuing effect than the intermediate DM pectin with a low DB or high DM (DM84 and DM88). The DM dependent effects corroborate the findings of Vogt et al.20 and Sun et al.36 that showed that pretreatment with pectins with low DM had protective effects on phorbol-12-myristate-13-acetate or bacterial infection induced disruption of gut epithelial barrier function. A possible explanation for this DM- and DB-dependent effect is that the level of methyl esters has an impact on the ζ-potential of cells.37 The low DM and intermediate DM pectins with high DB contain more blocks of negative charges, which confer them the opportunity to cross-link more divalent calcium ions than intermediate DM pectins with a low DB or high DM.38 Also, it can potentially reinforce tight junctions between epithelial cells, which can make the cells more resistant to barrier disruption.
The beneficial effects of pectins on gut barrier dysfunction were accompanied by changes in the expression of tight junctions, which is in line with previous studies.39,40 We found that A23187 as such increased the mRNA expression of claudin-1, occludin, and ZO-1. A23187 is known to be a mobile ion carrier and can induce the influx of intracellular Ca2+, which mediates PKC activity.41 Although it is still not completely understood how exactly A23187 actives PKC, we show here that the responsible processes increase claudin-1, occludin, and ZO-1 expression. Effects were different at low and high glucose levels. We found that at high glucose levels, DM43HB pectin that enhanced TEER also increased the expression of claudin1, occludin, and ZO-1. The benefit of ECIS is that we can test the impact of many molecules simultaneously, but the downside is that we need to work with lower numbers of cells, which requires us to make strategic choices. We have chosen to use the cells to do RNA isolations and to follow the dynamics of tight junction regulation on the mRNA rather than the protein level.
At high glucose concentrations, we found strong effects of both metformin and pectins on preventing gut barrier disruption. Because of these rescuing effects at high glucose concentrations only, we questioned whether this might also impact the glucose transporters in T84 gut epithelial cells. The effects were most pronounced on SGTL-1. This corroborates the findings of others42 as SGLT1 is the primary glucose transporter in the intestine, even at high glucose levels. Several studies show that most of the SGLT1 is inside the enterocyte and that SGLT1 is abundantly present on the plasma membrane at high D-glucose concentrations.43,44 We show here that upon A23187 exposure, SGLT1 is highly expressed on the basolateral side under low glucose conditions, but this changes and moves to the apical side at high glucose concentrations. This apical expression of SGLT1 was less when the gut epithelial cells were pretreated with metformin under high glucose conditions. This same effect was found for DM18LB, DM19HB, DM84LB, and DM88HB pectins. Low DM pectins induced a lower expression of SGLT1 at the apical side, similar to what we observed for metformin.45
5. Conclusions
Our study suggests that low DM pectins (DM18 and DM19) or intermediate DM pectin DM43 with high DB, can prevent barrier dysfunction in T84 gut epithelial cells under low and high glucose conditions. In contrast to the antidiabetogenic drug metformin, pectin's rescuing effects on gut barrier function are not restricted to high glucose but are also observed at lower glucose levels. Also, high DB pectins DM19HB and DM88HB have a stronger impact on the glucose transporter SGLT-1 than DM18LB and DM84LB in regulating glucose transport. This study provided novel evidence for structure dependent effects of pectin on preventing gut barrier disruption at low and high glucose levels. Our data suggest that pectin in specific chemical structures might contribute to improved barrier function in diabetic individuals.
Author contributions
X. T. and P. D. V. designed the study. X. T. performed the experiments. B. D. H., M. F., M. T. C. W. and M. B. assisted in the experiments. X. T., M. B. and P. D. V. revised and edited the manuscript. P. D. V. supervised and administered the project. X. T. and P. D. V. wrote the manuscript. All authors have revised and approved the manuscript.
Conflicts of interest
The authors declare no conflicts of interest.
Acknowledgements
X. T. was financially supported by the China Scholarship Council (CSC).
References
- M. Chandalia, A. Garg, D. Lutjohann, K. Von Bergmann, S. M. Grundy and L. J. Brinkley, Beneficial effects of high dietary fiber intake in patients with type 2-diabetes mellitus, N. Engl. J. Med., 2000, 342(19), 1392 CrossRef CAS PubMed.
- P. D. Schley and C. J. Field, The immune-enhancing effects of dietary fibres and prebiotics, Br. J. Nutr., 2002, 87, S221 CrossRef CAS PubMed.
- J. L. Slavin, Dietary fiber and body weight, Nutrition, 2005, 21(3), 411 CrossRef PubMed.
- M. O. Wellington, K. Hamonic, J. E. Krone, J. K. Htoo, A. G. Van Kessel and D. A. Columbus, Effect of dietary fiber and threonine content on intestinal barrier function in pigs challenged with either systemic E. coli lipopolysaccharide or enteric Salmonella Typhimurium, J. Anim. Sci. Biotechnol., 2020, 11(1), 1 CrossRef PubMed.
- J. R. Turner, Intestinal mucosal barrier function in health and disease, Nat. Rev. Immunol., 2009, 9(11), 799 CrossRef CAS PubMed.
- F. G. Chirdo, O. R. Millington, H. Beacock-Sharp and A. M. Mowat, Immunomodulatory dendritic cells in intestinal lamina propria, Eur. J. Immunol., 2005, 35(6), 1831 CrossRef CAS PubMed.
- D. Hollander and J. D. Kaunitz, The “leaky gut”: Tight junctions but loose associations?, Dig. Dis. Sci., 2020, 65(5), 1277 CrossRef PubMed.
- C. A. Thaiss, M. Levy, I. Grosheva, D. Zheng, E. Soffer, E. Blacher, S. Braverman, A. C. Tengeler, O. Barak, M. Elazar and R. Ben-Zeev, Hyperglycemia drives intestinal barrier dysfunction and risk for enteric infection, Science, 2018, 359(6382), 1376 CrossRef CAS PubMed.
- W. Bielka, A. Przezak and A. Pawlik, The role of the gut microbiota in the pathogenesis of diabetes, Int. J. Mol. Sci., 2022, 23(1), 480 CrossRef CAS PubMed.
- M. Beukema, M. M. Faas and P. De Vos, The effects of different dietary fiber pectin structures on the gastrointestinal immune barrier: Impact via gut microbiota and direct effects on immune cells, Exp. Mol. Med., 2020, 52, 1364 CrossRef CAS PubMed.
- A. J. Mort, F. Qiu and N. O. Maness, Determination of the pattern of methyl esterification in pectin. Distribution of contiguous nonesterified residues, Carbohydr. Res., 1993, 247, 21 CrossRef CAS PubMed.
- M. Beukema, É. Jermendi, M. A. van den Berg, M. M. Faas, H. A. Schols and P. De Vos, The impact of the level and distribution of methyl-esters of pectins on TLR2–1 dependent anti-inflammatory responses, Carbohydr. Polym., 2021, 251, 117093 CrossRef CAS PubMed.
-
K. Behall and S. Reiser, Effects of pectin on human metabolism, in Chemistry and Function of Pectins, 1986, p. 248 Search PubMed.
- J. Slavin, Fiber and prebiotics: Mechanisms and health benefits, Nutrients, 2013, 5(4), 1417 CrossRef CAS PubMed.
- R. K. Toivonen, R. Emani, E. Munukka, A. Rintala, A. Laiho, S. Pietilä, J. P. Pursiheimo, P. Soidinsalo, M. Linhala, E. Eerola and P. Huovinen, Fermentable fibres condition colon microbiota and promote diabetogenesis in NOD mice, Diabetologia, 2014, 57(10), 2183 CrossRef CAS PubMed.
- S. Hu, R. Kuwabara, M. Beukema, M. Ferrari, B. J. De Haan, M. T. Walvoort, P. De Vos and A. M. Smink, Low methyl-esterified pectin protects pancreatic β-cells against diabetes-induced oxidative and inflammatory stress via galectin-3, Carbohydr. Polym., 2020, 249, 116863 CrossRef CAS PubMed.
- J. B. Furness, L. R. Rivera, H. J. Cho, D. M. Bravo and B. Callaghan, The gut as a sensory organ, Nat. Rev. Gastroenterol. Hepatol., 2013, 10(12), 729 CrossRef CAS PubMed.
- J. Deng, L. Zeng, X. Lai, J. Li, L. Liu, Q. Lin and Y. Chen, The gut as a sensory organ. Metformin protects
against intestinal barrier dysfunction via AMPKα1-dependent inhibition of JNK signalling activation, J. Cell. Mol. Med., 2018, 22(1), 546 CrossRef CAS PubMed.
- N. M. Sahasrabudhe, M. Beukema, L. Tian, B. Troost, J. Scholte, E. Bruininx, G. Bruggeman, M. Van den Berg, A. Scheurink, H. A. Schols and M. M. Faas, Dietary fiber pectin directly blocks toll-like receptor 2–1 and prevents doxorubicin-induced ileitis, Front. Immunol., 2018, 9, 383 CrossRef PubMed.
- L. M. Vogt, N. M. Sahasrabudhe, U. Ramasamy, D. Meyer, G. Pullens, M. M. Faas, K. Venema, H. A. Schols and P. De Vos, The impact of lemon pectin characteristics on TLR activation and T84 intestinal epithelial cell barrier function, J. Funct. Foods, 2016, 22, 398 CrossRef CAS.
- N. F. Wiernsperger and C. J. Bailey, The antihyperglycaemic effect of metformin, Drugs, 1999, 58(1), 31 CrossRef CAS PubMed.
- O. Horakova, P. Kroupova, K. Bardova, J. Buresova, P. Janovska, J. Kopecky and M. Rossmeisl, Metformin acutely lowers blood glucose levels by inhibition of intestinal glucose transport, Sci. Rep., 2019, 9, 6156 CrossRef PubMed.
- A. Spruss, G. Kanuri, C. Stahl, S. C. Bischoff and I. Bergheim, Metformin protects against the development of fructose-induced steatosis in mice: role of the intestinal barrier function, Lab. Invest., 2012, 92(7), 1020 CrossRef CAS PubMed.
- N. Harada and N. Inagaki, Role of sodium–glucose transporters in glucose uptake of the intestine and kidney, J. Diabetes Invest., 2012, 3(4), 352 CrossRef CAS PubMed.
- Y. Wang, L. Ding, J. Yang, L. Liu and L. Dong, a biomarker of intestinal barrier dysfunction, increases with the progression of type 2 diabetes, PeerJ, 2021, 9, e10800 CrossRef PubMed.
- G. Fuschillo, V. Celentano, M. Rottoli, G. Sciaudone, A. G. Gravina, R. Pellegrino, R. Marfella, M. Romano, F. Selvaggi and G. Pellino, Influence of diabetes mellitus on inflammatory bowel disease course and treatment outcomes. A systematic review with meta-analysis, Dig. Liver Dis., 2023, 5, 55 Search PubMed.
- M. A. McGuckin, R. Eri, L. A. Simms, T. H. Florin and G. Radford-Smith, Intestinal barrier dysfunction in inflammatory bowel diseases, Inflammatory Bowel Dis., 2009, 15(15), 100 CrossRef PubMed.
- Á. Camilleri, K. Madsen, R. Spiller, B. G. Van Meerveld and G. N. Verne, Intestinal barrier function in health and gastrointestinal disease, Neurogastroenterol. Motil., 2012, 24(6), 503 CrossRef PubMed.
- J. König, J. Wells, P. D. Cani, C. L. García-Ródenas, T. MacDonald, A. Mercenier, J. Whyte, F. Troost and R. J. Brummer, Human intestinal barrier function in health and disease, Clin. Transl. Gastroenterol., 2016, 7(10), e196 CrossRef PubMed.
- N. Kamada, S. U. Seo, G. Y. Chen and G. Núñez, Role of the gut microbiota in immunity and inflammatory disease, Nat. Rev. Immunol., 2013, 13(5), 321 CrossRef CAS PubMed.
- F. Takata, S. Dohgu, J. Matsumoto, T. Machida, S. Kaneshima, M. Matsuo, S. Sakaguchi, Y. Takeshige, A. Yamauchi and Y. Kataoka, Metformin induces up-regulation of blood–brain barrier functions by activating AMP-activated protein kinase in rat brain microvascular endothelial cells, Biochem. Biophys. Res. Commun., 2013, 433(4), 586 CrossRef CAS PubMed.
- M. Tauseef, R. Patel, C. Yadav, M. Shahid, H. Faridi, M. R. Siddiqui, M. Newaz and Z. Ahmad, Metformin prevents high glucose induced disruption of coronary artery endothelial barrier function and accelerates wound healing via regulating junctional protein,
VE-cadherin, FASEB J., 2019, 33(S1), 512 Search PubMed.
- P. W. Reed and H. A. Lardy, A23187: A divalent cation ionophore, J. Biol. Chem., 1972, 247(21), 6970 CrossRef CAS PubMed.
- C. Fernández-Lainez, M. J. Logtenberg, X. Tang, H. A. Schols, G. López-Velázquez and P. De Vos, β(2→1) chicory and β(2→1)-β(2→6) agave fructans protect the human intestinal barrier function in vitro in a stressor-dependent fashion, Food Funct., 2022, 13(12), 6737 RSC.
- R. O. Stuart, A. Sun, M. Panichas, S. C. Hebert, B. M. Brenner and S. K. Nigam, Critical role for intracellular calcium in tight junction biogenesis, J. Cell. Physiol., 1994, 159(3), 423 CrossRef CAS PubMed.
- Y. Sun, Y. He, F. Wang, H. Zhang, P. de Vos and J. Sun, Low-methoxyl lemon pectin attenuates inflammatory responses and improves intestinal barrier integrity in caerulein-induced experimental acute pancreatitis, Mol. Nutr. Food Res., 2017, 61(4), 1600885 CrossRef PubMed.
- S. H. E. Verkempinck, C. Kyomugasho, L. Salvia-Trujillo, S. Denis, M. Bourgeois, A. M. Van Loey, M. E. Hendrickx and T. Grauwet, Emulsion stabilizing properties of citrus pectin and its interactions with conventional emulsifiers in oil-in-water emulsions, Food Hydrocolloids, 2018, 85, 144 CrossRef CAS.
- R. Kohn, Ion binding on polyuronates—Alginate and pectin, Pure Appl. Chem., 1975, 42(3), 371 CrossRef CAS.
- C. Chelakkot, J. Ghim and S. H. Ryu, Mechanisms regulating intestinal barrier integrity and its pathological implications, Exp. Mol. Med., 2018, 50(8), 1 CrossRef CAS PubMed.
- S. H. Lee, Intestinal permeability regulation by tight junction: implication on inflammatory bowel diseases, Intest. Res., 2015, 13(1), 11 CrossRef PubMed.
- Y. H. Tai, J. Flick, S. A. Levine, J. L. Madara, G. W. G. Sharp and M. Donowitz, Regulation of tight junction resistance in T84 monolayers by elevation in intracellular Ca2+: a protein kinase C effect, J. Membr. Biol., 1996, 149(1), 71 CrossRef CAS PubMed.
- P. V. Röder, K. E. Geillinger, T. S. Zietek, B. Thorens, H. Koepsell and H. Daniel, The role of SGLT1 and GLUT2 in intestinal glucose transport and sensing, PLoS One, 2014, 9(2), e89977 CrossRef PubMed.
- H. Kipp, S. Khoursandi, D. Scharlau and R. K. Kinne, More than apical: Distribution of SGLT1 in Caco-2 cells, Am. J. Physiol.: Cell Physiol., 2003, 285(4), C737 CrossRef CAS PubMed.
- P. A. Sharp, E. S. Debnam and S. K. Srai, Rapid enhancement of brush border glucose uptake after exposure of rat jejunal mucosa to glucose, Gut, 1996, 39(4), 545 CrossRef CAS PubMed.
- P. V. Bauer, F. A. Duca, T. Z. Waise, B. A. Rasmussen, M. A. Abraham, H. J. Dranse, A. Puri, C. A. O'Brien and T. K. Lam, Metformin alters upper small intestinal microbiota that impact a glucose-SGLT1-sensing glucoregulatory pathway, Cell Metab., 2018, 27(1), 101 CrossRef CAS PubMed.
|
This journal is © The Royal Society of Chemistry 2023 |
Click here to see how this site uses Cookies. View our privacy policy here.