DOI:
10.1039/D3FO00818E
(Paper)
Food Funct., 2023,
14, 6914-6928
Rebaudioside A from Stevia rebaudiana stimulates GLP-1 release by enteroendocrine cells via bitter taste signalling pathways†
Received
27th February 2023
, Accepted 29th June 2023
First published on 3rd July 2023
Abstract
Glucagon-like peptide 1 (GLP-1) is a multifaceted intestinal hormone with diverse physiological functions throughout the body. Previously, we demonstrated that the steviol glycoside rebaudioside A (rebA) from Stevia rebaudiana stimulates the release of GLP-1 from mouse intestinal organoids and pig intestinal segments. To further unravel the underlying mechanisms, we examined the involvement of sweet- and bitter taste receptors and their associated signal transduction pathways. Experiments with mouse and human intestinal enteroendocrine cell lines (STC-1 and HuTu-80, respectively) confirmed that rebA stimulates GLP-1 release in a concentration-dependent manner. Experiments with selective inhibitors of sweet signalling in both the murine as well as the human enteroendocrine cells showed that the GLP-1-induced release by rebA occurs independently of the sweet taste receptor. Functional screening of 34 murine bitter taste receptors (Tas2rs) revealed an activation response with Tas2r108, Tas2r123 and Tas2r134. Moreover, we found evidence in human HuTu-80 cells, that TAS2R4 and TRPM5 are involved in rebA-induced GLP-1 secretion, suggesting a role for bitter taste signaling in gut hormone release. Interestingly, the rebA-dependent GLP-1 release may be modulated by GABA and 6-methoxyflavanone present in the diet. Together, our findings warrant further characterization of the specific metabolic effects of rebA among the non-caloric sweeteners.
Introduction
Since the approval of steviol glycosides from the plant Stevia rebaudiana in Europe and the US, their use as non-caloric sweeteners in foods and beverages has considerably increased.1 The numerous steviol glycosides differ in sweet sensation intensity.2 Stevioside, rebaudioside A (rebA) and rebaudioside D display low sweet taste thresholds when tested by sensory panels, and demonstrate comparable relative potencies in cell-based assays functionally expressing sweet taste receptors.3 Next to their intense sweet taste, steviol glycosides such as rebA and stevioside induce a notorious bitter aftertaste.4,5
Despite their abundant use in foods and beverages, the effects of steviol glycosides on glucose homeostasis and energy intake are not completely clear, with studies reporting diverse outcomes, either beneficial or neutral.6 A randomized controlled 12-week human intervention study comparing different low-caloric sweeteners with sucrose showed a significant increase in body weight in the groups receiving sucrose and saccharin compared to rebA.7 However, the intervention did not affect postprandial blood glucose response. Other studies also found no effects of rebA and stevioside on fasting glucose and insulin levels or energy intake in type-2 diabetic (T2D) patients and healthy subjects when compared to placebo.8–10 Still, these results differ from those of other studies performed in lean and obese subjects where stevia consumption resulted in reduced postprandial blood glucose and insulin plasma concentrations in comparison to the aspartame and the sucrose conditions.11 Similar results were found in T2D subjects where 1 g of stevioside compared to placebo (maize starch), reduced post-prandial blood glucose by 18% after a standard meal in an acute, paired crossed-over study.12 In rats, Fujita et al. found no effects of stevia on glucose levels in a glucose tolerance test or on the plasma levels of incretins glucagon-like peptide-1 (GLP-1) and gastric inhibitory polypeptide (GIP),13 while others did report effects of stevioside and rebA on glucose and/or insulin in healthy and diabetic rodents.14,15 Whether steviol glycosides modulate appetite, food intake and satiety remains unclear. In humans, a rebA-based product contributed to decreased ad libitum food intake after intraduodenal infusion of a sweet-umami combination containing rebA as the sweet tastant.16 Additionally, appetite and total energy intake during a lunch decreased following consumption of a rebA containing beverage.17
After ingestion, steviol glycosides remain intact throughout the small intestine. Only upon contact with bacteria in the colon, the glycosidic bonds are hydrolyzed and free steviol is subsequently absorbed.18 Thus, presumably these stevia components interact as glycosides with G protein-coupled taste receptors (GPCRs) expressed on enteroendocrine cells (EECs) located in the small intestinal tract.19,20 Enteroendocrine cells produce and secrete hormones, such as GLP-1, peptide YY (PYY) and cholecystokinin (CCK) upon being activated by nutritional components and other compounds such as bile acids.21 The gut-derived GLP-1 is a pleiotropic hormone with numerous physiological functions, including regulating glucose homeostasis, gastric emptying,22 and appetite.23 It is released by a subpopulation of enteroendocrine L-type cells and can signal in a paracrine manner via receptors located on sensory nerve endings, as well as in an endocrine manner.24 Circulating GLP-1, of which 95% is derived from the proximal- and distal intestinal tract,22 acts as incretin by inducing glucose-dependent insulin release from the pancreas.25 Various dietary components and microbial metabolites can induce the release of GLP-1 from EECs, irrespective of their nutritional value, including molecules that induce a sweet, bitter or umami taste.26–37
Previously, we showed that rebA elicits GLP-1 and PYY release in a location-specific manner using 2D intestinal murine organoids and small intestinal-porcine tissue segments.38,39 Another study reported that rebA activated three human GPCRs in a transfected HEK (human embryonic kidney) cell-reporter assay, namely the sweet taste receptor TAS1R2/TAS1R3, as well as the two bitter taste receptors TAS2R4 and TAS2R14.3 Additionally, steviol glycosides (including rebA) were reported to potentiate TRPM5 channel activity and influence TRPM5-mediated depolarizing currents in HEK293T cells as assessed by whole-cell patch-clamp.40 TRPM5 is a Na+ transient receptor potential channel gated by intracellular calcium located at the cell membrane. Its expression is enriched on taste cells where it plays a role in taste transduction of bitter and sweet molecules.41,42 Currently, it is not known whether the sweet- or the bitter taste receptor, or/and the TRPM5 is involved in mediating the effect of steviol glycosides on intestinal hormone release. Therefore, we investigate the involvement of the intestinal sweet- and bitter taste receptors, as well as of the TRPM5 membrane channel, in rebA-induced GLP-1 release. Following up our research with mouse intestinal organoids,38 we first examined underlying mechanism(s) by rebA-elicited effects in the murine enteroendocrine STC-1 cell line. These findings have subsequently been confirmed in human enteroendocrine HuTu-80 cells where the involvement of human taste receptors and associated signal transduction pathways has been further unraveled.
Methods
Materials
The following materials were used for cell culture: Dulbecco's modified Eagle's medium (DMEM 41966029 and 42430025; Gibco, Paisley, Scotland), fetal calf serum (FCS; Lonza, Verviers SPRL, Belgium), foetal bovine serum (FBS; HyClone, Logan, Utah, USA), Penicillin–Streptomycin 10
000 U mL−1 and 10
000 μg mL−1 100×, MEM Non-essential amino acids solution 100× (NEAA), Hank's Balanced Salt Solution (HBSS) pH 7.0 to 7.4, and HEPES 1 M pH 7.4. Chemical compounds, such as rebaudioside A (≥96% purity), stevioside (≥95% purity), sucralose, saccharin, ionomycin, poly-L-lysine, phenylmethanesulphonyl fluoride (PMSF), MDL-12330A, colchicine, 4-hydroxyanisol, flufenamic acid, γ-aminobutyric acid (GABA), 2-(p-methoxyphenoxy)propionic acid (lactisole), triphenylphosphine oxide (TPPO), and neutral red (NR) dye, were purchased from Merck (Darmstadt, Germany). Gurmarin was kindly provided by Dr. Loic Briand (INRAE, Dijon, France). Acetoxymethyl(AM)-ester of Fura-2 was obtained from Life Technologies (Carlsbad, USA). 6-Methoxyflavanone (6MF) was purchased from ABCR GmbH (Karlsruhe, Germany). Water-soluble probenecid and TRIzol™ Reagent were acquired from Invitrogen (Thermo Fisher Scientific, Waltham, Massachusetts, USA). RNeasy mini kit and on column DNase treatment used are from Qiagen (Venlo, The Netherlands). LDH Cytotoxicity Detection Kit and an XTT Cell Proliferation Kit II are from Roche Applied Science (Almere, The Netherlands). GLP-1 kits active (EGLP-35K) and total (EZGLP1T-36K) were from Merck (Darmstadt, Germany), and CCK-8 RIA kits (EURIA-CCK, RB302) from Euro-diagnostica, (Malmö, Sweden).
Cell culture
The STC-1 (ATCC, LGC Standards, Teddington, UK) is an enteroendocrine cell line derived from a double-transgenic mouse (small intestine). STC-1 cells were cultured in DMEM containing 10% FCS, at 37 °C, 5% CO2 and 90–95% humidity. The cells were passaged when reaching 80% confluence. Passage numbers between 25–50 were used. Human duodenal enteroendocrine HuTu-80 cells (ATCC® HTB-40, LGC Standards, Teddington, UK) were maintained in DMEM (without pyruvate) supplemented with 10% FBS, 1% NEAA, and Penicillin–Streptomycin (100 U mL−1–100 μg mL−1), at 37 °C, 5% CO2 and 90–95% humidity. Passage numbers between 10–22 were used.
Preparation of the stimuli
The sweet- and bitter compounds rebaudioside A, stevioside, sucralose, saccharin, colchicine, 4-hydroxyanisol, and flufenamic acid were all dissolved in assay buffer (see "secretion studies") at room temperature (RT), in a concentration 10–103 times higher than the final concentrations, by mixing and vortexing briefly. Stock solutions obtained in this way were all homogenous and clear and then subjected to filter-sterilization (0.4 μm pore size). Further dilution steps (in assay buffer) to reach final concentrations for stimulation were performed under sterile conditions. Final dilutions were prewarmed at 37 °C for 10 min before being supplied to the cells.
Secretion studies
STC-1 cells were seeded at 2 × 105 cells per mL and grown in 24-well plates (500 μL per well) for 48 hours in culture media, until reaching 80% confluence. Next, medium was removed and cells were rinsed with HBSS containing 10 mM HEPES (assay buffer). Subsequently, cells were incubated in assay buffer alone or supplemented with rebA, stevioside or sucralose at 37 °C, 5% CO2 and 90–95% humidity. After 2 hours of incubation, assay buffers were removed, mixed with PMSF (final concentration 100 μM), centrifuged to remove cell debris, and stored at −20 °C for analysis. The cAMP antagonist MDL-12330A was used at 10 μM and added without preincubation. STC-1 cells were pre-incubated for 30 min with/without various concentrations of purified recombinant gurmarin (half-maximal inhibitory concentration of 0.030 μg mL−1),43 and subsequently incubated with/without rebA with gurmarin for 2 hours.
HuTu-80 cells were seeded at a concentration of 2.5 × 105 cells per mL (160 μL per well) and grown in 96-well plates for 48 hours till they reached 80% confluence. Cells were washed twice with HBSS and subsequently incubated for 2 hours with sweet compounds (rebA, sucrose, sucralose and saccharin) or the bitter compounds (colchicine, 4-hydroxyanisol and flufenamic acid), or ionomycin in HBSS supplemented with 10 mM HEPES (assay buffer) at 37 °C, 5% CO2 and 90–95% humidity. The pre-incubation times with inhibitors proceeded as detailed: GABA, 6MF (or a combination thereof), and lactisole (pH 7.7) were added 40 min in advance; TPPO was added 5 min before. Solvent control e.g. DMSO never exceeded 0.1% during stimulation. All pre-incubations occurred at 37 °C, 5% CO2 and 90–95% humidity, and non-harmful concentrations of single and combined chemicals were employed. Samples were collected on ice, combined with PMSF (final concentration of 0.625 mM), centrifuged to remove cell debris, and stored (−80 °C) for GLP-1 analysis.
Cytotoxicity
Effects of the test compounds on the viability of the STC-1 cells were assessed using the LDH cytotoxicity detection method, according to the manufacturer's instructions. Briefly, cells were first incubated with the test compounds for 2 hours. Thereafter, the levels of the enzyme lactate dehydrogenase (LDH) were measured in supernatants to assess cell membrane damage and cell death. Triton 1% was used as a control of total cell lysis. For HuTu-80 cells, a Neutral Red (NR) test was performed after each stimulation. NR test relies on the colorimetric quantification of the lysosomes-incorporated dye of viable cells, whereas non-viable cells cannot actively transport it.44 For this, a precipitate-free solution of 40 μg mL−1 of NR in assay buffer was incubated with the cells for 2 hours. After dye extraction with a solution of 0.05 M NaH2PO4/96% ethanol (1
:
1), absorbance was measured at 540 nm. False positive detection of GLP-1 release due to cell membrane damage was discarded by LDH test. For cytotoxicity data see ESI S1.†
Gut hormone measurements
GLP-1 active and GLP-1 total levels were determined using ELISA kits EGLP-35K and EZGLP1T-36K, respectively, according to the manufacturer's instructions. GLP-1 active (7–36 amide and 7–37 active forms) was determined by employing STC-1 cell samples, and GLP-1 total was determined in HuTu-80 cell samples. GLP-1 total ELISA detects active and inactive GLP-1 in the amide form. Concentrations of CCK-8 (CCK 26-33) were analyzed with a validated commercial RIA kit (EURIA-CCK). Radioactivity of [125I]-CCK-8 was measured by liquid scintillation.
STC-1 intracellular calcium ion measurements
First, STC-1 cells stably expressing the Cameleon YC3.6 calcium reporter protein45 were cultivated in DMEM supplement with 10% FBS, 1× Pen/Strep and 1× NEAA in the presence of the selection marker geneticin (50 μg mL−1). Cells were employed to examine sweet receptor activity by cytoplasmic calcium ion changes upon sucrose stimulus with/without sweet receptor blocker (gurmarin). For the experiment cells were seeded on 15 × 45 mm2 borosilicate cover slides (coated with 100 μg mL−1 poly-L-lysine) at a density of 3 × 105 cells per mL in DMEM containing 5.5 mM glucose, and grown at 37 °C and 5% CO2 for 24 h. Subsequently, the slides were mounted in a flow cell (Micronit Microfluidics B.V., Enschede, The Netherlands),45 and cells were imaged with a Leica M205 FA Fluorescence Stereo Microscope equipped with CFP and FRET excitation/emission filters (excitation CFP 436/20 nm, emission CFP 480/40 nm, FRET emission YFP 535/30 nm), and images were analyzed using ImageJ software (open source, NIH). Slides with a constant flow of 100 μL min−1 received injected stimuli by a 50 μL sample loop.
In addition, STC-1 cells were plated onto clear bottom black 96-well plates (BD Biosciences, Erembodegem, Belgium) at a density of 4 × 105 cells per mL (100 μL). The next day cells were washed with HBSS and loaded with 5 μM Fura-2 for 30 min at 37 °C. After a subsequent wash with HBSS, the plate was incubated in the BD pathway™ 855 bioimager (BD Biosciences, Erembodegem, Belgium), and kept at 37 °C and 5% CO2. Measurements were performed with excitation at 340 and 380 nm for ratiometric analysis, and images were acquired using 20× magnification. Cells were automatically identified as regions of interest and changes in 340/380 fluorescence emission ratio for these regions were analyzed using BD Attovision software (BD Biosciences, Erembodegem, Belgium). Stimuli (rebA or control buffer) were applied in a fully automated manner, adding 20 μL of 10×-concentrated solution.
Functional screening of mouse bitter response to rebaudioside A: HEK293T calcium ions flux
Functional expression of mouse Tas2rs was done as before.36,46 Briefly, HEK293T cells stably expressing the chimeric G protein Gα16gust44 were seeded onto 96-well plates and transiently transfected with cDNA-constructs of 34 out of the 35 mouse Tas2rs46 using Lipofectamine 2000. After 24 h cells were loaded with the calcium sensitive dye Fluo4-am in the presence of probenecid, washed with assay buffer (130 mM NaCl, 5 mM KCl, 2 mM CaCl2, 10 mM glucose, 10 mM HEPES; pH 7.4) and placed in a fluorometric imaging plate reader (FLIPRtetra). The changes in fluorescence after automated application of 1 and 3 mM rebA were monitored. For negative controls, empty vector transfected cells were treated identically. Receptors exhibiting rebA responses were selected for the establishment of dose–response relationships using the same procedures. Graphs showing the dose-dependent curves were obtained with SigmaPlot software (Inpixon Inc., USA).
Microarray analysis: gene expression levels
STC-1 cells were incubated for 2 hours without (control) or with 1 mM rebA, in three biological repetitions. In brief, RNA isolation followed by microarray hybridization was performed according to the manufacturer's instructions. First, TRIzol and RNeasy mini kit with on-column DNase treatment were employed. Thereafter, the hybridization, washing and scanning steps of the Affymetrix GeneChip Mouse Gene 1.1 ST array employed, were carried out according to the Affymetrix protocol. Analysis of the arrays included normalizing using the Robust Multi-array Average method.47,48 Mouse Tas1r2, Tas1r3, Gnat3, Tas2r108, Tas2r123 and Tas2r134 genes (gene IDs 83770, 83771, 242851, 57253, 353167, and 387511, respectively) were reviewed and normalized average gene intensities (unlogged scale) were represented in bar plots (arbitrary units).
For HuTu-80 cells, the expression levels of the human genes TAS1R2, TAS1R3, TAS2R4, TAS2R14, GNAT3 and TRPM5 (gene IDs 80834, 83756, 50832, 50840, 346562, and 29850, respectively) were derived from the publicly available microarray dataset GSE36139,49 from the GEO repository (Gene Expression Omnibus, NCBI). Briefly, the mRNA expression analysis has been obtained by employing the microarray platform GPL15308, from a study on unstimulated cells cultured according to the vendors’ instructions.49 Unfortunately, the Entrez gene ID 83756 for the TAS1R3 gene of one monomer of the sweet taste receptor is not included in this particular microarray type (GPL15308), therefore its expression level could not be studied.
Statistical analysis
Normalized data of hormone detection in cell supernatants were plotted in GraphPad Prism 9.3.0 software for Windows (San Diego, California USA), and statistical analyses for dose–response curves were conducted using one-way ANOVA with Dunnett's post hoc test for multiple comparisons against the control. Blocking experiments were analyzed by one-way ANOVA and Bonferroni's post hoc test comparing selected pairs. Uncorrected Fisher's LSD test was employed for multiple pairs comparisons (against the control). Confidence intervals and p-values for each comparison are given in the ESI (S2).† For every data set, normality of residuals was checked by Anderson-Darling and/or D'Agostino Pearson tests, and homogeneity of variance by QQ plot. P values <0.05 were considered statistically significant, with 95% confidence interval.
Results
Rebaudioside A and stevioside induce GLP-1 and CCK secretion from STC-1 cells
Dose–response relationships for GLP-1 and CCK release were assessed by stimulating STC-1 cells with rebA, stevioside and/or sucralose (Fig. 1A–C). The low-caloric sweeteners rebA and stevioside elicited a significant dose-dependent GLP-1 release (Fig. 1A and B) compared to the control. Specifically, 0.5 and 1 mM rebA evoked a 1.9 ± 0.4 and 2.3 ± 0.2-fold increase, respectively, while 5 mM stevioside induced a 3.4 ± 0.5-fold rise in GLP-1 release. By contrast, sucralose did not induce GLP-1 secretion within a 0.001 to 10 mM range (Fig. 1C). Furthermore, only rebA elicited, in a concentration-dependent manner, CCK release (Fig. 1D). Interestingly, a significant increase of CCK release was already observed at a 5-fold lower concentration (0.1 mM) compared to the rebA concentration needed to induce GLP-1 release.
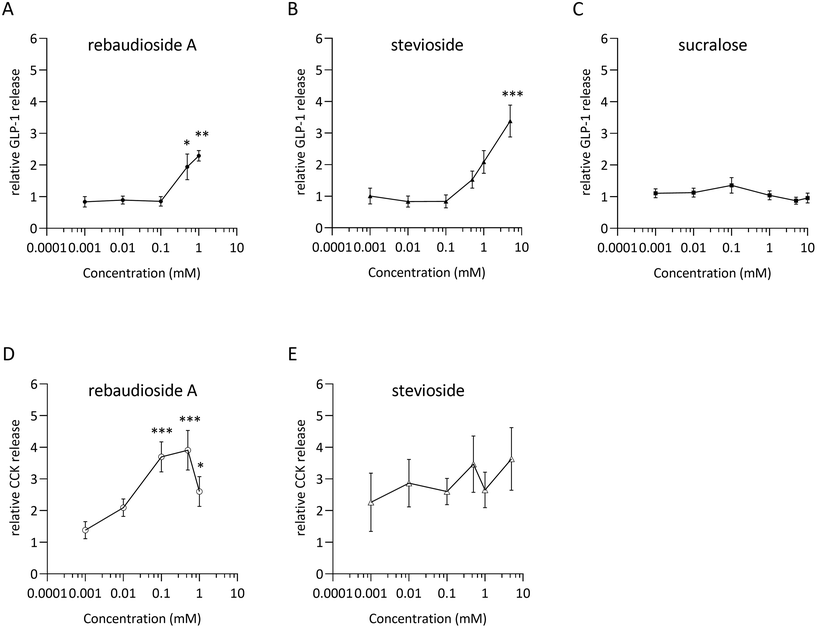 |
| Fig. 1 Hormone secretion by STC-1 cells in response to sweeteners. GLP-1 (active) release after 2-hours of incubation with increasing concentrations of (A) rebaudioside A, (B) stevioside, and (C) sucralose. CCK hormonal release after 2-hours incubation with (D) rebaudioside A, and (E) stevioside. Concentrations tested were 0.001, 0.01, 0.1, 0.5, and 1 mM of rebA; 0.001, 0.01, 0.1, 0.5, 1 and 5 mM of stevioside; and 0.001, 0.01, 0.1, 1, 5 and 10 mM of sucralose. Relative values indicate hormone release normalized by the basal release without sweetener (assay buffer). Values are means of separate biological experiments performed on different days, ±SEM: n = 4 for rebA, n = 3 for stevioside and n = 7 for sucralose. *p < 0.05, **p < 0.01, ***p < 0.001 (Dunnett's multiple comparisons test). | |
The Tas1r2/Tas1r3 antagonist gurmarin does not block rebA-induced GLP-1 release in murine STC-1 cells
We aimed to investigate whether the sweet taste receptor Tas1r2/Tas1r3 is involved in the rebA-induced effect on GLP-1 release. Therefore, first the expression levels of the mouse Tas1r2, Tas1r3, and Gnat3 (α-gustducin) genes were determined by microarray analysis of STC-1-mRNA. The two monomers and the G-protein subunit α-gustducin were found expressed and gene expression levels did not change by a 2 hours exposure to 1 mM rebA (Fig. 2A). Notably, Tas1r3 transcripts were expressed at a higher level than Tas1r2 and Gnat3 transcripts.
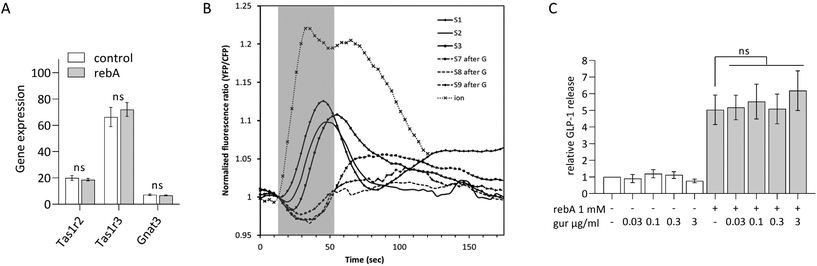 |
| Fig. 2 Role of the murine sweet taste receptor Tas1r2/Tas1r3 in rebA-induced GLP-1 response. (A) Gene expression levels (Robust multi-array Average normalized intensity) of STC-1 cells assessed by microarray analysis, after 2 hours of incubation with/without rebA (1 mM), of the murine Tas1r2, Tas1r3 and of α-Gustducin (Gnat3). Values are means ± SEM, n = 3. ns non-significant (Limma regularised paired t-test (control versus rebA)). (B) Superimposed response chart of the effect of the murine Tas1r2/Tas1r3 blocker gurmarin on sucrose-induced cytoplasmic Ca2+ levels in STC-1 cells stably expressing the Cameleon YC3.6 calcium reporter protein. Cells were exposed to 100 mM sucrose (S1–S9) before (S1–S3) and after gurmarin injections (S7–S9) of 0.3 μM gurmarin alone (S4–S6, see ESI Fig. S3†) and co-administered with 100 mM sucrose (S7–S9); find injections series in ESI Fig. S2.† The ionomycin (ion) injection (10 μM) works as a positive control for the calcium reporter. Injections at t = 0 reached cells approximately 16.25 seconds later (normalization point = 1 fluorescence ratio, greyed time zone). Shown are the global averages of all imaged cells (1315 ROIs). (C) GLP-1 release after 2 hours of incubation of STC-1 cells with rebA (1 mM) combined with a concentration series of gurmarin, and the control series. The cells were pre-incubated with the blocker for 30 min. Values represent (active) GLP-1 release relative to the basal level (control). Values are means ± SEM, n = 3. ns non-significant (Bonferroni's multiple comparisons test). | |
Next, we confirmed the ability of gurmarin to block Tas1r2/Tas1r3 in our STC-1 cell model. STC-1 cells stably expressing the Cameleon YC3.6 calcium reporter protein responded to 100 mM sucrose by showing a significant [Ca2+]i signal (Fig. 2B), which was strongly inhibited by gurmarin. Finally, a concentration series ranging from 0.03 to 3 mM gurmarin was combined with 1 mM rebA to assess the involvement of Tas1r2/Tas1r3 in rebA-elicited GLP-1 release. At none of the concentrations tested, gurmarin inhibited the rebA-induced GLP-1 release (Fig. 2C), indicating no involvement of the sweet taste receptor.
Rebaudioside A activates Tas2r108, Tas2r123 and Tas2r134 mouse bitter taste receptors
To elucidate if rebA-mediated effects on GLP-1 might involve the bitter taste signalling pathway, we first aimed to identify those Tas2rs that potentially respond to rebA. To this end, we screened HEK293T-Gα16gust44 cells transfected with 34 different mouse Tas2r-constructs46 by calcium mobilization assays. Of these receptors tested, only three, Tas2r108, Tas2r123, and Tas2r134 responded (Fig. 3A) to rebA at the used concentrations of 1 and 3 mM. These rebA-responsive receptors were subsequently stimulated with a range of rebA concentrations to obtain dose–response relationships (Fig. 3B). Whereas the highest concentration of rebA (3 mM) that could be tested without resulting in receptor-independent artefacts, elicited similar responses for all three receptors, the Tas2r123 showed higher responses at lower concentrations compared to the other two receptors, indicating higher sensitivity of this receptor. Separately, microarray analysis of STC-1 cells confirmed the expression of Tas2r108, Tas2r123 and Tas2r134 (Fig. 3C). Withal, mRNA expression levels of Tas2r108 were relatively higher than those of Tas2r123 and Tas2r134 in our murine model.
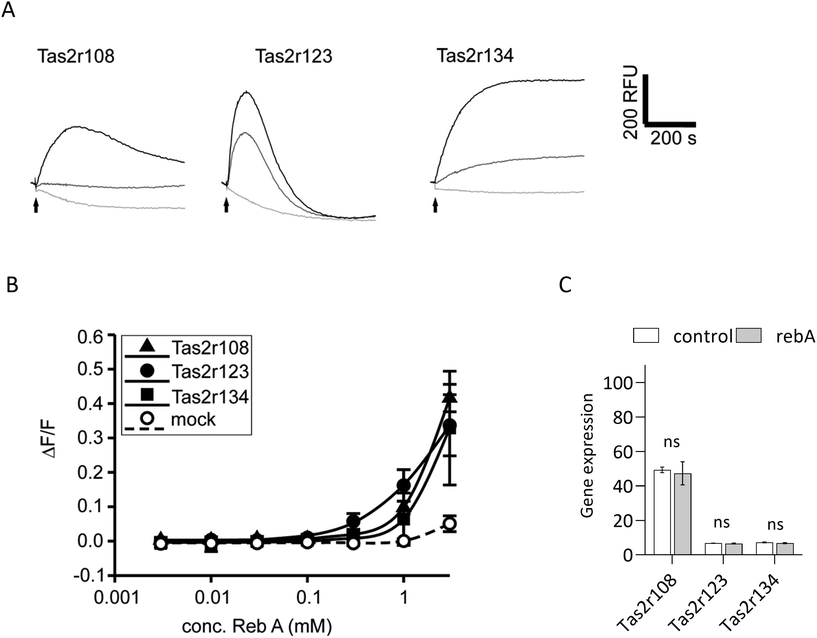 |
| Fig. 3 Rebaudioside A activates the 3 mouse bitter taste receptors Tas2r108, Tas2r123, and Tas2r134. (A) Fluorescence traces of Tas2r108, Tas2r123, and Tas2r134 transfected HEK 293T-Gα16gust44 cells stimulated with 3 mM (black traces), 1 mM (dark gray traces), or control medium (light gray traces). Substance application is indicated by arrows. (B) Dose–response relationships of Tas2r108, Tas2r123, and Tas2r134 transfected HEK 293T-Gα16gust44 cells stimulated with increasing concentrations of rebA. The relative changes of fluorescence (ΔF/F) are indicated on the y-axis, the logarithmically scaled x-axis shows the applied rebA concentration in mM. (C) Gene expression levels of Tas2r108, Tas2r123 and Tas2r134 in STC-1 cells assessed by mRNA isolation and microarray analysis after incubation with assay buffer (control, white bar) or 1 mM rebA for 2 hours (grey bar). Values are the means ± SEM (n = 3), ns non-significant (Limma regularised paired t-test (control versus rebA)). | |
GLP-1 release induced by rebA involves cAMP signalling
To investigate intracellular mechanisms of rebA-induced gut hormone release, the involvement of possible second messengers was investigated by employing STC-1 cells. To this end, cytosolic calcium ion fluctuations were measured by an intracellular [Ca2+] indicator. The calcium ionophore ionomycin, used as a control, effectively increased the [Ca2+]i levels. However, upon stimulation with rebA (2.5 mM) no rise in [Ca2+]i was observed (Fig. 4A). Subsequently, we investigated the role of cAMP using MDL-12330A, a specific inhibitor of adenylate cyclase. Combining MDL-12330A with 1 mM rebA resulted in a 77.1% reduction in GLP-1 release compared to rebA treatment alone (Fig. 4B), providing evidence that cAMP is involved in the cell signalling pathway leading to rebA-induced GLP-1 release.
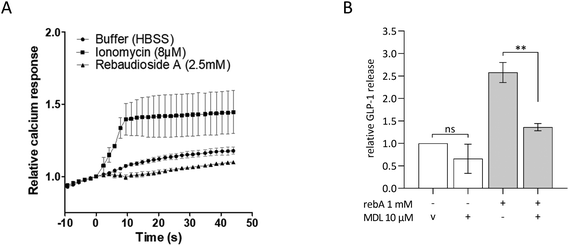 |
| Fig. 4 Role of intracellular signalling in GLP-1 response to rebA in STC-1 cells. (A) Intracellular [Ca2+] changes in response to buffer, ionomycin and rebA were measured with Fura-2. Values represent the average 340/380 nm ratio of about 40–60 regions of interest, normalized to the time of injection (t = 0). Values are expressed as means ± SEM, n = 3. (B) GLP-1 release after a 2-hours incubation with assay buffer supplemented with rebA and/or adenylate cyclase inhibitor MDL-12330A. Values are means ± SEM, n = 3. ns non-significant, v vehicle, **p < 0.01 (Bonferroni's multiple comparisons test of selected pairs). | |
Rebaudioside A induces higher GLP-1 release than other sweet compounds in HuTu-80 EECs and rebA's secretion is not blocked by lactisol
As we considered a human approach to be of preeminent value, the human duodenum-derived enteroendocrine cell line HuTu-80 was explored for its gut hormonal response to rebA and the outcomes were compared with the findings in murine STC-1 cells. The results in STC-1 EECs suggest that the murine sweet receptor is not involved in the rebA-elicited GLP-1 release while pointing towards a possible role of bitter signalling pathways. Therefore, we subsequently used a pharmacological approach to study the potential involvement of the bitter taste receptor cascade, thereby relying on available inhibitors of human TAS2Rs.
First, these EECs were tested for their ability to respond to rebA by showing GLP-1 release in a similar way as observed with STC-1 cells. To this end, HuTu-80 cells were stimulated with a concentration series of rebA and other sweet compounds. As shown in Fig. 5A, a concentration series of 250 μM rebA and higher elicited a significant dose-dependent release of GLP-1. Additionally, we studied the effects of sucrose and the non- (low-) caloric sweeteners saccharin and sucralose (Fig. 5B and C). At the concentrations tested, saccharin did not elicit any GLP-1 secretion, whereas 80 mM sucrose induced GLP-1 release significantly. Sucralose at 60 μM generated a small but significant response, comparable to the response to sucrose (Fig. 5C).
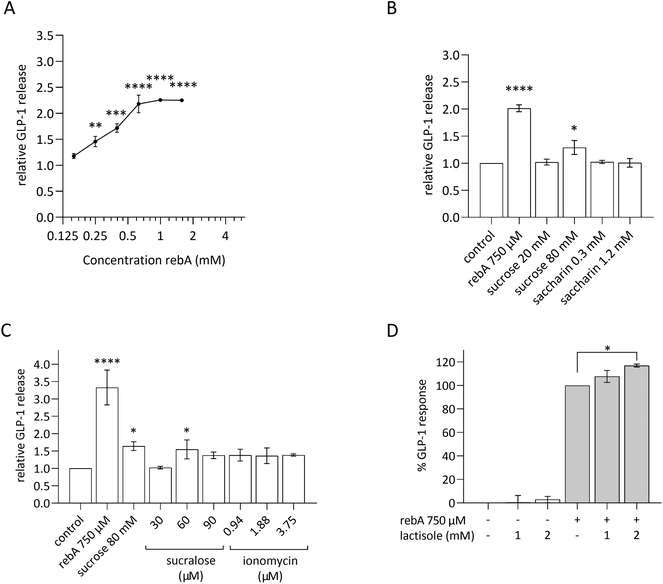 |
| Fig. 5 Effects of rebA on GLP-1 response in HuTu-80 EECs and modulation by sweeteners, lactisole and ionomycin. (A) GLP-1 release after 2 hours of incubation with increasing concentrations of rebA. (B) GLP-1 release upon exposure to sucrose and saccharine, compared to 750 μM rebA. (C) Effects of a concentration range of sucralose and ionomycin on GLP-1 release. (D) GLP-1 release after 2 hours of incubation with 750 μM rebA combined with the sweet taste receptor blocker lactisole (1 and 2 mM) and the control series. The cells were pre-incubated with the inhibitor for 40 min. Values are means ± SEM, n = 3; in (A–C) values are relative to the basal GLP-1 release in assay buffer (set at 1), and in (D) basal level of the assay buffer (control) is set at zero. *p < 0.05, **p < 0.01, ***p < 0.001, ****p < 0.0001 ((A) Dunnett's multiple comparisons test against the control, (B and C) Uncorrected Fisher's LSD test comparing mean of each bar with the mean of the control, and (D) Bonferroni's multiple comparisons test for selected pairs). | |
In addition, as calcium is a known second messenger in TAS1R2/TAS1R3 signalling, we explored GLP-1 release when HuTu-80 cells were treated with ionomycin. This membrane-permeable calcium ionophore triggers cytoplasmic Ca2+ influx.50 Ionomycin did not generate a GLP-1 release. Of note, cell viability assays showed that sucralose and ionomycin became cytotoxic at higher concentrations (120 μM and 7.5 μM, respectively) (ESI S1D and S1E, F†).
The involvement of the TAS1R2/TAS1R3 in rebA-induced GLP-1 secretion was tested in HuTu-80 cells by using lactisole, a selective antagonist of the human sweet taste receptor.51,52Fig. 5D shows the absence of an inhibitory effect with two concentrations of lactisole (1 and 2 mM). Notably, lactisole caused a small GLP-1 increase at a concentration of 2 mM. Altogether, also in HuTu-80 cells, our results point to the absence of involvement of TAS1R2/TAS1R3 (sweet) receptors in mediating the effect of rebA on GLP-1 release by EECs.
Involvement of human bitter receptor TAS2R4 in rebA-induced GLP-1 response
Next, we explored a possible role for bitter taste receptors in rebA-induced effects on GLP-1 in HuTu-80 cells. TAS2R4, the human ortholog of the mouse Tas2r108, and TAS2R14 are known to be activated by rebA in a HEK cell line overexpressing either TAS2R4 or TAS2R14.3 The involvement of these two TAS2Rs in HuTu-80 cells was investigated using known blockers: GABA for TAS2R4,53 and 6-MF for TAS2R14;54 and known bitter ligands: colchicine (TAS2R4), 4-hydroxyanisol (TAS2R14), and flufenamic acid (TAS2R14).55,56 However, the agonists of TAS2R4 or TAS2R14 did not induce GLP-1 release from HuTu-80 cells in our hands (Fig. 6A). Next, our pharmacological approach revealed that co-incubation with 1 μM GABA significantly reduced the rebA-induced GLP-1 release by 21% (Fig. 6B), while none of the 6-MF concentrations tested blocked the effect of 750 μM rebA. Instead, 6-MF caused an increase of the rebA-induced GLP-1 secretion of up to 57% and 51% with 50 nM and 100 nM, respectively (Fig. 6C).
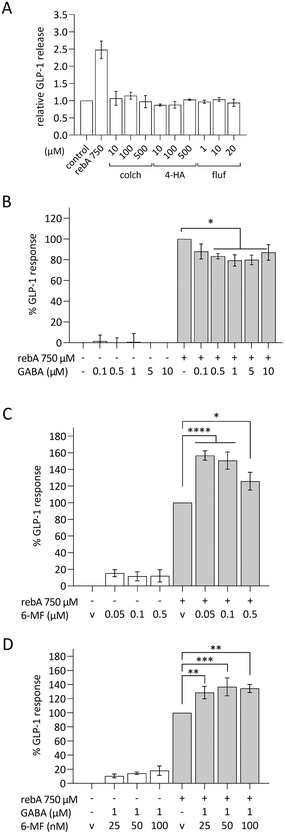 |
| Fig. 6 Modulation of rebA-induced GLP-1 response. (A) GLP-1 release in HuTu-80 by the TAS2R4 and TAS2R14 ligands colchicine (TAS2R4 semi-specific agonist), 4-hydroxyanisol (TAS2R14-specific agonist), and flufenamic acid (potent TAS2R14-specific agonist) in HuTu-80 cells (indicated in the plot as colch, 4-HA and fluf, respectively) compared to rebA (750 μM). Values are means ± SEM, n = 3, relative to the basal GLP-1 release in assay buffer. Modulation of GLP-1 response to rebA by (B) TAS2R4-inhibitor GABA, and by (C) the TAS2R14-inhibitor 6-MF, after pre-incubation with the inhibitor and 2-hours stimulation with 750 μM rebA with/without inhibitor. (D) Combined experiment with both TAS2R4/R14 bitter inhibitors consisting of a set concentration of 1 μM GABA plus increasing concentrations of 6-MF. White bars show the control series with the corresponding mixtures of inhibitors and the solvent control (v). (B), (C) and (D) values are means ± SEM, n = 3, relative to the GLP-1 response to rebA (GLP-1 release – basal release); v (C and D) = 0.1% DMSO. *p < 0.05, **p < 0.01, ***p < 0.001, ****p < 0.0001 (one-way ANOVA followed by uncorrected Fisher's LSD for selected pairs). | |
Then, it was assessed whether the combination of GABA and 6-MF could affect rebA-mediated GLP-1 release. As shown in Fig. 6D, preincubation with a combination of both 1 μM GABA and 25 nM, 50 nM or 100 nM 6-MF resulted in a significant increase of rebA-induced GLP-1 release at 25, 50 and 100 nM. However, in the presence of GABA, rebA-induced GLP-1 secretion was found to be lower than with 6-MF alone (50% less enhancement than with 50 nM 6-MF). Combined incubation with both GABA and 6-MF did not affect GLP-1 secretion in the control, and had no effects on cell viability (ESI Fig. S1L†). Altogether, these results point to a partial involvement of TAS2R4 in the GLP-1 secretion induced by rebA.
TRPM5 forms part of the mechanism underlying rebA-induced GLP-1 release
The intracellular calcium-activated membrane receptor TRPM5 is known to perform an important role in canonical taste signalling.57–59 We therefore investigated whether TRPM5 would contribute to GLP-1 secretion by HuTu-80 cells. The expression of TRPM5, determined by microarray data analysis in HuTu-80 cells was low, but within the levels of the previously observed taste signalling molecules and receptors (ESI S4†). By the use of TPPO, a selective inhibitor of TRPM5,60 the GLP-1 release in HuTu-80's was significantly reduced (27%) (Fig. 7). TPPO concentrations did not affect the control series, nor cell viability (ESI Fig. S1M†). By exploring this, we showed that the TRPM5 ion channel is (partly) involved in the release of GLP-1 elicited by rebA.
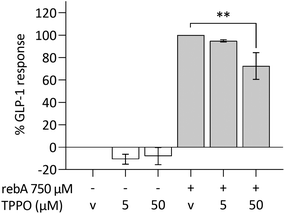 |
| Fig. 7 Effects of the TRPM5-inhibitor TPPO on rebA-induced GLP-1 response in HuTu-80 cells. Modulation of the GLP-1 response to rebA by 5 and 50 μM of the TRPM5 inhibitor TPPO, after 5 min pre-incubation with the inhibitor and 2 hours of stimulation with 750 μM rebA with inhibitor or vehicle (v). Values are means ± SEM, n = 3, relative to the GLP-1 response to rebA (GLP-1 release – basal release); v = 0.05% DMSO, **p < 0.01 (one-way ANOVA and uncorrected Fisher's LSD for comparison of selected pairs). | |
Discussion
Low-calorie (non-nutritive) sweeteners are a structurally diverse group of substances that stimulate sweet receptors, and their biological heterogeneity should be considered in view of their metabolic and off-target effects. Previously, we reported that rebA stimulates GLP-1 and PYY secretion in 2D mice organoids38 and porcine gut explants.39 Our present study confirms these findings in human and murine EECs and demonstrates that the bitter taste receptor signalling pathway plays a role in the rebA-induced GLP-1 release. Our data further indicate that rebA does not exert this effect via the sweet taste receptor (TAS1R2/TAS1R3) but instead interacts with bitter signalling pathways in EECs by acting on TAS2R4 and possibly also via the TRPM5 channel.
Steviol glycosides are known to be potent ligands for the human sweet taste receptor,3 and in mice, Tas1r2/Tas1r3 is essential for stevia tasting.61 Beyond the taste bud cells, the sweet taste receptor is expressed in many extra-oral tissues including the pancreas and intestines,62 where it is co-expressed with GLP-1 and GIP positive cells.26,63 Cell-based studies provide evidence for the involvement of the sweet taste receptor in mediating gut hormone release. For example, GLP-1 secretion in response to sucralose and glucose in colon-derived human NCI-H716 cells was reduced by the TAS1R2/TAS1R3 inhibitor lactisole and this process was dependent on signalling via α-gustducin G protein.26 Similarly, in murine GLUTag cells, sucralose-stimulated GLP-1 and GIP release was inhibited by gurmarin.64 Also, high glucose-stimulated GLP-1 secretion in STC-1 cells has been reported to be attenuated by downregulation of Tas1r2/Tas1r3 signalling.65 However, there are also studies in which no direct involvement was observed. For example, studies showed that glucose-induced GLP-1 release was mediated primarily via SGLT-1-dependent transport and closure of ATP-dependent potassium channels, and secondary by GLUT2 transport.64,66 Additionally, in small intestinal primary cells or perfused tissue, no effect of sucralose on GLP-1 release was observed.29,67–69 In our work, we found a small increase in the release of GLP-1 with sucralose (60 μM) and sucrose (80 mM) in HuTu-80 cells, but no effect of saccharin. The lack of a GLP-1 response to sucralose in STC-1 cells points to differences between mouse and human, or to differences in sensitivity between cell models.
At first, we investigated whether the sweet taste receptor is involved in rebA-induced hormone secretion. Our study showed that gurmarin, a specific Tas1r2/Tas1r3 antagonist43 inhibited intracellular [Ca2+] in response to sucrose in STC-1 cells, but did not attenuate rebA-elicited GLP-1 release. Similarly, lactisole, a specific TAS1R2/TAS1R3 antagonist,52 did not reduce GLP-1 release in HuTu-80 cells exposed to rebA. The action of lactisole relies on its interaction with the transmembrane domains of TAS1R3 receptor.51 Although it did not block the effect of rebA in HuTu-80, earlier studies did show lactisole mediated inhibition of GLP-1 secretion stimulated by glucose or by non-nutritive sweeteners, though those were based on other cell models such as the human NCI-H716- or murine GLUTag cells.26,64 Therefore, the induction of GLP-1 secretion by sweet-tasting compounds may involve multiple mechanisms, operating either dependently or independently of the luminally expressed sweet taste receptor.
We also provide evidence that the rebA-induced GLP-1 release is dependent on cAMP signalling. MDL-12330A, has previously been shown to reduce cAMP production and block cAMP-dependent GLP-1 release in STC-1 cells.70–72 Also in our hands, it reduced GLP-1 secretion, elicited by rebA. Cyclic AMP has been shown to trigger GLP-1 release in colonic murine GLUTag cells,73 suggesting that cellular cAMP plays a role in the signalling of rebA-mediated effects on GLP-1 secretion. We did not observe changes in [Ca2+]i levels upon stimulation of rebA in STC-1. The absence of a rise in [Ca2+]i in combination with increased intracellular cAMP levels was reported previously for different low-calorie sweeteners in intestinal cells28 as well as in pancreatic β-cells74 and in taste bud cells,75 indicating a pathway independent from intracellular calcium fluxes.
Apart from their sweetness, steviol glycosides are also known for their bitter taste. Steviol glycosides activate two human bitter taste receptors, TAS2R4 and TAS2R14.3 Many bitter receptors are expressed along the GI tract76 and their activation has been related to GLP-1 and CCK secretion.30,32,77,78 Here, we show that out of 34 mouse Tas2rs, rebA activates three bitter taste receptors, namely Tas2r108, Tas2r123 and Tas2r134. All three were found to be expressed in STC-1 cells, although Tas2r123 and Tas2r134 at a much lower expression level than Tas2r108. The Tas2r108 has been linked before to GLP-1 release.36 Kok et al. showed that the bitter isohumulone KDT501 induced GLP-1 secretion in a Tas2r108-dependent manner, and improved glucose tolerance when orally administered to pre-diabetic mice.36 Also, another Tas2r108 agonist (emetine) lowered blood glucose.36 Interestingly, the rebA-responsive human TAS2R4 is the ortholog of the murine Tas2r108. However, it has been observed that orthologous bitter taste receptors of human and mouse rarely share similar response profiles.46
Subsequently, in the absence of established antagonists for the murine bitter taste receptors, we explored in HuTu-80 the effects of available inhibitors for human TAS2R4 and TAS2R14. Previously, GABA was shown to inhibit TAS2R4 quinine-induced activation.53 In our set-up, GABA significantly blocked GLP-1 release in rebA-stimulated HuTu-80 cells, by maximally 21% at 1 μM. This concentration is around the IC50 value of 3.2 μM as previously determined in TAS2R4-overexpressing HEK293 cells.53 Modeling studies with TAS2R4 receptors located a GABA binding site at the more intracellular regions,53 compared to the extracellular binding sites predicted for rebA.79,80 This might explain why preincubation with GABA was a necessity and we did not have full inhibition of rebA-mediated effects on GLP-1 release.
By contrast, the reported TAS2R14 antagonist 6-MF54 did not inhibit, but instead augmented GLP-1 secretion at concentrations far lower than the IC50 of around 500 μM as shown for HEK293 cells functionally overexpressing TAS2R14.54 Remarkably, we found that 6-MF in a concentration range between 1 nM and 50 nM largely enhanced, up to 55%, rebA-stimulated GLP-1-release. It should be further studied how lower concentrations of 6-MF in combination with rebA can elicit the robust increase seen. Interestingly, GABA seemed to inhibit the enhancement of 6-MF on the rebA-induced GLP-1 release. Altogether, this suggests that GABA attenuates rebA-mediated GLP-1 secretion involving TAS2R4, and that TAS2R14-activation either contributes to rebA-mediated effect on GLP-1 or exerts some modulation on TAS2R4 as well. However, while rebA-induced GLP-1 release in HuTu-80 was partly inhibited by GABA, we found no effects of colchicine, a human TAS2R4 agonist, on GLP-1 release. Among the 33 ligands listed in BitterDB, the database of bitter compounds,55 there is no specific ligand for TAS2R4. Colchicine is a ligand that activates TAS2R4 and two other human bitter taste receptors (TAS2R39 and TAS2R46),81 but it shows highest affinity for TAS2R4.55 There are 151 reported ligands for TAS2R14, which is a known promiscuous receptor.82,83 The two ligands tested in our study, 4-hydroxyanisol and flufenamic acid are reported to uniquely activate TAS2R14,81 with flufenamic acid having a higher affinity than 4-hydroxyanisol (0.01 μM and 300 μM, respectively). Nevertheless, none of these bitter ligands elicited GLP-1 secretion in HuTu-80 cells. Of note, we cannot rule out that ligand–receptor activation in EECs differs from HEK-screening models in underlying signalling cascades. Therefore, further research is warranted to investigate the interactions and subsequent signalling processes of rebA with specific taste receptors.
Except for TAS2R4, we studied another candidate potentially involved in the rebA-induced GLP-1 release, namely TRPM5. TRPM5 plays a central role as intrinsic signalling element in the taste transduction cascade of chemosensory cells,84 and has also been found to be extra-orally expressed.26,40 Philippaert and collaborators have shown that steviol glycosides enhance pancreatic β-cell function by potentiation of TRPM5 channel activity.40 Also, the authors have shown the involvement of TRPM5 in the effect of stevioside preventing high fat diet-induced glucose intolerance in mice. For that reason, we included TRPM5 in our search for pathways possibly involved in the rebA-stimulated release of GLP-1 by EECs. The specific antagonist TPPO attenuated rebA-induced GLP-1 release in human HuTu-80 cells (27%). It is not clear if an effect of rebA on TRPM5, being located downstream in the bitter taste signalling cascade,41,42,84 is a result of taste receptor activation or of a direct interaction with the TRPM5 channel. Philippaert et al.40 suggested an interplay between rebA and the TRPM5 channels at the cell membrane of the β-pancreatic cell. We observed only a non-significant small trend in GLP-1 release when using the ionophore ionomycin, suggesting that calcium-ion transport from the endoplasmic reticulum towards the cytosol may not be the exclusive trigger for rebA-induced GLP-1 secretion, and therefore, supports the idea of a direct interplay of rebA with TRPM5, thereby potentiating the Na+ channel TRMP5.
The concentrations of rebaudioside A used in our cellular experiments are in line with the levels reached with normal consumption of stevia-containing products. The European Food Safety Authority (EFSA) has established an acceptable daily intake of 1 g day−1 of rebA (>97% purity) for an 80 kg person.1 Therefore, the effective concentration of 750 μM rebA used in vitro would be equivalent to the use of 172 mg rebA in a medium coffee cup (barista standard cup of 8 fl. oz., or 237 mL, with 3–4 drops of liquid stevia). Our findings also suggest that rebA-induced effects on GLP-1 can be modulated by other compounds present in the diet. For example, GABA, present in tomatoes85,86 and potatoes87,88 and 6-MF, as a constituent of citrus fruits,89 can have opposite effects on rebA-mediated effects. These data highlight the potential for interactions of dietary constituents with rebA at the taste receptor level, which warrants further investigation.
In conclusion, while both humans and mice can taste rebA via bitter- and sweet taste receptors, our data suggest that the stimulation of GLP-1 release from EECs by rebA occurs through interaction with bitter taste signalling, and not via sweet taste receptors. Specifically, our findings implicate that TAS2R4 and TRPM5 can act as modulators of rebA-elicited GLP-1 release, suggesting that these receptors could be targets to enhance incretin GLP-1 release through nutrition. Moreover, interactions with other food constituents, such as GABA, can influence rebA-mediated effects on GLP-1 and should be considered when studying dietary effects of rebA. In summary, the bitter taste of rebA which humans perceive as a lingering aftertaste appears to be relevant for enteroendocrine secretion of GLP-1.
Abbreviations
4-HA | 4-Hydroxyanisol |
6-MF | 6-Methoxyflavanone |
colch | Colchicine |
DMEM | Dulbecco's modified Eagle's Medium |
DMSO | Dimethyl sulfoxide |
EECs | Enteroendocrine cells |
ELISA | Enzyme-linked immunosorbent assay |
fluf | Flufenamic acid |
GABA | Gamma-aminobutyric acid |
GLP-1 | Glucagon-like peptide-1 |
HBSS | Hank's balanced salt solution |
HEPES | 4-(2-Hydroxyethyl)-1-piperazineethanesulphonic acid |
ion | Ionomycin |
LDH | Lactate dehydrogenase |
mRNA | Messenger RNA |
NEAA | Non-essential amino acids solution |
NR | Neutral red |
PMSF | Phenylmethanesulphonyl fluoride |
rebA | Rebaudioside A |
SEM | Standard error of the mean |
TPPO | Triphenylphosphine oxide |
Author contributions
FNL: conceptualization, methodology, investigation, formal analysis, writing – original draft, and funding acquisition. NvdW: conceptualization, methodology, investigation, formal analysis, and writing – original draft. MB: investigation, formal analysis, and writing – original draft. SR: investigation. JvA: investigation, and writing – original draft. MJ: formal analysis, and writing – original draft. RW: supervision, funding acquisition and writing – review & editing. JJM: supervision, writing – review & editing. SBN: supervision, conceptualization, methodology, and writing – review & editing. JM: conceptualization, methodology, supervision, writing – original draft, and writing – review & editing.
Conflicts of interest
There are no conflicts to declare.
Acknowledgements
The research was supported in part by the “Agencia Nacional de Investigación y Desarrollo” (ANID), “Becas Chile/Doctorado” grant, 2016-Folio 72170330 (F. N. L.), the TI Food and Nutrition fund, a Public-private Partnership on Pre-competitive Research in Food and Nutrition (N. v. d. W., J. M., and R. W.), and the financial support “Covid-19 compensation” given by Stichting Wageningen Research (F. N. L.). S. B. N. and J. J. M. acknowledge the financial support of the ‘Healthy and Safe Food System (KB-37)’ knowledge base program (KB-37-001-007) of the Dutch Ministry of Agriculture, Nature and Food Quality (Ministerie van LNV). The funders had no role in study design, data collection and analysis, or preparation of the manuscript. The authors thank Ulrike Redel for excellent technical assistance. We also thank Loic Briand, CNRS, INRAE, Institut Agro, Dijon, France, who kindly provided us with gurmarin.
References
- EFSA, Scientific Opinion on the safety of steviol glycosides for the proposed uses as a food additive, EFSA J., 2010, 8, 1537–1620 Search PubMed.
- U. Wolwer-Rieck, The leaves of Stevia rebaudiana (Bertoni), their constituents and the analyses thereof: a review, J. Agric. Food Chem., 2012, 60, 886–895 CrossRef PubMed.
- C. Hellfritsch, A. Brockhoff, F. Stahler, W. Meyerhof and T. Hofmann, Human psychometric and taste receptor responses to steviol glycosides, J. Agric. Food Chem., 2012, 60, 6782–6793 CrossRef CAS PubMed.
-
G. E. DuBois, D. E. Walters, S. S. Schiffman, Z. S. Warwick, B. J. Booth, S. D. Pecore, K. Gibes, B. T. Carr and L. M. Brands, in Sweeteners, American Chemical Society, 1991, ch. 20, vol. 450, pp. 261–276 Search PubMed.
- A. L. Allen, J. E. McGeary and J. E. Hayes, Rebaudioside A and Rebaudioside D bitterness do not covary with Acesulfame K bitterness or polymorphisms in TAS2R9 and TAS2R31, Chemosens. Percept., 2013, 6, 109–117 CrossRef CAS PubMed.
- P. Samuel, K. T. Ayoob, B. A. Magnuson, U. Wolwer-Rieck, P. B. Jeppesen, P. J. Rogers, I. Rowland and R. Mathews, Stevia Leaf to Stevia Sweetener: Exploring Its Science, Benefits, and Future Potential, J. Nutr., 2018, 148, 1186S–1205S CrossRef PubMed.
- K. A. Higgins and R. D. Mattes, A randomized controlled trial contrasting the effects of 4 low-calorie sweeteners and sucrose on body weight in adults with overweight or obesity, Am. J. Clin. Nutr., 2019, 109, 1288–1301 CrossRef PubMed.
- K. C. Maki, L. L. Curry, M. C. Carakostas, S. M. Tarka, M. S. Reeves, M. V. Farmer, J. M. McKenney, P. D. Toth, S. L. Schwartz, B. C. Lubin, M. R. Dicklin, A. C. Boileau and J. D. Bisognano, The hemodynamic effects of rebaudioside A in healthy adults with normal and low-normal blood pressure, Food Chem. Toxicol., 2008, 46(Suppl. 7), S40–S46 CrossRef CAS PubMed.
- K. C. Maki, L. L. Curry, M. S. Reeves, P. D. Toth, J. M. McKenney, M. V. Farmer, S. L. Schwartz, B. C. Lubin, A. C. Boileau, M. R. Dicklin, M. C. Carakostas and S. M. Tarka, Chronic consumption of rebaudioside A, a steviol glycoside, in men and women with type 2 diabetes mellitus, Food Chem. Toxicol., 2008, 46(Suppl 7), S47–S53 CrossRef CAS PubMed.
- L. A. Barriocanal, M. Palacios, G. Benitez, S. Benitez, J. T. Jimenez, N. Jimenez and V. Rojas, Apparent lack of pharmacological effect of steviol glycosides used as sweeteners in humans. A pilot study of repeated exposures in some normotensive and hypotensive individuals and in Type 1 and Type 2 diabetics, Regul. Toxicol. Pharmacol., 2008, 51, 37–41 CrossRef CAS PubMed.
- S. D. Anton, C. K. Martin, H. Han, S. Coulon, W. T. Cefalu, P. Geiselman and D. A. Williamson, Effects of stevia, aspartame, and sucrose on food intake, satiety, and postprandial glucose and insulin levels, Appetite, 2010, 55, 37–43 CrossRef CAS PubMed.
- S. Gregersen, P. B. Jeppesen, J. J. Holst and K. Hermansen, Antihyperglycemic effects of stevioside in type 2 diabetic subjects, Metab., Clin. Exp., 2004, 53, 73–76 CrossRef CAS PubMed.
- Y. Fujita, R. D. Wideman, M. Speck, A. Asadi, D. S. King, T. D. Webber, M. Haneda and T. J. Kieffer, Incretin release from gut is acutely enhanced by sugar but not by sweeteners in vivo, Am. J. Physiol. Endocrinol. Metab., 2009, 296, E473–E479 CrossRef CAS PubMed.
- B. Geeraert, F. Crombe, M. Hulsmans, N. Benhabiles, J. M. Geuns and P. Holvoet, Stevioside inhibits atherosclerosis by improving insulin signaling and antioxidant defense in obese insulin-resistant mice, Int. J. Obes., 2010, 34, 569–577 CrossRef CAS PubMed.
- R. Saravanan and V. Ramachandran, Modulating
efficacy of Rebaudioside A, a diterpenoid on antioxidant and circulatory lipids in experimental diabetic rats, Environ. Toxicol. Pharmacol., 2013, 36, 472–483 CrossRef CAS PubMed.
- M. van Avesaat, F. J. Troost, D. Ripken, J. Peters, H. F. Hendriks and A. A. Masclee, Intraduodenal infusion of a combination of tastants decreases food intake in humans, Am. J. Clin. Nutr., 2015, 102, 729–735 CrossRef CAS PubMed.
- N. S. Stamataki, C. Scott, R. Elliott, S. McKie, D. Bosscher and J. T. McLaughlin, Stevia Beverage Consumption prior to Lunch Reduces Appetite and Total Energy Intake without Affecting Glycemia or Attentional Bias to Food Cues: A Double-Blind Randomized Controlled Trial in Healthy Adults, J. Nutr., 2020, 150, 1126–1134 CrossRef PubMed.
- M. C. Carakostas, L. L. Curry, A. C. Boileau and D. J. Brusick, Overview: the history, technical function and safety of rebaudioside A, a naturally occurring steviol glycoside, for use in food and beverages, Food Chem. Toxicol., 2008, 46(Suppl 7), S1–S10 CrossRef CAS PubMed.
- N. van der Wielen, M. van Avesaat, N. J. de Wit, J. T. Vogels, F. Troost, A. Masclee, S. J. Koopmans, J. van der Meulen, M. V. Boekschoten, M. Muller, H. F. Hendriks, R. F. Witkamp and J. Meijerink, Cross-species comparison of genes related to nutrient sensing mechanisms expressed along the intestine, PLoS One, 2014, 9, e107531 CrossRef PubMed.
- P. Lu, C. H. Zhang, L. M. Lifshitz and R. ZhuGe, Extraoral bitter taste receptors in health and disease, J. Gen. Physiol., 2017, 149, 181–197 CrossRef CAS PubMed.
- K. Sjölund, G. Sandén, R. Håkanson and F. Sundler, Endocrine Cells in Human Intestine: An Immunocytochemical Study, Gastroenterology, 1983, 85, 1120–1130 CrossRef.
- Y. Song, J. A. Koehler, L. L. Baggio, A. C. Powers, D. A. Sandoval and D. J. Drucker, Gut-Proglucagon-Derived Peptides Are Essential for Regulating Glucose Homeostasis in Mice, Cell Metab., 2019, 30, 976–986 CrossRef CAS PubMed.
- L. van Bloemendaal, R.G IJzerman, J. S. ten Kulve, F. Barkhof, R. J. Konrad, M. L. Drent, D. J. Veltman and M. Diamant, GLP-1 receptor activation modulates appetite- and reward-related brain areas in humans, Diabetes, 2014, 63, 4186–4196 CrossRef CAS PubMed.
- H. R. Berthoud, Vagal and hormonal gut-brain communication: from satiation to satisfaction, Neurogastroenterol. Motil., 2008, 20(Suppl 1), 64–72 CrossRef CAS PubMed.
- J. E. Campbell and D. J. Drucker, Pharmacology, physiology, and mechanisms of incretin hormone action, Cell Metab., 2013, 17, 819–837 CrossRef CAS PubMed.
- H. J. Jang, Z. Kokrashvili, M. J. Theodorakis, O. D. Carlson, B. J. Kim, J. Zhou, H. H. Kim, X. Xu, S. L. Chan, M. Juhaszova, M. Bernier, B. Mosinger, R. F. Margolskee and J. M. Egan, Gut-expressed gustducin and taste receptors regulate secretion of glucagon-like peptide-1, Proc. Natl. Acad. Sci. U. S. A., 2007, 104, 15069–15074 CrossRef CAS PubMed.
- R. E. Steinert, A. C. Gerspach, H. Gutmann, L. Asarian, J. Drewe and C. Beglinger, The functional involvement of gut-expressed sweet taste receptors in glucose-stimulated secretion of glucagon-like peptide-1 (GLP-1) and peptide YY (PYY), Clin. Nutr., 2011, 30, 524–532 CrossRef CAS PubMed.
- Y. Ohtsu, Y. Nakagawa, M. Nagasawa, S. Takeda, H. Arakawa and I. Kojima, Diverse signaling systems activated by the sweet taste receptor in human GLP-1-secreting cells, Mol. Cell. Endocrinol., 2014, 394, 70–79 CrossRef CAS PubMed.
- R. E. Kuhre, F. M. Gribble, B. Hartmann, F. Reimann, J. A. Windelov, J. F. Rehfeld and J. J. Holst, Fructose stimulates GLP-1 but not GIP secretion in mice, rats, and humans, Am. J. Physiol.: Gastrointest. Liver Physiol., 2014, 306, G622–G630 CrossRef CAS PubMed.
- K. S. Kim, J. M. Egan and H. J. Jang, Denatonium induces secretion of glucagon-like peptide-1 through activation of bitter taste receptor pathways, Diabetologia, 2014, 57, 2117–2125 CrossRef CAS PubMed.
- K.-S. Kim, N. H. Cha, K.-W. Kim, M. H. Shin, K.-H. Kim, I.-S. Lee, W.-S. Chung, M.-Y. Song and H.-J. Jang, Transcriptomic analysis of the bitter taste receptor-mediated glucagon-like peptide-1 stimulation effect of quinine, BioChip J., 2013, 7, 386–392 CrossRef CAS.
- Y. Yu, G. Hao, Q. Zhang, W. Hua, M. Wang, W. Zhou, S. Zong, M. Huang and X. Wen, Berberine induces GLP-1 secretion through activation of bitter taste receptor pathways, Biochem. Pharmacol., 2015, 97, 173–177 CrossRef CAS PubMed.
- H. Pham, H. Hui, S. Morvaridi, J. Cai, S. Zhang, J. Tan, V. Wu, N. Levin, B. Knudsen, W. A. Goddard 3rd, S. J. Pandol and R. Abrol, A bitter pill for type 2 diabetes? The activation of bitter taste receptor TAS2R38 can stimulate GLP-1 release from enteroendocrine L-cells, Biochem. Biophys. Res. Commun., 2016, 475, 295–300 CrossRef CAS PubMed.
- X. Yue, J. Liang, F. Gu, D. Du and F. Chen, Berberine activates bitter taste responses of enteroendocrine STC-1 cells, Mol. Cell. Biochem., 2018, 447, 21–32 CrossRef CAS PubMed.
- K. Harada, H. Sakaguchi, S. Sada, R. Ishida, Y. Hayasaka and T. Tsuboi, Bitter tastant quinine modulates glucagon-like peptide-1 exocytosis from clonal GLUTag enteroendocrine L cells via actin reorganization, Biochem. Biophys. Res. Commun., 2018, 500, 723–730 CrossRef CAS PubMed.
- B. P. Kok, A. Galmozzi, N. K. Littlejohn, V. Albert, C. Godio, W. Kim, S. M. Kim, J. S. Bland, N. Grayson, M. Fang, W. Meyerhof, G. Siuzdak, S. Srinivasan, M. Behrens and E. Saez, Intestinal bitter taste receptor activation alters hormone secretion and imparts metabolic benefits, Mol. Metab., 2018, 16, 76–87 CrossRef CAS PubMed.
- T. Nakamura, K. Harada, T. Kamiya, M. Takizawa, J. Kuppers, K. Nakajima, M. Gutschow, T. Kitaguchi, K. Ohta, T. Kato and T. Tsuboi, Glutamine-induced signaling pathways via amino acid receptors in enteroendocrine L cell lines, J. Mol. Endocrinol., 2020, 64, 133–143 CAS.
- N. van der Wielen, J. P. ten Klooster, S. Muckenschnabl, R. Pieters, H. F. Hendriks, R. F. Witkamp and J. Meijerink, The Noncaloric Sweetener Rebaudioside A Stimulates Glucagon-Like Peptide 1 Release and Increases Enteroendocrine Cell Numbers in 2-Dimensional Mouse Organoids Derived from Different Locations of the Intestine, J. Nutr., 2016, 146, 2429–2435 CrossRef CAS PubMed.
- D. Ripken, N. van der Wielen, H. M. Wortelboer, J. Meijerink, R. F. Witkamp and H. F. Hendriks, Steviol glycoside rebaudioside A induces glucagon-like peptide-1 and peptide YY release in a porcine ex vivo intestinal model, J. Agric. Food Chem., 2014, 62, 8365–8370 CrossRef CAS PubMed.
- K. Philippaert, A. Pironet, M. Mesuere, W. Sones, L. Vermeiren, S. Kerselaers, S. Pinto, A. Segal, N. Antoine, C. Gysemans, J. Laureys, K. Lemaire, P. Gilon, E. Cuypers, J. Tytgat, C. Mathieu, F. Schuit, P. Rorsman, K. Talavera, T. Voets and R. Vennekens, Steviol glycosides enhance pancreatic beta-cell function and taste sensation by potentiation of TRPM5 channel activity, Nat. Commun., 2017, 8, 14733 CrossRef CAS PubMed.
- C. A. Perez, L. Huang, M. Rong, J. A. Kozak, A. K. Preuss, H. Zhang, M. Max and R. F. Margolskee, A transient receptor potential channel expressed in taste receptor cells, Nat. Neurosci., 2002, 5, 1169–1176 CrossRef CAS PubMed.
- Y. Zhang, M. A. Hoon, J. Chandrashekar, K. L. Mueller, B. Cook, D. Wu, C. S. Zuker and N. J. P. Ryba, Coding of Sweet, Bitter, and Umami Tastes: Different Receptor Cells Sharing Similar Signaling Pathways, Cell, 2003, 112, 293–301 CrossRef CAS PubMed.
- M. Sigoillot, A. Brockhoff, E. Lescop, N. Poirier, W. Meyerhof and L. Briand, Optimization of the production of gurmarin, a sweet-taste-suppressing protein, secreted by the methylotrophic yeast Pichia pastoris, Appl. Microbiol. Biotechnol., 2012, 96, 1253–1263 CrossRef CAS PubMed.
- G. Repetto, A. del Peso and J. L. Zurita, Neutral red uptake assay for the estimation of cell viability/cytotoxicity, Nat. Protoc., 2008, 3, 1125–1131 CrossRef CAS PubMed.
- M. Roelse, N. C. de Ruijter, E. X. Vrouwe and M. A. Jongsma, A generic microfluidic biosensor of G protein-coupled receptor activation-monitoring cytoplasmic [Ca(2+)] changes in human HEK293 cells, Biosens. Bioelectron., 2013, 47, 436–444 CrossRef CAS PubMed.
- K. Lossow, S. Hubner, N. Roudnitzky, J. P. Slack, F. Pollastro, M. Behrens and W. Meyerhof, Comprehensive Analysis of Mouse Bitter Taste Receptors Reveals Different Molecular Receptive Ranges for Orthologous Receptors in Mice and Humans, J. Biol. Chem., 2016, 291, 15358–15377 CrossRef CAS PubMed.
- B. M. Bolstad, R. A. Irizarry, M. Åstrand and T. P. Speed, A comparison of normalization methods for high density oligonucleotide array data based on variance and bias, Bioinformatics, 2003, 19, 185–193 CrossRef CAS PubMed.
- R. A. Irizarry, B. M. Bolstad, F. Collin, L. M. Cope, B. Hobbs and T. P. Speed, Summaries of Affymetrix GeneChip probe level data, Nucleic Acids Res., 2003, 31, e15 CrossRef PubMed.
- J. Barretina, G. Caponigro, N. Stransky, K. Venkatesan, A. A. Margolin, S. Kim, C. J. Wilson, J. Lehar, G. V. Kryukov, D. Sonkin, A. Reddy, M. Liu, L. Murray, M. F. Berger, J. E. Monahan, P. Morais, J. Meltzer, A. Korejwa, J. Jane-Valbuena, F. A. Mapa, J. Thibault, E. Bric-Furlong, P. Raman, A. Shipway, I. H. Engels, J. Cheng, G. K. Yu, J. Yu, P. Aspesi Jr., M. de Silva, K. Jagtap, M. D. Jones, L. Wang, C. Hatton, E. Palescandolo, S. Gupta, S. Mahan, C. Sougnez, R. C. Onofrio, T. Liefeld, L. MacConaill, W. Winckler, M. Reich, N. Li, J. P. Mesirov, S. B. Gabriel, G. Getz, K. Ardlie, V. Chan, V. E. Myer, B. L. Weber, J. Porter, M. Warmuth, P. Finan, J. L. Harris, M. Meyerson, T. R. Golub, M. P. Morrissey, W. R. Sellers, R. Schlegel and L. A. Garraway, The Cancer Cell Line Encyclopedia enables predictive modelling of anticancer drug sensitivity, Nature, 2012, 483, 603–607 CrossRef CAS PubMed.
- E. N. Dedkova, A. A. Sigova and V. P. Zinchenko, Mechanism of action of calcium ionophores on intact cells: ionophore-resistant cells, Membr. Cell Biol., 2000, 13, 357–368 CAS.
- P. Jiang, M. Cui, B. Zhao, Z. Liu, L. A. Snyder, L. M. Benard, R. Osman, R. F. Margolskee and M. Max, Lactisole interacts with the transmembrane domains of human T1R3 to inhibit sweet taste, J. Biol. Chem., 2005, 280, 15238–15246 CrossRef CAS PubMed.
- M. Matholouthi, J. F. Angiboust, M. Kacurakova, R. W. W. Hooft, J. A. Kanters and J. Kroon, Structural studies on sweet taste inhibitors: lactisole, DL-2(4-methoxyphenoxy)-propanoic acid, J. Mol. Struct., 1994, 326, 25–34 CrossRef.
- S. P. Pydi, T. Sobotkiewicz, R. Billakanti, R. P. Bhullar, M. C. Loewen and P. Chelikani, Amino acid derivatives as bitter taste receptor (T2R) blockers, J. Biol. Chem., 2014, 289, 25054–25066 CrossRef CAS PubMed.
- W. S. Roland, R. J. Gouka, H. Gruppen, M. Driesse, L. van Buren, G. Smit and J. P. Vincken, 6-methoxyflavanones as bitter taste receptor blockers for hTAS2R39, PLoS One, 2014, 9, e94451 CrossRef PubMed.
- A. Dagan-Wiener, A. Di Pizio, I. Nissim, M. S. Bahia, N. Dubovski, E. Margulis and M. Y. Niv, BitterDB: taste ligands and receptors database in 2019, Nucleic Acids Res., 2019, 47, D1179–D1185 CrossRef PubMed.
- A. Levit, S. Nowak, M. Peters, A. Wiener, W. Meyerhof, M. Behrens and M. Y. Niv, The bitter pill: clinical drugs that activate the human bitter taste receptor TAS2R14, FASEB J., 2014, 28, 1181–1197 CrossRef CAS PubMed.
- K. Talavera, K. Yasumatsu, R. Yoshida, R. F. Margolskee, T. Voets, Y. Ninomiya and B. Nilius, The taste transduction channel TRPM5 is a locus for bitter-sweet taste interactions, FASEB J., 2008, 22, 1343–1355 CrossRef CAS PubMed.
- Y. Cui, H. Wu, Q. Li, J. Liao, P. Gao, F. Sun, H. Zhang, Z. Lu, X. Wei, C. He, T. Ma, X. Wei, X. Chen, H. Zheng, G. Yang, D. Liu and Z. Zhu, Impairment of Bitter Taste Sensor Transient Receptor Potential Channel M5-Mediated Aversion Aggravates High-Salt Intake and Hypertension, Hypertension, 2019, 74, 1021–1032 CrossRef CAS PubMed.
- D. Dutta Banik, L. E. Martin, M. Freichel, A. M. Torregrossa and K. F. Medler, TRPM4 and TRPM5 are both required for normal signaling in taste receptor cells, Proc. Natl. Acad. Sci. U. S. A., 2018, 115, E772–E781 CrossRef PubMed.
- R. K. Palmer, K. Atwal, I. Bakaj, S. Carlucci-Derbyshire, M. T. Buber, R. Cerne, R. Y. Cortes, H. R. Devantier, V. Jorgensen, A. Pawlyk, S. P. Lee, D. G. Sprous, Z. Zhang and R. Bryant, Triphenylphosphine oxide is a potent and selective inhibitor of the transient receptor potential melastatin-5 ion channel, Assay Drug Dev. Technol., 2010, 8, 703–713 CrossRef CAS PubMed.
- A. Sclafani, M. Bahrani, S. Zukerman and K. Ackroff, Stevia and saccharin preferences in rats and mice, Chem. Senses, 2010, 35, 433–443 CrossRef CAS PubMed.
- K. Yamamoto and Y. Ishimaru, Oral and extra-oral taste perception, Semin. Cell Dev. Biol., 2013, 24, 240–246 CrossRef PubMed.
- J. Dyer, K. S. Salmon, L. Zibrik and S. P. Shirazi-Beechey, Expression of sweet taste receptors of the T1R family in the intestinal tract and enteroendocrine cells, Biochem. Soc. Trans., 2005, 33, 302–305 CrossRef CAS PubMed.
- R. F. Margolskee, J. Dyer, Z. Kokrashvili, K. S. H. Salmon, E. Ilegems, K. Daly, E. L. Maillet, Y. Ninomiya, B. Mosinger and S. P. Shirazi-Beechey, T1R3 and gustducin in gut sense sugars to regulate expression of Na+-glucose cotransporter 1, Proc. Natl. Acad. Sci. U. S. A., 2007, 104, 15075–15080 CrossRef CAS PubMed.
- F. Wang, X. Song, L. Zhou, G. Liang, F. Huang, G. Jiang and L. Zhang, The downregulation of sweet taste receptor signaling in enteroendocrine L-cells mediates 3-deoxyglucosone-induced attenuation of high glucose-stimulated GLP-1 secretion, Arch. Physiol. Biochem., 2018, 124, 430–435 CrossRef CAS PubMed.
- R. E. Kuhre, C. R. Frost, B. Svendsen and J. J. Holst, Molecular mechanisms of glucose-stimulated GLP-1 secretion from perfused rat small intestine, Diabetes, 2015, 64, 370–382 CrossRef CAS PubMed.
- F. M. Gribble, L. Williams, A. K. Simpson and F. Reimann, A novel glucose-sensing mechanism contributing to glucagon-like peptide-1 secretion from the GLUTag cell line, Diabetes, 2003, 52, 1147–1154 CrossRef CAS PubMed.
- F. Reimann, A. M. Habib, G. Tolhurst, H. E. Parker, G. J. Rogers and F. M. Gribble, Glucose sensing in L cells: a primary cell study, Cell Metab., 2008, 8, 532–539 CrossRef CAS PubMed.
- H. E. Parker, K. Wallis, C. W. le Roux, K. Y. Wong, F. Reimann and F. M. Gribble, Molecular mechanisms underlying bile acid-stimulated glucagon-like peptide-1 secretion, Br. J. Pharmacol., 2012, 165, 414–423 CrossRef CAS PubMed.
- C. Thomas, A. Gioiello, L. Noriega, A. Strehle, J. Oury, G. Rizzo, A. Macchiarulo, H. Yamamoto, C. Mataki, M. Pruzanski, R. Pellicciari, J. Auwerx and K. Schoonjans, TGR5-mediated bile acid sensing controls glucose homeostasis, Cell Metab., 2009, 10, 167–177 CrossRef CAS PubMed.
- S. Katsuma, A. Hirasawa and G. Tsujimoto, Bile acids promote glucagon-like peptide-1 secretion through TGR5 in a murine enteroendocrine cell line STC-1, Biochem. Biophys. Res. Commun., 2005, 329, 386–390 CrossRef CAS PubMed.
- S. Sundaresan, R. Shahid, T. E. Riehl, R. Chandra, F. Nassir, W. F. Stenson, R. A. Liddle and N. A. Abumrad, CD36-dependent signaling mediates fatty acid-induced gut release of secretin and cholecystokinin, FASEB J., 2013, 27, 1191–1202 CrossRef CAS PubMed.
- A. K. Simpson, P. S. Ward, K. Y. Wong, G. J. Collord, A. M. Habib, F. Reimann and F. M. Gribble, Cyclic AMP triggers glucagon-like peptide-1 secretion from the GLUTag enteroendocrine cell line, Diabetologia, 2007, 50, 2181–2189 CrossRef CAS PubMed.
- Y. Nakagawa, M. Nagasawa, H. Mogami, M. Lohse, Y. Ninomiya and I. Kojima, Multimodal function of the sweet taste receptor expressed in pancreatic β-cells: generation of diverse patterns of intracellular signals by sweet agonists, Endocr. J., 2013, 60, 1191–1206 CrossRef CAS PubMed.
- K. R. Trubey, S. Culpepper, Y. Maruyama, S. C. Kinnamon and N. Chaudhari, Tastants evoke cAMP signal in taste buds that is independent of calcium signaling, Am. J. Physiol.: Cell Physiol., 2006, 291, C237–C244 CrossRef CAS PubMed.
- S. V. Wu, N. Rozengurt, M. Yang, S. H. Young, J. Sinnett-Smith and E. Rozengurt, Expression of bitter taste receptors of the T2R family in the gastrointestinal tract and enteroendocrine STC-1 cells, Proc. Natl. Acad. Sci. U. S. A., 2002, 99, 2392–2397 CrossRef CAS PubMed.
- T. I. Jeon, Y. K. Seo and T. F. Osborne, Gut bitter taste receptor signalling induces ABCB1 through a mechanism involving CCK, Biochem. J., 2011, 438, 33–37 CrossRef CAS PubMed.
- B. Le Neve, M. Foltz, H. Daniel and R. Gouka, The steroid glycoside H.g.-12 from Hoodia gordonii activates the human bitter receptor TAS2R14 and induces CCK release from HuTu-80 cells, Am. J. Physiol.: Gastrointest. Liver Physiol., 2010, 299, G1368–G1375 CrossRef CAS PubMed.
- W. Acevedo, F. Gonzalez-Nilo and E. Agosin, Docking and Molecular Dynamics of Steviol Glycoside-Human Bitter Receptor Interactions, J. Agric. Food Chem., 2016, 64, 7585–7596 CrossRef CAS PubMed.
- S. K. Kim, Y. Chen, R. Abrol, W. A. Goddard 3rd and B. Guthrie, Activation mechanism of the G protein-coupled sweet receptor heterodimer with sweeteners and allosteric agonists, Proc. Natl. Acad. Sci. U. S. A., 2017, 114, 2568–2573 CrossRef CAS PubMed.
- W. Meyerhof, C. Batram, C. Kuhn, A. Brockhoff, E. Chudoba, B. Bufe, G. Appendino and M. Behrens, The molecular receptive ranges of human TAS2R bitter taste receptors, Chem. Senses, 2010, 35, 157–170 CrossRef CAS PubMed.
- M. Behrens, A. Brockhoff, C. Kuhn, B. Bufe, M. Winnig and W. Meyerhof, The human taste receptor hTAS2R14 responds to a variety of different bitter compounds, Biochem. Biophys. Res. Commun., 2004, 319, 479–485 CrossRef CAS PubMed.
- A. Di Pizio and M. Y. Niv, Promiscuity and selectivity of bitter molecules and their receptors, Bioorg. Med. Chem., 2015, 23, 4082–4091 CrossRef CAS PubMed.
- S. Kaske, G. Krasteva, P. Konig, W. Kummer, T. Hofmann, T. Gudermann and V. Chubanov, TRPM5, a taste-signaling transient receptor potential ion-channel, is a ubiquitous signaling component in chemosensory cells, BMC Neurosci., 2007, 8, 49 CrossRef PubMed.
- T. de Bie, M. Balvers, M. Jongsma and R. Witkamp, Dietary Neurotransmitters: The Relative Oral Bioavailability of GABA and Glutamic Acid From Tomato in Healthy Human Volunteers, Curr. Dev. Nutr., 2021, 5, 311–311 CrossRef.
- M. Takayama and H. Ezura, How and why does tomato accumulate a large amount of GABA in the fruit?, Front. Plant Sci., 2015, 6, 612 Search PubMed.
- S.-H. Oh, Y.-J. Moon and C.-H. Oh, γ-Aminobutyric Acid (GABA) Content of Selected Uncooked Foods, Prev. Nutr. Food Sci., 2003, 8, 75–78 CrossRef CAS.
- M. Diana, J. Quílez and M. Rafecas, Gamma-aminobutyric acid as a bioactive compound in foods: a review, J. Funct. Foods, 2014, 10, 407–420 CrossRef CAS.
- M. K. Khan, E. H. Zill and O. Dangles, A comprehensive review on flavanones, the major citrus polyphenols, J. Food Compos. Anal., 2014, 33, 85–104 CrossRef CAS.
|
This journal is © The Royal Society of Chemistry 2023 |