DOI:
10.1039/D2FO03975C
(Paper)
Food Funct., 2023,
14, 2212-2222
Bovine milk-derived extracellular vesicles prevent gut inflammation by regulating lipid and amino acid metabolism†
Received
22nd December 2022
, Accepted 13th January 2023
First published on 9th February 2023
Abstract
Inflammatory bowel disease (IBD) is a global health problem in which metabolite alteration plays an important pathogenic role. Bovine milk-derived extracellular vesicles (mEVs) have been shown to regulate nutrient metabolism in healthy animal models. This study investigated the effect of oral mEVs on metabolite changes in DSS-induced murine colitis. We performed metabolomic profiling on plasma samples and measured the concentrations of lipids and amino acids in both fecal samples and colonic tissues. Plasma metabolome analysis found that mEVs significantly upregulated 148 metabolite levels and downregulated 44 metabolite concentrations (VIP > 1, and p < 0.05). In the fecal samples, mEVs significantly increased the contents of acetate and butyrate and decreased the levels of tridecanoic acid (C13:0), methyl cis-10-pentadecenoate (C15:1) and cis-11-eicosenoic acid (C20:1). Moreover, the concentrations of eicosadienoic acid (C20:2), eicosapentaenoic acid (C20:5), and docosahexaenoic acid (C22:6) were decreased in colonic tissues with mEV supplementation. In addition, compared with the DSS group, mEVs significantly increased the content of L-arginine, decreased the level of L-valine in the fecal samples, and also decreased the levels of L-serine and L-glutamate in the colonic tissues. Collectively, our findings demonstrated that mEVs could recover the metabolic abnormalities caused by inflammation and provided novel insights into mEVs as a potential modulator for metabolites to prevent and treat IBD.
Introduction
Inflammatory bowel disease (IBD), Crohn's disease (CD), and ulcerative colitis (UC) are collective terms for human gastrointestinal tract diseases.1 In the western world, the prevalence of IBD is now up to 0.5% and is forecasted to reach 1% of the population by 2030.2–4 In China, the incidence of IBD has risen dramatically with the modernization of society. Given the substantial effects of IBD on all aspects of individual lives and the huge burden of overall health expenditure, research into the treatment of IBD is urgently needed.5 Previous studies have revealed that more than 200 susceptibility loci,6 dysbiosis of intestinal microbes, and metabolites alteration7 contribute to the development of IBD.
Altered metabolites in numerous biological tissues, such as feces, urine, blood, gut, liver, and cerebrospinal fluid, could explain the role of metabolites in disease development and progress and provide novel candidate biomarkers for disease diagnosis.8,9 In IBD patients, nutrient metabolism is significantly changed, with a decrease in carbohydrate and nucleotide metabolism in favor of increased lipid and amino acid metabolism.10 Moreover, the potential link between lipid profiling and colitis has been documented, which shows that the balance between the pro-inflammatory and anti-inflammatory bioactive lipid mediators is disrupted.11,12 The alteration of amino acid profiles in biofluids and intestinal tissues is closely related with the severity of disease in IBD patients.13 Previous studies reported that about 17 fecal amino acids were higher in IBD patients compared with healthy individuals.14,15 In brief, the regulation of lipid and amino acid metabolism is believed to be a promising method to treat IBD.
Bovine milk-derived extracellular vesicles (mEVs), carrying unique RNAs, proteins, lipids, and DNAs, have potential anti-degradation, anti-oxidation, and anti-inflammatory biological properties, with known therapeutic benefits in a murine model of colitis.16–18 As a natural compound, mEVs have been reported to regulate lipid and amino acid metabolism in healthy mice.19 Although several studies have demonstrated the anti-inflammatory effects and mechanism of mEVs by transcription and microbiome in vivo,17,20 the potential mechanism of mEVs to attenuate intestinal inflammation is still unclear, especially from the perspective of lipid and amino acid metabolism. Metabolomics can quantify small molecules (<1500 Da) in body fluids or tissues and characterize the metabolic profile of a biological system. Therefore, we performed untargeted liquid chromatography–mass spectrometry (LC–MS) and targeted metabolomics of plasma, feces, and tissues in murine model of colitis offered mEVs, hoping to elucidate the protective role and mechanism of mEVs against colitis.
Materials and methods
Ethics statement
All the experimental design and operations were performed following the guidelines for Care and Use of Laboratory Animals of the Chinese Academy of Agricultural Sciences and was approved by the Animal Ethics Committee of Chinese Academy of Agricultural Sciences (approval number: IAS2021-235).
Bovine mEVs isolation and characterization
Isolation of bovine mEVs was carried out as previously described.21 All centrifugation was performed at 4 °C. Whole milk samples were centrifuged at 3000g for 30 min and subsequently at 12
000g for 60 min to remove fat globules, casein aggregates, and residual chymosin. Then, the harvested milk supernatants were centrifuged at 30
000g, 75
000g for 60 min to further remove large particles and other impurities. Next, whey was centrifuged at 120
000g for 90 min with a SW32Ti rotor (Optima XPN-100; Beckman Coulter Instru-ments, Fullerton, CA, USA) to obtain mEVs pellet. The pelleted mEVs were resuspended in PBS, filtered by a 0.22 μm membrane filter (Millipore, USA), and kept at −80 °C until use.
Western blotting (WB) was used to identify the positive marker proteins on the mEVs surface, including HSP70, TSG101, CD63, and CD9 (cat. no. ab275018, Abcam, UK). The negative marker proteins on the mEVs surface were also verified (cat. no. ab275018, Abcam, UK). The morphology of mEVs was observed using transmission electron microscopy (TEM) (HT7700, Hitachi, Japan). The particle size and concentration were measured by nanoparticle tracking analysis (NTA) with ZetaView PMX 110 and ZetaView v8.04.02 SP2 software (Particle Metrix, Meerbusch, Germany). The results of WB, TEM, and NTA21 are presented in Fig. S1.† These results demonstrated that mEVs were successfully extracted.
Induction of acute colitis
Six weeks old C57BL/6 male mice were originally purchased from SPF Biotechnology Co., Ltd (Beijing, China). The mice were housed under specific pathogen-free (SPF) environment to adapt to the laboratory conditions, with free access to food and water. For dextran sulfate sodium (DSS)-induced colitis (molecular weight 36
000–50
000 kDa; MP Biomedicals, CA, USA), mice were provided 3.5% (w/v) DSS in their drinking water for 7 days continuously. To test the role of mEVs on colitis model, mice were orally administrated the mEVs for 30 days before the DSS treatment (3.0 × 109 particles per gram body weight/mouse). Body weight was monitored daily. Disease activity index (DAI) was evaluated by weight loss, stool consistency, and presence of blood in the feces. Finally, mice were euthanatized and sacrificed, and plasma, colon tissues, and colon contents were obtained for the following experiments. A portion of the collected colons was subjected to further histology analysis.
Quantification of inflammatory cytokines in the plasma
Fresh blood was transferred into centrifuge tubes containing heparin sodium and centrifuged at 3000g for 10 min at 4 °C to obtain the plasma. The concentrations of cytokines including IL-6 (cat. no. VAL604, R&D), IL-1β (cat. no. 1210122, Dakewe), and IL-10 (cat. no. 1211002, Dakewe) in the plasma were measured by ELISA according to the manufacturers’ recommendations.
Metabolite extraction and analysis
The thawed plasma (80 μL) was added with L-2-chlorophenylalanine (20 μL) as the internal standard. The sample was mixed by vortexing for 10 s and then addeded with 240 μL ice-cold mixture of methanol and acetonitrile (2/1, v/v) to precipitate protein. The mixture was vortexed for 30 s, ultrasonicated for 10 min in ice-water bath, and placed at −20 °C for 30 min. Then, it was centrifuged at 15
000g at 4 °C for 10 min. The supernatant (200 μL) was transferred to a glass vial and dried in a freeze concentration centrifugal dryer. About 120 μL of reconstitution fluid (acetonitrile
:
water = 1
:
4, v/v) was added into the supernatant sample, followed by vortexing for 30 s, ultrasonication for 3 min, and placed at −20 °C for 2 h. The sample was then centrifugated at 15
000g at 4 °C for 10 min. The extract was filtered by a 0.22 μm membrane filter (Millipore, USA) and transferred to autosampler vials for LC-MS analysis. The quality control (QC) samples were prepared by mixing equal volumes of all the samples.
The extracted metabolites were conducted using an ACQUITY UPLC I-Class Plus ultraperformance liquid tandem QE high resolution mass spectrometer (Thermo Fisher Scientific, Waltham, MA, USA), equipped with an Acquity UPLC HSS T3 column (100 mm × 2.1 mm × 1.8 μm; Waters Corporation, Milford, USA). The mobile phase included solvent A (water, 0.1% formic acid) and solvent B (acetonitrile, 0.1% formic acid), and the flow rate was 0.35 mL min−1 with 2 μL injection volume. The optimal elution procedure gradient was as follows: 0 min, 5% B; 2 min, 5% B; 4 min, 25% B; 8 min, 50% B; 10 min, 80% B; 14 min, 100% B; 15 min, 100% B; 15.1 min, 5% and 16 min, 5% B.
The raw LC-MS data were treated by Progenesis QI V2.3 software (Nonlinear, Dynamics, Newcastle, UK) for baseline filtering, peak identification, retention time correction, peak alignment, and normalization. The extracted data removed the peaks with a missing value (ion intensity = 0) of more than 50% in groups and filtrated based on the qualitative results of the compounds. Human metabolome database (HMDB), and Metlin database were used to do qualitative analysis. The data matrix was imported into the R to perform principal component analysis (PCA) and orthogonal partial least squares discriminant analysis (OPLS-DA). Variable importance of projection (VIP) values obtained from the OPLS-DA analysis and Student's t-test were used to screen differential metabolites (VIP > 1 and p < 0.05). Differential metabolites were mapped to Kyoto Encyclopedia of Genes and Genomes (KEGG) database and gained enriched pathways.
Amino acid profile analysis
The feces and colonic tissues samples were mixed with a 10% sulfosalicylic acid solution to deproteinize and placed at 4 °C for overnight. Thereafter, the supernatant of the sample was transferred into a new tube and added with methanol in a 1
:
1 (v/v) ratio. The mixed solution was centrifuged at 12
000g for 10 min and filtrated by a 0.2 μm Millipore filter. Finally, the extracted supernatant was transferred into vials for free amino acid analysis.
The amino acid profile was analyzed using ultrahigh-performance liquid chromatography-tandem mass spectrometry (UHPLC-MS/MS) method performed by the Agilent 6470 triple quadrupole mass spectrometer system, furnished with a G1312B Agilent 1290 Infinity binary pump, a G7129A Agilent 1290 Infinity autosampler, a G1316A Agilent 1290 Infinity thermos tatted column compartment, and Agilent Jet Stream Electrospray ionization source (Agilent Technologies, CA, USA). The type of the chromatography (HILIC)-Z column was Agilent InfinityLab Poroshell 120 (2.1 mm × 100 mm × 2.7 μm, Agilent Technologies, CA, USA). The UPLC-MS/MS system was conducted with eluent A and eluent B. The flow rate of the former was 0.8 mL min−1 using 20 mM ammonium formate in water, whereas the latter was 20 mM ammonium formate in 9
:
1 acetonitrile/water (v/v). The gradient of eluent B was as follows: 0 min, 100%, 11.5 min, ramped to 70%, and 12 min, ramped to 100%. The column temperature was 30 °C, injection volume was 1 μL, and the run time was 15 min. The amino acids’ standard curves were converted into corresponding amino acid concentrations by an external standard method according to the instruction of the manufacturer (Sigma-Aldrich, St Louis, MO, USA).
Fatty acid profile analysis
The feces and colonic tissues samples were added with methanol
:
acetylchloride (8
:
1, v/v) and methyl undecacarbonate was added as the internal standard. Then, the mixture was homogenized and placed at 95 °C for 2 h. Hexane and 12% potassium carbonate solution were added to the mixture, respectively. After vortexing, the samples were centrifuged at 5000g for 3 min to collect the supernatant.
A gas chromatograph (Agilent 8890, CA, USA) equipped with an SP-2560 column (100 m × 0.25 mm × 0.20 μm; Supelco) was used to separate and detect fatty acids in the extracted samples. The temperature of the column oven was 140 °C for 5 min, then increased up to 180 °C by 12 °C min−1 for 6 min, increased up to 210 °C by 2.5 °C min−1 for 20 min, and increased up to 230 °C by 4 °C min−1 for 10 min; the detector and injector temperatures were 260 °C and 250 °C, respectively. The flow rate was 1 mL min−1 and the injection volume was 1 μL.
Short-chain fatty acid (SCFAs) profile analysis
The thawed feces were dissolved in distilled water and centrifuged at 10
000g at 4 °C for 10 min. The supernatant was added to 25% metaphosphoric acid solution (10
:
1, v/v) and held for 30 min at room temperature. Then, the mixture was centrifuged at 10
000g at 4 °C for 15 min. Finally, the solution was transferred into brown tubes and measured by an Agilent 8890 gas chromatograph (Agilent Technologies, Inc, Palo Alto, CA, USA) equipped with a capillary column (30 m × 0.25 mm × 0.25 μm; DB-FFAP, Agilent Technologies, USA).
Statistical analysis
The significant difference of body weights, DAI, and histological score of colons were performed by one-way analysis of variance (ANOVA), followed by Duncan test for multiple comparison (SPSS v22. IBM Corp). The data of cytokines, amino acids, fatty acids, and SCFAs were analyzed using unpaired Student's t-test (SPSS v22. IBM Corp). The PCA scores plot of the metabolome was used to reveal the presence of outliers outside the 95% significance level of Hotelling's T2 ellipse. The significantly different metabolites between the groups were determined by VIP > 1 and p < 0.05. All data were presented as the mean ± standard error of the mean (SEM) and drawn by GraphPad Prism 8.0 (GraphPad Software, Inc. San Diego, USA). p < 0.05 indicated significant difference.
Results
Effect of mEVs supplementation on cytokine concentrations
As expected, mEVs treatment significantly prevented weight loss (Fig. 1A) and DAI score (Fig. 1B) in DSS-induced colitis.21 Histological analysis found that mEVs significantly decreased the infiltration of inflammatory cells, mucosal injury, and overall histology score21 (Fig. 1C and D). Moreover, mEVs significantly decreased the concentrations of pro-inflammatory cytokines (IL-6 and IL-1β) and increased the level of anti-inflammatory cytokine IL-10 (p < 0.05) (Fig. 1E). The above results indicated that mEVs treatment significantly ameliorated DSS-induced colitis.
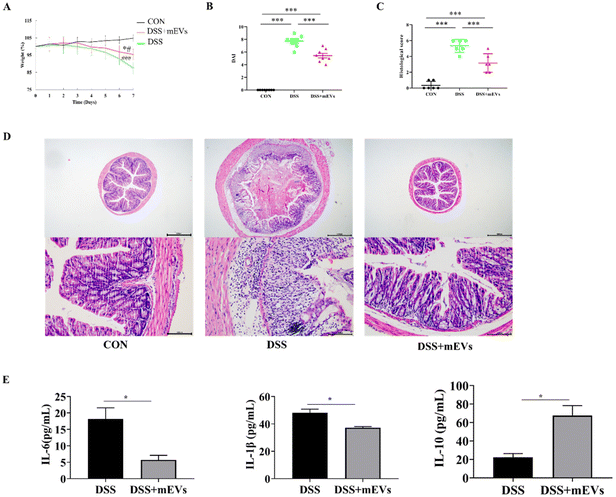 |
| Fig. 1 Bovine milk-derived (mEVs) treatment alleviated the clinical symptoms of DSS-induced colitis. (A) The body weight. (B) Disease activity index (DAI) assessment. (C) The histology score. (D) Hematoxylin and eosin (H&E)-stained analysis, scale bar = 500 μm (top) and 100 μm (bottom). (E) The concentrations of cytokines in the plasma. Data represent the mean ± SEM, n = 12, * p < 0.05, ** p < 0.01, *** p < 0.001. * p < 0.05 versus DSS group; # p < 0.05, ### p < 0.001 versus CON group. | |
Effect of mEVs supplementation on plasma metabolites
LC-MS technology was used to investigate the metabolite profiles in plasma samples. PCA presented obvious separation of metabolic features after oral mEVs in DSS-induced colitis (Fig. 2A). Heat map analysis found that mEVs feeding resulted in a distinct cluster of metabolites in mouse plasma (Fig. 2B). A total number of 192 metabolites were significantly changed in plasma samples, with 148 upregulated and 44 downregulated (VIP > 1, and p < 0.05) (Fig. 2C and Table S1†). Besides, the top 10 significantly increased metabolites in response to mEVs were Cer(d18:1/24:1(15Z)), PGP(18:0/18:0), oxitropium, Nb-palmitoyltryptamine, C17 sphinganine, pristanic acid, isopentenyladenosine-5′-diphosphate, nalbuphone, forasartan, and PC(P-15:0/0:0) (Table S1†). mEVs also improved the concentrations of certain phosphatidylcholine (PC), phosphatidic acid (PA), sphingomyelin (SM), and lysophospholipid (LysoPE), specifically PC(20:3(8Z,11Z,14Z)/20:4(5Z,8Z,11Z,14Z)), PC(P-15:0/0:0), PC(22:2(13Z,16Z)/22:2(13Z,16Z)), PC(22:5(4Z,7Z,10Z,13Z,16Z)/16:0), PA(22:4(7Z,10Z,13Z,16Z)/14:0), PA(16:0/22:2(13Z,16Z)), SM(d18:2(4E,14Z)/24:0), LysoPE(18:3(9Z,12Z,15Z)/0:0), and LysoPE(0:0/20:3(5Z,8Z,11Z)) (Table S1†). In addition, mEVs also significantly improved the level of other inflammation-related metabolites, such as gabapentin, β-lapachone, formononetin, umbelliferone, and noscapine (Table S1†).
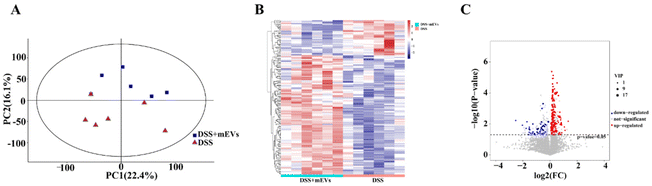 |
| Fig. 2 Bovine milk-derived (mEVs) altered metabolic profiling in DSS-induced colitis. (A) Principal component analysis (PCA) of metabolites in plasma samples from DSS and DSS + mEVs groups. (B) Heat map of significantly altered metabolites in plasma samples. (C) Volcano plots of differentially changed metabolites in plasma samples. n = 6. | |
As shown in Fig. 3A, at the superclass level, 192 metabolites could be categorized into more than 18 different super classes, and the predominant super classes included lipids and lipid-like molecules (33.85%), organic acids and derivatives (12.5%), organoheterocyclic compounds (8.85%), organic oxygen compounds (5.73%), benzenoids (5.73%), and phenylpropanoids and polyketides (3.13%). At the class level, fatty acyls (13.02%), carboxylic acids and derivatives (9.90%), prenol lipids (6.25%), organooxygen compounds (6.25%), and glycerophospholipids (6.25%) were the main components (Fig. 3B).
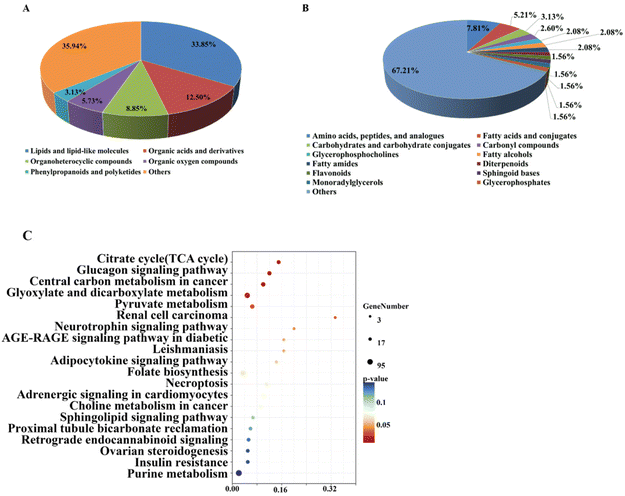 |
| Fig. 3 The pie chart of the classification of significantly different metabolites at superclass (A) and class (B) level. (C) Pathway enrichment analysis of the significantly altered metabolites. The x-axis represents the pathway impact, and the y-axis represents the pathway enrichment. | |
These phenotype-associated metabolites were significantly enriched in 10 KEGG pathways, including citrate cycle (TCA cycle), glucagon signaling pathway, central carbon metabolism in cancer, glyoxylate and dicarboxylate metabolism, pyruvate metabolism, renal cell carcinoma, neurotrophin signaling pathway, AGE-RAGE signaling pathway in diabetic complications, and leishmaniasis and adipocytokine signaling pathway (Fig. 3C). It is noteworthy that TCA cycle, glyoxylate and dicarboxylate metabolism, and pyruvate metabolism were the central hubs of nutrient metabolism.
Effect of mEVs supplementation on lipid metabolism
In the current study, we determined the fecal concentration of SCFAs, saturated fatty acids (SFAs), monounsaturated fatty acids (MUFAs), and polyunsaturated fatty acids (PUFAs) between the DSS group and DSS + mEVs group. Among SCFAs, mEVs significantly improved the levels of acetate and butyrate, with no apparent difference in the propionate level (Fig. 4A–C). In contrast, the tridecanoic acid (C13:0) content was significantly decreased in response to mEVs. The proportions of methyl cis-10-pentadecenoate (C15:1) and cis-11-eicosenoic acid (C20:1) were reduced in the mEVs group. Moreover, mEVs tended to decrease the level of α-linolenic acid (C18:3) and had no significant effect in other PUFAs concentrations.
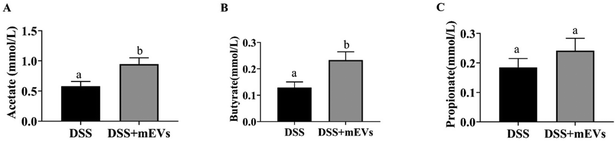 |
| Fig. 4 Bovine milk-derived (mEVs) treatment changed the production of SCFAs in the feces. (A) Acetate, (B) propionate, (C) butyrate. Mean values ± SEM are presented. Different letters in each figure panel indicate significant difference (p < 0.05). n = 6. | |
Table 1 presented the SFAs, MUFAs, and PUFAs levels in colonic tissue samples. Compared with the DSS group, myristoic acid (C14:0), palmitic acid (C16:0), and nervonic acid (C24:1) were significantly decreased in the colonic tissues with mEVs supplementation. Among PUFAs, the contents of linoleic acid (C18:2ω-6), eicosadienoic acid (C20:2), eicosapentaenoic acid (C20:5), and docosahexaenoic acid (C22:6) were significantly reduced in colonic tissues with mEVs supplementation.
Table 1 mEVs treatment altered the fatty acids profile in colitis mice
Items |
Metabolites |
Compound ID |
DSS |
DSS + mEVs |
SEM |
p
|
mEVs: milk-derived extracellular vesicles; DSS: dextran sodium sulfate; SEM = standard error of mean; SFA: saturated fatty acid; MUFA: monounsaturated fatty acid; PUFA: polyunsaturated fatty acids; compound ID: based on Human metabolome database (HMDB) database or Pubchem database; n = 7. |
Fecal sample (g per 100 g)
|
SFA
|
C13:0 |
Tridecanoic acid |
HMDB00910 |
0.442 |
0.336 |
0.4742 |
0.047 |
C14:0 |
Myristoic acid |
HMDB00806 |
0.038 |
0.010 |
0.0215 |
0.237 |
C15:0 |
Methyl pentadecanoate |
23518 |
0.012 |
0.005 |
0.0043 |
0.117 |
C16:0 |
Palmitic acid |
HMDB0000220 |
0.377 |
0.146 |
0.1653 |
0.201 |
C17:0 |
Methyl heptadecanoate |
LMFA07010473 |
0.005 |
0.004 |
0.0013 |
0.527 |
C18:0 |
Octadecanoic acid |
HMDB0010737 |
0.174 |
0.093 |
0.1038 |
0.452 |
C20:0 |
Eicosanoic acid |
HMDB0006740 |
0.005 |
0.004 |
0.0009 |
0.356 |
C22:0 |
Behenic acid |
HMDB0000944 |
0.005 |
0.006 |
0.0007 |
0.214 |
C24:0 |
Tetracosanoic acid |
HMDB0002003 |
0.004 |
0.005 |
0.0008 |
0.309 |
MUFA
|
C15:1 |
Methyl cis-10-pentadecenoate |
6112358 |
0.158 |
0.072 |
0.0374 |
0.041 |
C16:1 |
Palmitoleic acid |
HMDB0003229 |
0.004 |
0.003 |
0.0013 |
0.646 |
C20:1 |
cis-11-Eicosenoic acid |
HMDB0002231 |
0.015 |
0.008 |
0.0027 |
0.030 |
PUFA
|
C18:2ω-6 |
Linoleic acid |
HMDB0000673 |
0.086 |
0.074 |
0.0128 |
0.351 |
C18:3ω-3 |
α-Linolenic acid |
HMDB0001388 |
0.007 |
0.004 |
0.0013 |
0.078 |
C20:5ω-3 |
Eicosapentaenoic acid |
HMDB0001999 |
0.020 |
0.014 |
0.1190 |
0.626 |
C20:4ω-6 |
Arachidonic acid |
HMDB0001043 |
0.014 |
0.015 |
0.0042 |
0.866 |
C22:6ω-3 |
Docosahexaenoic acid |
HMDB0002183 |
0.007 |
0.007 |
0.0016 |
0.857 |
ω-6/ω-3 |
Omega-6/omega-3 ratio |
|
3.425 |
3.864 |
0.6520 |
0.273 |
Colonic tissue (g per 100 g)
|
SFA
|
C14:0 |
Myristoic acid |
HMDB0000806 |
0.025 |
0.015 |
0.0030 |
0.005 |
C16:0 |
Palmitic acid |
HMDB0000220 |
0.683 |
0.424 |
0.1113 |
0.045 |
C16:1 |
Palmitoleic acid |
HMDB0003229 |
0.066 |
0.040 |
0.0143 |
0.093 |
C18:0 |
Octadecanoic acid |
HMDB0000827 |
0.389 |
0.281 |
0.0822 |
0.246 |
C22:0 |
Behenic acid |
HMDB0000944 |
0.011 |
0.009 |
0.0018 |
0.258 |
MUFA
|
C18:1 |
Oleic acid |
HMDB0000207 |
0.289 |
0.248 |
0.0573 |
0.498 |
C20:1 |
cis-11-Eicosenoic acid |
HMDB0002231 |
0.015 |
0.010 |
0.0032 |
0.186 |
C22:1 |
Methyl cis-13-docosenoate |
87145151 |
0.028 |
0.031 |
0.0041 |
0.479 |
C24:1 |
Nervonic acid |
HMDB0002368 |
0.020 |
0.012 |
0.0022 |
0.005 |
PUFA
|
C18:2ω-6 |
Linoleic acid |
HMDB0000673 |
0.378 |
0.233 |
0.6544 |
0.047 |
C20:2ω-6 |
Eicosadienoic acid |
HMDB0005060 |
0.010 |
0.006 |
0.0009 |
0.004 |
C20:5ω-3 |
Eicosapentaenoic acid |
HMDB0001999 |
0.067 |
0.029 |
0.0117 |
0.017 |
C20:4ω-6 |
Arachidonic acid |
HMDB0001043 |
0.127 |
0.113 |
0.008 |
0.104 |
C22:6ω-3 |
Docosahexaenoic acid |
HMDB0002183 |
0.066 |
0.052 |
0.0051 |
0.025 |
ω-6/ω-3 |
Omega-6/omega-3 ratio |
|
4.326 |
4.487 |
0.3683 |
0.165 |
Effect of mEVs supplementation on amino acid metabolism
We also determined the levels of amino acids in colonic tissues and fecal samples between the two groups (Table 2). Compared with the DSS group, mEVs significantly increased the content of L-arginine and decreased L-valine in the fecal samples. However, fecal glycine, L-alanine, L-serine, L-proline, L-threonine, L-isoleucine, L-leucine, L-aspartate, L-glutamate, L-methionine, L-histidine, L-tyrosine, and L-cystine were not affected by mEVs. In the colon tissues, the level of glycine was significantly increased by oral gavage of mEVs, the levels of L-serine and L-glutamate were significantly decreased, while the other amino acids remained unchanged.
Table 2 mEVs treatment altered the amino acids profile in colitis mice
Items |
DSS |
DSS + mEVs |
SEM |
p
|
mEVs: milk-derived extracellular vesicles; DSS: dextran sodium sulfate; SEM = standard error of mean; n = 7. |
Fecal sample (mmol L
−1
)
|
Glycine |
0.051 |
0.061 |
0.0139 |
0.500 |
L-Alanine |
0.102 |
0.127 |
0.0329 |
0.464 |
L-Serine |
0.067 |
0.080 |
0.0225 |
0.578 |
L-Proline |
0.024 |
0.026 |
0.0042 |
0.773 |
L-Valine |
0.052 |
0.035 |
0.00741 |
0.042 |
L-Threonine |
0.268 |
0.313 |
0.0907 |
0.628 |
L-Isoleucine |
0.020 |
0.023 |
0.0041 |
0.478 |
L-Leucine |
0.040 |
0.067 |
0.0216 |
0.242 |
L-Aspartate |
0.028 |
0.030 |
0.0099 |
0.895 |
L-Glutamate |
0.043 |
0.057 |
0.0139 |
0.331 |
L-Methionine |
0.019 |
0.021 |
0.0028 |
0.516 |
L-Histidine |
0.016 |
0.017 |
0.0018 |
0.693 |
L-Arginine |
0.021 |
0.041 |
0.0083 |
0.034 |
L-Tyrosine |
0.035 |
0.036 |
0.0075 |
0.909 |
L-Cystine |
0.014 |
0.003 |
0.0099 |
0.284 |
Colonic tissue (mmol L
−1
)
|
Glycine |
0.008 |
0.017 |
0.0031 |
0.048 |
L-Alanine |
0.102 |
0.103 |
0.0033 |
0.936 |
L-Serine |
0.418 |
0.094 |
0.0716 |
0.002 |
L-Proline |
0.006 |
0.011 |
0.0032 |
0.176 |
L-Valine |
0.006 |
0.007 |
0.0010 |
0.108 |
L-Threonine |
0.003 |
0.004 |
0.0006 |
0.358 |
L-Isoleucine |
0.009 |
0.123 |
0.0023 |
0.215 |
L-Leucine |
0.009 |
0.011 |
0.0024 |
0.402 |
L-Aspartate |
0.011 |
0.015 |
0.0036 |
0.316 |
L-Lysine |
0.006 |
0.007 |
0.0017 |
0.320 |
L-Glutamate |
0.029 |
0.015 |
0.0059 |
0.039 |
L-Methionine |
0.007 |
0.009 |
0.0019 |
0.250 |
L-Histidine |
0.007 |
0.007 |
0.0004 |
0.284 |
L-Phenylalanine |
0.005 |
0.008 |
0.0030 |
0.467 |
L-Arginine |
0.004 |
0.007 |
0.0017 |
0.185 |
L-Tyrosine |
0.004 |
0.006 |
0.0009 |
0.126 |
L-Cystine |
0.031 |
0.037 |
0.0144 |
0.709 |
Discussion
Previous literature investigated that mEVs could avoid digestion treatment and are assimilated by intestinal cells through endocytosis, remaining bioavailable systemically.22 Meanwhile, mEVs can also exert direct effects on intestinal cells on absorption.22 Evidence also documented that the cargos in mEVs, in particular RNAs, were delivered to circulating immune cells in the host.23,24 In addition, mEVs also carry abundant proteins, wherein lactadherin plays an important role in serving intestinal epithelium health.25,26 In addition, mEVs have abundant lipids such as phosphatidylcholine (PC), phosphatidylserine (PS), phosphatidylethanolamine (PE), and sphingomyelin (SM).27 Some lipidic fractions of mEVs were found to be beneficial against intestinal inflammation.22 In the current study, we found the protective and therapeutic functions of mEVs on the animal model, as evidenced by the reduction in body weight loss, DAI score, and histology score. Further, higher concentration of anti-inflammatory cytokines (IL-10) and lower concentrations of pro-inflammatory (IL-6 and IL-1β) were determined in the plasma of mice offered mEVs, indicating that mEVs could alleviate colitis by inhibiting pro-inflammatory cytokine production and promote anti-inflammatory cytokine production.
Lipids and lipid-like molecules
Lipids play a critical role in the regulation of barrier permeability and could inhibit the development of colitis because it could change the cell membrane tight junction complex, fluidity, and gene expression of epithelial cells. However, these effects depend on the type of fatty acids. Sugiol is a diterpenoid and has been demonstrated to have anti-inflammatory effect by inhibiting NF-κB and MAPK signaling pathways and reducing the inflammatory markers such as TNF-α, IL-1β, and IL-6.28 β-Ionone, a precursor of carotenoids, could be converted to retinol and retinal.29 The anti-inflammatory effect of β-ionone was that it could suppress Akt and MAPK activation in LPS-induced inflammation.30 Oleic acid, a type of MUFA, might play an anti-inflammatory role due to its ability to inhibit the enzymes responsible for the formation of arachidonic acid, with strong pro-inflammatory properties.31 A recent study reported that serum oleic acid was decreased in the colitis mice compared to the control group.12 Therefore, the increased levels of sugiol, β-ionone, and oleic acids in plasma by mEVs is beneficial for suppressing inflammation.
In our study, mEVs supplementation promotes the utilization of n-3 PUFAs such as docosahexaenoic acid (DHA) and eicosapentaenoic acid (EPA) in the colonic tissue of colitis mice, which are beneficial to alleviate the inflammatory symptoms. DHA is the origin of the D-series resolvins, protectins, and maresins, while EPA constitutes the family of E-series resolvins.32 Recently, DHA and EPA were demonstrated to maintain intestinal barrier function by regulating the expressions of occludin, ZO-1, and MUC2.33 Besides, they also decrease the production of TNF-α, LTB4, and COX-2 by inhibiting NF-κB activity in a colitis model.34 The anti-inflammatory effect of DHA and EPA is associated with the activation of PPAR-γ agonists, which contributed to the alleviation of intestinal inflammation in IBD.33 Moreover, a study presented that the level of blood DHA is significantly decreased in the active inflammatory UC relative to UC remission.35 However, mEVs could improve the content of DHA in plasma, which is useful for colitis remission. In addition, mEVs promote the absorption of n-6 PUFAs such as eicosadienoic acid (EDA) in colonic tissues. EDA play an important role in pain initiation and anti-inflammatory role in IBD.36 Above all, mEVs promoted the intake of PUFAs, which was considered as a potential anti-inflammatory and health benefiting function.
Amino acids
Amino acids are required for intestinal growth and mucosal integrity, which are involved in intestinal inflammation, epithelial barrier, and energy homeostasis of the host. Compelling evidences indicate that amino acids play a crucial role in attenuating inflammation, oxidative stress, and the contents of pro-inflammatory cytokines.37,38 Firstly, a previous study reported that the blood level of glycine was decreased in DSS-induced colitis;39 however, mEVs could improve the plasma glycine level. Glycine has been reported to have anti-inflammatory effect in DSS-induced colitis,40 which may be related to promoting glutathione synthesis in cells, inactivating inflammatory cells in the lamina propria, and suppressing the secretion of pro-inflammatory cytokines.41
Secondly, colonic glutamate level was significantly decreased by mEVs, indicating that mEVs promoted the absorption of glutamate. Li et al. found that the microinjection of glutamate into hypothalamic paraventricular nucleus (PVN) facilitated cell proliferation, improved antioxidant levels, suppressed apoptosis, and the expression of pro-inflammatory cytokines (TNF-α and IL-1) in a colitis model.42 Moreover, tissue serine showed higher values in UC,39,43 and mEVs could reverse this symptom, with the higher uptake of serine. Accumulating studies elucidated that serine supplementation had beneficial effects on intestinal integrity, gut microbiota composition, and cell apoptosis inhibition in DSS-induced colitis.44,45
Finally, we also found several different fecal amino acids in response to mEVs. Valine belongs to branched chain amino acids, and the increased level in fecal samples was demonstrated to have positive association with the severity of IBD.15 Arginine was decreased in IBD patients,14 while mEVs increased its concentration in our results. Arginine, a semi-essential amino acid, is the precursor of nitric oxide (NO) and polyamines, which played a vital role in B-cell maturation, T-cell proliferation, and cytokine production.46 Besides, arginine supplementation effectively increased the abundance of anti-inflammatory gut microbes, decreased the pro-inflammatory factor expression, and protected the intestinal mucosa.47,48
According to previous studies, the increase and decrease of specific amino acids in blood, and increases in all tissues and feces might be owing to malabsorption in the intestinal or colonic leakage driven by inflammation.49,50 In the current study, mEVs could reverse the higher concentrations of certain amino acids in tissues and feces caused by inflammation, indicating that mEVs promoted the absorption of amino acids and were conducive to alleviating colitis.
Other metabolites
In this study, we also found that mEVs increased other inflammation-related metabolites in plasma. Gabapentin was able to reduce the number of mast cell count and degranulated mast cells in the colonic tissue, with the release of pro-inflammatory cytokines TNF-α and IL-1β.51 In addition, gabapentin could also activate the PPAR-γ receptor, which in turn inactivated NF-κB and consequently reduced the expression of inflammatory genes.51 β-Lapachone, a derivative of the naturally occurring element lapachol, plays an anti-inflammatory role by repressing the expression of iNOS, COX-2, and pro-inflammatory cytokines (IL-6, IL-1β, and TNF-α) in LPS-induced inflammation.52 Also, β-lapachone could inhibit LPS-stimulated protein tyrosine phosphorylation and NF-κB binding action in macrophages.52 Formononetin, an isoflavone, has anti-inflammatory effect by inhibiting the NLRP3 inflammasome pathway.53 Umbelliferone is a potential anti-inflammatory and antioxidant coumarin derivative.54 Moreover, umbelliferone might be suitable for therapeutic application in colitis because it could alleviate the symptoms through the regulation of TLR4/NF-κB-p65/iNOS and SIRT1/PPARγ signaling pathways.54 Noscapine is a plant-derived non-toxic alkaloid with anti-inflammatory properties.55 In summary, the increased levels of the above metabolites in the mEVs group might contribute to exerting anti-inflammatory function.
Conclusion
In conclusion, our results indicated that bovine mEVs shifted metabolomic profiles in the DSS-induced murine colitis, especially increased the levels of lipid anti-inflammatory metabolites, and reduced the levels of fecal amino acids, which could be a major driving force of mEVs in the remission of colitis. These findings provided novel insights into the effects of mEVs in suppressing inflammation by regulating the metabolism of nutrients, which might contribute to the development of new methods for the treatment of colitis.
Conflicts of interest
All authors have no conflicts of interest.
Acknowledgements
We extremely thank the staff of the Institute of Animal Science for their material and assistance in animal management. This project was funded by the National Key R&D Program of China (2019YFE0125600, 2021YFD2000800).
References
- D. C. Baumgart and S. R. Carding, Inflammatory bowel disease: cause and immunobiology, Lancet, 2007, 369, 1627–1640 CrossRef CAS PubMed
.
- S. C. Ng, H. Y. Shi, N. Hamidi, F. E. Underwood, W. Tang, E. I. Benchimol, R. Panaccione, S. Ghosh, J. C. Y. Wu, F. K. L. Chan, J. J. Y. Sung and G. G. Kaplan, Worldwide incidence and prevalence of inflammatory bowel disease in the 21st century: a systematic review of population-based studies, Lancet, 2017, 390, 2769–2778 CrossRef PubMed
.
- G. G. Kaplan and J. W. Windsor, The four epidemiological stages in the global evolution of inflammatory bowel disease, Nat. Rev. Gastroenterol. Hepatol., 2021, 18, 56–66 CrossRef PubMed
.
- S. Coward, F. Clement, E. I. Benchimol, C. N. Bernstein, J. A. Avina-Zubieta, A. Bitton, M. W. Carroll, G. Hazlewood, K. Jacobson, S. Jelinski, R. Deardon, J. L. Jones, M. E. Kuenzig, D. Leddin, K. A. McBrien, S. K. Murthy, G. C. Nguyen, A. R. Otley, R. Panaccione, A. Rezaie, G. Rosenfeld, J. N. Peña-Sánchez, H. Singh, L. E. Targownik and G. G. Kaplan, Past and Future Burden of Inflammatory Bowel Diseases Based on Modeling of Population-Based Data, Gastroenterology, 2019, 156, 1345–1353 CrossRef PubMed
.
- M. Zhao, L. Gönczi, P. L. Lakatos and J. Burisch, The Burden of Inflammatory Bowel Disease in Europe in 2020, J. Crohn's Colitis, 2021, 15, 1573–1587 CrossRef
.
- J. Z. Liu, S. van Sommeren, H. Huang, S. C. Ng, R. Alberts, A. Takahashi, S. Ripke, J. C. Lee, L. Jostins, T. Shah, S. Abedian, J. H. Cheon, J. Cho, N. E. Dayani, L. Franke, Y. Fuyuno, A. Hart, R. C. Juyal, G. Juyal, W. H. Kim, A. P. Morris, H. Poustchi, W. G. Newman, V. Midha, T. R. Orchard, H. Vahedi, A. Sood, J. Y. Sung, R. Malekzadeh, H. J. Westra, K. Yamazaki, S. K. Yang, C. International Multiple Sclerosis Genetics, I. B. D. G. C. International, J. C. Barrett, B. Z. Alizadeh, M. Parkes, T. Bk, M. J. Daly, M. Kubo, C. A. Anderson and R. K. Weersma, Association analyses identify 38 susceptibility loci for inflammatory bowel disease and highlight shared genetic risk across populations, Nat. Genet., 2015, 47, 979–986 CrossRef CAS PubMed
.
- R. J. Xavier and D. K. Podolsky, Unravelling the pathogenesis of inflammatory bowel disease, Nature, 2007, 448, 427–434 CrossRef CAS PubMed
.
- U. Daniluk, J. Daniluk, R. Kucharski, T. Kowalczyk, K. Pietrowska, P. Samczuk, A. Filimoniuk, A. Kretowski, D. Lebensztejn and M. Ciborowski, Untargeted Metabolomics and Inflammatory Markers Profiling in Children With Crohn's Disease and Ulcerative Colitis-A Preliminary Study, Inflammatory Bowel Dis., 2019, 25, 1120–1128 CrossRef PubMed
.
- M. Li, L. Yang, C. Mu, Y. Sun, Y. Gu, D. Chen, T. Liu and H. Cao, Gut microbial metabolome in inflammatory bowel disease: From association to therapeutic perspectives, Comput. Struct. Biotechnol. J., 2022, 20, 2402–2414 CrossRef PubMed
.
- M. Davenport, J. Poles, J. M. Leung, M. J. Wolff, W. M. Abidi, T. Ullman, L. Mayer, I. Cho and P. Loke, Metabolic alterations
to the mucosal microbiota in inflammatory bowel disease, Inflammatory Bowel Dis., 2014, 20, 723–731 CrossRef PubMed
.
- J. Diab, R. Al-Mahdi, S. Gouveia-Figueira, T. Hansen, E. Jensen, R. Goll, T. Moritz, J. Florholmen and G. Forsdahl, A Quantitative Analysis of Colonic Mucosal Oxylipins and Endocannabinoids in Treatment-Naïve and Deep Remission Ulcerative Colitis Patients and the Potential Link With Cytokine Gene Expression, Inflammatory Bowel Dis., 2019, 25, 490–497 CrossRef PubMed
.
- R. Wang, X. Gu, W. Dai, J. Ye, F. Lu, Y. Chai, G. Fan, F. J. Gonzalez, G. Duan and Y. Qi, A lipidomics investigation into the intervention of celastrol in experimental colitis, Mol. BioSyst., 2016, 12, 1436–1444 RSC
.
- K. Sugihara, T. L. Morhardt and N. Kamada, The Role of Dietary Nutrients in Inflammatory Bowel Disease, Front. Immunol., 2018, 9, 3183 CrossRef CAS PubMed
.
- K. Diederen, J. V. Li, G. E. Donachie, T. G. de Meij, D. R. de Waart, T. B. M. Hakvoort, A. Kindermann, J. Wagner, V. Auyeung, A. A. Te Velde, S. E. M. Heinsbroek, M. A. Benninga, J. Kinross, A. W. Walker, W. J. de Jonge and J. Seppen, Exclusive enteral nutrition mediates gut microbial and metabolic changes that are associated with remission in children with Crohn's disease, Sci. Rep., 2020, 10, 18879 CrossRef PubMed
.
- J. Z. Jagt, E. A. Struys, I. Ayada, A. Bakkali, E. E. W. Jansen, J. Claesen, J. E. van Limbergen, M. A. Benninga, N. K. H. de Boer and T. G. J. de Meij, Fecal Amino Acid Analysis in Newly Diagnosed Pediatric Inflammatory Bowel Disease: A Multicenter Case-Control Study, Inflammatory Bowel Dis., 2022, 28, 755–763 CrossRef PubMed
.
- L. Wang, X. Wang, Z. Shi, L. Shen, J. Zhang and J. Zhang, Bovine milk exosomes attenuate the alteration of purine metabolism and energy status in IEC-6 cells induced by hydrogen peroxide, Food Chem., 2021, 350, 129142 CrossRef CAS PubMed
.
- C. Du, K. Wang, Y. Zhao, X. Nan, R. Chen, S. Quan and B. Xiong, Supplementation with Milk-Derived Extracellular Vesicles Shapes the Gut Microbiota and Regulates the Transcriptomic Landscape in Experimental Colitis, Nutrients, 2022, 14, 1808 CrossRef CAS PubMed
.
- H. Izumi, N. Kosaka, T. Shimizu, K. Sekine, T. Ochiya and M. Takase, Bovine milk contains microRNA and messenger RNA that are stable under degradative conditions, J. Dairy Sci., 2012, 95, 4831–4841 CrossRef CAS PubMed
.
- C. Du, S. Quan, X. Nan, Y. Zhao, F. Shi, Q. Luo and B. Xiong, Effects of oral milk extracellular vesicles on the gut microbiome and serum metabolome in mice, Food Funct., 2021, 12, 10938–10949 RSC
.
- L. Tong, H. Hao, Z. Zhang, Y. Lv, X. Liang, Q. Liu, T. Liu, P. Gong, L. Zhang, F. Cao, G. Pastorin, C. N. Lee, X. Chen, J. W. Wang and H. Yi, Milk-derived extracellular vesicles alleviate ulcerative colitis by regulating the gut immunity and reshaping the gut microbiota, Theranostics, 2021, 11, 8570–8586 CrossRef CAS PubMed
.
- C. Du, Y. Zhao, K. Wang, X. Nan, R. Chen and B. Xiong, Effects of Milk-Derived Extracellular Vesicles on the Colonic Transcriptome and Proteome in Murine Model, Nutrients, 2022, 14, 3057 CrossRef CAS PubMed
.
- J. Garcia-Martinez, I. M. Perez-Castillo, R. Salto, J. M. Lopez-Pedrosa, R. Rueda and M. D. Giron, Beneficial Effects of Bovine Milk Exosomes in Metabolic Interorgan Cross-Talk, Nutrients, 2022, 14, 1442 CrossRef CAS PubMed
.
- L. Lin, G. Zhou, P. Chen, Y. Wang, J. Han, M. Chen, Y. He and S. Zhang, Which long noncoding RNAs and circular RNAs contribute to inflammatory bowel disease?, Cell Death Dis., 2020, 11, 456 CrossRef CAS PubMed
.
- S. Moein, M. Vaghari-Tabari, D. Qujeq, M. Majidinia, S. M. Nabavi and B. Yousefi, MiRNAs and inflammatory bowel disease: An interesting new story, J. Cell Physiol., 2019, 234, 3277–3293 CrossRef CAS PubMed
.
- K. M. Vaswani, H. Peiris, Y. Qin Koh, R. J. Hill, T. Harb, B. J. Arachchige, J. Logan, S. Reed, P. S. W. Davies and M. D. Mitchell, A complete proteomic profile of human and bovine milk exosomes by liquid chromatography mass spectrometry, Expert Rev. Proteomics, 2021, 18, 719–735 CrossRef CAS PubMed
.
- H. Shen, Y. Lei, X. He, D. Liu and Z. He, Role of lactadherin in intestinal barrier integrity in experimental neonatal necrotizing enterocolitis, J. Cell. Biochem., 2019, 120, 19509–19517 CrossRef CAS PubMed
.
- W. Chen, X. Chen, Y. Qian, X. Wang, Y. Zhou, X. Yan, B. Yu, S. Yao, Z. Yu, J. Zhu and S. Han, Lipidomic Profiling of Human Milk Derived Exosomes and Their Emerging Roles in the Prevention of Necrotizing Enterocolitis, Mol. Nutr. Food Res., 2021, 65, e2000845 CrossRef
.
- V. K. Bajpai, S. Sonwal, S. K. Hwang, S. Shukla, I. Khan, D. K. Dey, L. Chen, J. Simal-Gandara, J. Xiao, Y. S. Huh and Y. K. Han, Sugiol, a diterpenoid: Therapeutic actions and molecular pathways involved, Pharmacol. Res., 2021, 163, 105313 CrossRef CAS PubMed
.
- S. Baldermann, M. Kato, M. Kurosawa, Y. Kurobayashi, A. Fujita, P. Fleischmann and N. Watanabe, Functional characterization of a carotenoid cleavage dioxygenase 1 and its relation to the carotenoid accumulation and volatile emission during the floral development of Osmanthus fragrans Lour, J. Exp. Bot., 2010, 61, 2967–2977 CrossRef CAS PubMed
.
- C. H. Kang, R. G. Jayasooriya, Y. H. Choi, S. K. Moon, W. J. Kim and G. Y. Kim, beta-Ionone attenuates LPS-induced pro-inflammatory mediators such as NO, PGE2 and TNF-alpha in BV2 microglial cells via suppression of the NF-kappaB and MAPK pathway, Toxicol. in Vitro, 2013, 27, 782–787 CrossRef CAS PubMed
.
- R. P. D. Nascimento, A. Moya, A. Machado, M. V. Geraldi, P. Diez-Echave, T. Vezza, J. Galvez, C. B. B. Cazarin and M. R. Marostica Junior, Review on the potential application of non-phenolic compounds from native Latin American food byproducts in inflammatory bowel diseases, Food Res. Int., 2021, 139, 109796 CrossRef CAS PubMed
.
- N. Rohwer, C. Y. Chiu, D. Huang, C. Smyl, M. Rothe, K. M. Rund, N. Helge Schebb, H. Kuhn and K. H. Weylandt, Omega-3 fatty acids protect from colitis via an Alox15-derived eicosanoid, FASEB J., 2021, 35, e21491 CrossRef CAS PubMed
.
- C. Ma, R. Vasu and H. Zhang, The Role of Long-Chain Fatty Acids in Inflammatory Bowel Disease, Mediators Inflammation, 2019, 3, 8495913 Search PubMed
.
- A. Hassan, A. Ibrahim, K. Mbodji, M. Coëffier, F. Ziegler, F. Bounoure, J. M. Chardigny, M. Skiba, G. Savoye, P. Déchelotte and R. Marion-Letellier, An α-linolenic acid-rich formula reduces oxidative stress and inflammation by regulating NF-κB in rats with TNBS-induced colitis, J. Nutr., 2010, 140, 1714–1721 CrossRef CAS PubMed
.
- J. Kikut, A. Drozd, M. Mokrzycka, U. Grzybowska-Chlebowczyk, M. Zietek and M. Szczuko, Are EPA and DHA Derivatives Involved in IBD Remission?, J. Clin. Med., 2022, 11, 2388 CrossRef CAS PubMed
.
- S. Sitkin and J. Pokrotnieks, Alterations in Polyunsaturated Fatty Acid Metabolism and Reduced Serum Eicosadienoic Acid Level in Ulcerative Colitis: Is There a Place for Metabolomic Fatty Acid Biomarkers in IBD?, Dig. Dis Sci., 2018, 63, 2480–2481 CrossRef CAS PubMed
.
- X. Li, Z. H. Zhang, H. M. Zabed, J. Yun, G. Zhang and X. Qi, An Insight into the Roles of Dietary Tryptophan and Its Metabolites in Intestinal Inflammation and Inflammatory Bowel Disease, Mol. Nutr. Food Res., 2021, 65, e2000461 CrossRef PubMed
.
- Y. Liu, X. Wang and C. A. Hu, Therapeutic Potential of Amino Acids in Inflammatory Bowel Disease, Nutrients, 2017, 9, 920 CrossRef PubMed
.
- Y. Shiomi, S. Nishiumi, M. Ooi, N. Hatano, M. Shinohara, T. Yoshie, Y. Kondo, K. Furumatsu, H. Shiomi, H. Kutsumi, T. Azuma and M. Yoshida, GCMS-based metabolomic study in mice with colitis induced by dextran sulfate sodium, Inflammatory Bowel Dis., 2011, 17, 2261–2274 CrossRef PubMed
.
- Z. Dai, Z. Wu, S. Hang, W. Zhu and G. Wu, Amino acid metabolism in intestinal bacteria and its potential implications for mammalian reproduction, Mol. Hum. Reprod., 2015, 21, 389–409 CrossRef CAS
.
- Y. Zhang, D. Jiang, Y. Jin, H. Jia, Y. Yang, I. H. Kim, Z. Dai, J. Zhang, F. Ren and Z. Wu, Glycine Attenuates Citrobacter rodentium-Induced Colitis by Regulating ATF6-Mediated Endoplasmic Reticulum Stress in Mice, Mol. Nutr. Food Res., 2021, 65, e2001065 CrossRef PubMed
.
- T. T. Li, J. F. Zhang, S. J. Fei, S. P. Zhu, J. Z. Zhu, X. Qiao and Z. B. Liu, Glutamate microinjection into the hypothalamic paraventricular nucleus attenuates ulcerative colitis in rats, Acta Pharmacol. Sin., 2014, 35, 185–194 CrossRef CAS PubMed
.
- D. Xie, F. Li, D. Pang, S. Zhao, M. Zhang, Z. Ren, C. Geng, C. Wang, N. Wei and P. Jiang, Systematic Metabolic Profiling of Mice with Dextran Sulfate Sodium-Induced Colitis, J. Inflammation Res., 2021, 14, 2941–2953 CrossRef PubMed
.
- H. Zhang, R. Hua, B. Zhang, X. Zhang, H. Yang and X. Zhou, Serine Alleviates Dextran Sulfate Sodium-Induced Colitis and Regulates the Gut Microbiota in Mice, Front. Microbiol., 2018, 9, 3062 CrossRef PubMed
.
- X. Zhou, Y. Zhang, L. He, D. Wan, G. Liu, X. Wu and Y. Yin, Serine prevents LPS-induced intestinal inflammation and barrier damage via p53-dependent glutathione synthesis and AMPK activation, J. Funct. Foods, 2017, 39, 225–232 CrossRef CAS
.
- G. Wu, F. W. Bazer, T. A. Davis, S. W. Kim, P. Li, J. Marc Rhoads, M. Carey Satterfield, S. B. Smith, T. E. Spencer and Y. Yin, Arginine metabolism and nutrition in growth, health and disease, Amino Acids, 2009, 37, 153–168 CrossRef CAS PubMed
.
- J. Baier, M. Gansbauer, C. Giessler, H. Arnold, M. Muske, U. Schleicher, S. Lukassen, A. Ekici, M. Rauh, C. Daniel, A. Hartmann, B. Schmid, P. Tripal, K. Dettmer, P. J. Oefner, R. Atreya, S. Wirtz, C. Bogdan and J. Mattner, Arginase impedes the resolution of colitis by altering the microbiome and metabolome, J. Clin. Invest., 2020, 130, 5703–5720 CrossRef CAS PubMed
.
- M. E. Andrade, R. D. Santos, A. D. Soares, K. A. Costa, S. O. Fernandes, C. M. de Souza, G. D. Cassali, A. L. de Souza, A. M. Faria and V. N. Cardoso, Pretreatment and Treatment With L-Arginine Attenuate Weight Loss and Bacterial Translocation in Dextran Sulfate Sodium Colitis, JPEN, J. Parenter. Enteral Nutr., 2016, 40, 1131–1139 CrossRef CAS PubMed
.
- A. Eck, E. F. J. de Groot, T. G. J. de Meij, M. Welling, P. H. M. Savelkoul and A. E. Budding, Robust Microbiota-Based Diagnostics for Inflammatory Bowel Disease, J. Clin. Microbiol., 2017, 55, 1720–1732 CrossRef CAS PubMed
.
- J. T. Bjerrum, Y. Wang, F. Hao, M. Coskun, C. Ludwig, U. Günther and O. H. Nielsen, Metabonomics of human fecal extracts characterize ulcerative colitis, Crohn's disease and healthy individuals, Metabolomics, 2015, 11, 122–133 CrossRef CAS PubMed
.
- T. V. de Brito, G. J. D. Junior, J. S. da Cruz Junior, R. O. Silva, C. E. da Silva Monteiro, A. X. Franco, D. F. P. Vasconcelos, J. S. de Oliveira, D. V. da Silva Costa, T. B. Carneiro, A. S. Gomes Duarte, M. de Souza, P. M. G. Soares and A. Barbosa, Gabapentin attenuates intestinal inflammation: Role of PPAR-gamma receptor, Eur. J. Pharmacol., 2020, 873, 172974 CrossRef PubMed
.
- N. Mokarizadeh, P. Karimi, H. Kazemzadeh, N. Fathi Maroufi, S. Sadigh-Eteghad, S. Nikanfar and N. Rashtchizadeh, An evaluation on potential anti-inflammatory effects of beta-lapachone, Int. Immunopharmacol., 2020, 87, 106810 CrossRef CAS PubMed
.
- D. Wu, K. Wu, Q. Zhu, W. Xiao, Q. Shan, Z. Yan, J. Wu, B. Deng, Y. Xue, W. Gong, G. Lu and Y. Ding, Formononetin Administration Ameliorates Dextran Sulfate Sodium-Induced Acute Colitis by Inhibiting NLRP3 Inflammasome Signaling Pathway, Mediators Inflammation, 2018, 2018, 3048532 Search PubMed
.
- B. A. Abdel-Wahab, S. A. Alkahtani, A. A. Alqahtani and E. H. M. Hassanein, Umbelliferone ameliorates ulcerative colitis induced by acetic acid via modulation of TLR4/NF-kappaB-p65/iNOS and SIRT1/PPARgamma signaling pathways in rats, Environ. Sci. Pollut. Res. Int., 2022, 29, 37644–37659 CrossRef CAS PubMed
.
- K. Kaur, R. K. Sodhi, A. Katyal, R. Aneja, U. K. Jain, O. P. Katare and J. Madan, Wheat germ agglutinin anchored chitosan microspheres of reduced brominated derivative of noscapine ameliorated acute inflammation in experimental colitis, Colloids Surf., B, 2015, 132, 225–235 CrossRef CAS PubMed
.
|
This journal is © The Royal Society of Chemistry 2023 |
Click here to see how this site uses Cookies. View our privacy policy here.