DOI:
10.1039/D2FO02928F
(Paper)
Food Funct., 2023,
14, 2793-2806
Dietary intake of table olives exerts antihypertensive effects in association with changes in gut microbiota in spontaneously hypertensive rats†
Received
30th September 2022
, Accepted 24th February 2023
First published on 25th February 2023
Abstract
Arbequina table olive (AO) consumption lowers blood pressure (BP) in spontaneously hypertensive rats (SHR). This study evaluates whether dietary supplementation with AO induced changes in the gut microbiota that are consistent with the purported antihypertensive effects. Wistar-Kyoto rats (WKY-c) and SHR-c received water, while SHR-o were supplemented by gavage with AO (3.85 g kg−1) for 7 weeks. Faecal microbiota was analysed by 16S rRNA gene sequencing. SHR-c showed increased Firmicutes and decreased Bacteroidetes compared to WKY-c. AO supplementation in SHR-o decreased BP by approximately 19 mmHg, and reduced plasmatic concentrations of malondialdehyde and angiotensin II. Moreover, reshaped faecal microbiota associated with antihypertensive activity by lowering Peptoniphilus and increasing Akkermansia, Sutterella, Allobaculum, Ruminococcus, and Oscillospira. Also promoted the growth of probiotic strains of Lactobacillus and Bifidobacterium and modified the relationship of Lactobacillus with other microorganisms, from competitive to symbiotic. In SHR, AO promotes a microbiota profile compatible with the antihypertensive effects of this food.
1. Introduction
Hypertension is the main preventable risk factor for cardiovascular disease and premature death worldwide.1 Primary hypertension is induced by the interaction of non-modifiable genetic factors, which determine the risk of cardiovascular disease, with modifiable environmental factors, such as overweight and unhealthy lifestyles.1
An important element in the prevention of cardiovascular disease is the adherence to healthy dietary habits like those offered by the Mediterranean diet (MD). The MD has been widely studied, with strong evidence showing that it promotes cardiovascular health and prevents obesity and hypertension.2 The consumption of the core elements of the MD (fruits, virgin olive oil, cereals, vegetables, nuts, legumes, and fish) is associated with a lower risk of cardiovascular disease and lower blood pressure (BP).3
Extra virgin olive oil (EVOO) has anti-inflammatory, antioxidant, and vasodilator properties, which reduce the atherosclerotic burden.3,4 EVOO decreases BP in the spontaneously hypertensive rat (SHR) model5 and in individuals at a high risk of developing cardiovascular disease.6 In addition to oleic acid, olive oil contains polyphenols and pentacyclic triterpenes that have antioxidant, anti-inflammatory, and cardioprotective effects.4 Phenolic compounds from EVOO contribute to the protection of blood lipids from oxidative stress, as established by the Commission Regulation (EU) No. 432/2012 document.7
It is well known that during olive oil milling, only a minimal portion of the bioactive compounds is extracted along with the oil. The rest remains in the mill by-products, like olive pomace or olive pulp, which are used to prepare dietary supplements or ingredients for animal feed.8 These by-products have been studied as potential sources of bioactive compounds. For example, olive pomace powders have been shown to have gastrointestinal health benefits as they stimulate the production of short-chain fatty acids (SCFA) by the gut microbiota, which has well-known beneficial effects.9 The consumption of bread enriched with olive fibre has been observed to have beneficial effects on the host gut, increasing the abundance of probiotic bacteria such as Bifidobacteriaceae and Lactobacillales.10 However, there are only a few studies on the protective effects of table olive consumption on cardiovascular variables.
In recent years, interest has focused on the relationship between gut microbiota and the host health status. Since the gut microbiota produces active metabolites that are involved in several physiological processes, an altered microbiota may be implicated in the development of cardiometabolic diseases.11 Studies on animal models and humans have shown that the gut microbiota of hypertensive individuals has lower bacterial diversity and a different taxonomic composition compared to normotensive controls.12 Some studies also suggest a possible causal role of gut dysbiosis in the pathogenesis of hypertension.13
Recently, our group reported that dietary supplementation with Arbequina table olives (AO) for 7 weeks lowered BP in SHR from the second week until the end of the intervention.14 Therefore, in view of the role of the gut microbiota in the regulation of cardiovascular functions, the present study analysed differences in the faecal microbiota composition of hypertensive and normotensive rats and evaluated the hypothesis that dietary AO supplementation promotes the growth of bacteria involved in BP modulation in SHR.
2. Materials and methods
2.1. Table olives
Table olives of the Arbequina variety (AO), harvested in the 2016–2017 season and subjected to natural fermentation in brine, were obtained from Cooperativa del Camp (Maials, Lleida, Spain). The composition of AO (g per 100 g of destoned olives) consisted of 21.0 lipids, 1.6 proteins, 7.2 fibre, and 4.3 salt, with metabolizable energy of 211 kcal (868 kJ). The AO content of pentacyclic triterpenes and phenolic compounds was 3308 ± 195 mg kg−1 and 1048 ± 85 mg kg−1 of destoned olive (n = 5). The detailed content of bioactive compounds in AO is shown in Table S1.† AO was prepared as a homogeneous suspension of the edible part of the olive, at a dose of 3.85 g kg−1 of animal weight which is equivalent to the intake of 30 AO by a person weighing 60 kg, as previously described.14
2.2. Animals
The study was approved by the Animal Experimentation Ethics Committee of the Universitat de Barcelona (Ref. 105/17) and by the Generalitat de Catalunya (Ref. 9468), complying with the European Community Guidelines for the care and management of laboratory animals. Male spontaneously hypertensive rats (SHR) and normotensive Wistar-Kyoto (WKY) controls, all at the age of 11-week-old, were obtained from Envigo Laboratories (Huntingdon, United Kingdom). The animals were distributed into groups of two rats per cage and maintained under controlled conditions of temperature (22 ± 2 °C), humidity (50 ± 10%), and a 12-hour light–dark cycle. During the whole experiment, the rats were fed a standard diet (2014 Teklad Global 14%, Harlan, Barcelona, Spain) and water ad libitum.
2.3. Experimental design
At 14 weeks of age, the SHR group was randomly distributed into 2 groups, the untreated SHR (SHR-c n = 7) and the SHR supplemented with AO (SHR-o, n = 6). The third group was constituted of untreated WKY rats (WKY-c n = 8). During a period of 7 weeks, the control groups (WKY-c and SHR-c) received water by gavage at a volume of 10 mL kg−1 while the SHR-o had the corresponding dose of AO suspension.14 The body weight of the rats, as well as food and water consumption were measured at 14 and 21 weeks of age.
2.4. Blood pressure
Systolic (SBP), diastolic blood pressure (DBP) and heart rate (HR) were determined in WKY-c, SHR-c, and SHR-o rats at 14 and 21 weeks of age. Measurements were performed using a non-invasive automatic BP analyser for rodents (LE5001 Harvard Apparatus, Panlab, Barcelona, Spain) as previously described.14
2.5. Analysis of malondialdehyde, angiotensin II, IL6 and TNF-α in plasma
At the end of the experiments, overnight fasted rats were anaesthetized by intramuscular injection of ketamine (90 mg kg−1, Imalgene®, Merial, Lyon, France) and xylazine (10 mg kg−1, Rompun®, Bayer Hispania SL, Sant Joan Despí, Barcelona, Spain). Blood was collected from WKY-c, SHR-c, and SHR-o rats and transferred to EDTA-K3-coated tubes. Plasma samples were used to determine relevant biomarkers involved in the development of hypertension. Lipid peroxidation was assessed by measuring malondialdehyde (MDA) using the method described by Ohkawa et al.15 Enzyme-linked immunosorbent assay (ELISA) kits from FineTest (Wuhan, Hubei, China) were used to determine angiotensin II (ANG II) (Ref. ER1637) and tumour necrosis factor α (TNF-α) (Ref. ER1393) while the kit for interleukin 6 (IL6) (Ref. SEA079Ra) was provided by Cloud Clone Crop (Katy, TX, USA).
2.6. Collection of faecal samples
Stool samples were collected at 14 and 21 weeks of age in clean conditions. Faeces were collected directly into a sterile Eppendorf, frozen immediately in liquid N2, and stored at −80 °C until use.
2.7. DNA extraction and purification
Microbial DNA was extracted from stool samples using the QIAamp PowerFecal DNA kit (Mo Bio Laboratories, Carlsbad, CA, USA). DNA quantification was performed using the NanoDrop ND-1000 spectrophotometer (Thermo Fisher Scientific, Waltham, MA, USA), and the integrity of the extraction was verified by agarose gel electrophoresis.
2.8. Analysis of the 16S rRNA gene
The V3 and V4 hypervariable regions of the bacterial 16S rRNA gene were amplified by PCR using the specific primers PCR1_Forward (50 bp): 5′-TCGTCGGCAGCGTCAGATGTGTATAAGAGACAGCCTACGGGNGGCWGCAG-3′ and PCR1_Reverse (55 bp): 5′-GTCTCGTGGGCTCGGAGATGTGTATAAGAGACAGGACTACHVGGGTATCTAATCC-3′. Samples were sequenced using the Illumina MiSeq platform (Illumina, San Diego, CA, USA) at the Genomics and Bioinformatics Service of the Universitat Autònoma de Barcelona (Bellaterra, Barcelona, Spain). The analysis of the 16S rRNA gene was performed using the BaseSpace app 16S Metagenomics and the MiSeq Reporter software v 2.6 provided by the Illumina MiSeq platform. The raw paired-end reads were trimmed considering a Phred quality score equal to or greater than 30. The filtered sequences were analysed using the ClassifyReads algorithm, a high-performance implementation of the Ribosomal Database Project (RDP),16 with further sequence homology analysis by the RDP SeqMatch tool using the Greengenes database (v. 13.5) as a reference.
2.9. Statistical analysis
Microbiota analysis only included taxa with a percentage of reads higher than 0.001%. Alpha diversity was analysed using the Chao1 and Shannon indices and the number of species observed. Beta diversity was estimated based on Bray–Curtis dissimilarities, using analysis of similarities (ANOSIM) and permutational multivariate analysis of variance (PERMANOVA) with Bonferroni post-hoc test to determine differences between microbial communities. Both tests were performed in RStudio (R v. 4.2.2; R Core Team, Vienna, Austria) using the vegan package v. 2.4-6. Beta diversity was visualised using principal coordinate analysis from the 16s Metagenomics app.
The normality of data was assessed using the Shapiro–Wilk test. The faecal microbiota composition analysis was performed by two-way ANOVA for the factors strain and age (14 and 21 weeks) in WKY-c and SHR-c groups. The Benjamini-Hochberg procedure was used to control the false discovery rate (FDR 5%) in multiple comparisons. The effect of AO supplementation on the SHR-c and SHR-o faecal microbiota at 21 weeks of age was analysed using an independent Student t-test or the Mann–Whitney U test, according to data distribution. Correlation between faecal microbiota with BP, MDA and ANG II was performed using the Spearman rank correlation, and the correlation between microbiota genera was visualized by a network plot using Cytoscape v 3.9.1.17 The statistical analysis of body weight, feed intake, water consumption, BP, MDA, ANG II, IL6 and TNF-α was performed by one-way ANOVA considering treatment (water or AO) as the independent variable following of Bonferroni post-hoc test. Statistical significance was considered when p < 0.05. The sample size of the WKY-c (n = 8), SHR-c (n = 7), and SHR-o (n = 6) were established, considering an 80% power to detect a difference greater than or equal to 0.26 units for the Shannon index and changes of 16 mmHg or more in SBP, assuming in both cases a 5% significance level in a two-sided test. Statistical analysis was performed with GraphPad Prism v 8.0.2 (La Jolla, CA, USA).
3. Results
3.1. General characteristics
Table 1 shows the body weight as well as the food and water intake at the beginning and the end of the experiment, that is, at 14 and 21 weeks of age. No changes were found among groups in body weight gain and feed consumption. Conversely, the water consumption was higher in SHR-c and SHR-o than in the WKY-c rats (p < 0.05).
Table 1 General characteristics of WKY and SHR animals after the supplementation of Arbequina table olives (AO) at a dose of 3.85 g kg−1 or water during the experiment
|
WKY-c |
SHR-c |
SHR-o |
Results are presented as mean ± SEM in the WKY-c (n = 8), SHR-c (n = 7) and SHR-o (n = 6) groups. Data were analysed by one-way ANOVA followed by multiple comparison Bonferroni test. Means without a common letter differ, p < 0.05. SBP, systolic blood pressure; DBP, diastolic blood pressure; HR, heart rate. |
Body weight (g)
|
14-week-old |
270 ± 7a |
269 ± 4a |
272 ± 6a |
21-week-old |
347 ± 10a |
325 ± 4a |
323 ± 8a |
Feed intake (g day
−1
)
|
|
|
|
14-week-old |
18.2 ± 0.5a |
17.9 ± 0.2a |
18.4 ± 0.2a |
21-week-old |
18.0 ± 0.3a |
17.4 ± 0.2a |
16.8 ± 0.5a |
Water intake (mL day
−1
)
|
14-week-old |
21.8 ± 0.7a |
36.8 ± 2.3b |
37.4 ± 2.3b |
21-week-old |
21.1 ± 0.4a |
30.3 ± 1.6b |
29.9 ± 1.5b |
SBP (mmHg)
|
14-week-old |
147 ± 3a |
209 ± 3b |
209 ± 3b |
21-week-old |
152 ± 2a |
228 ± 4b |
208 ± 7c |
DBP (mmHg)
|
14-week-old |
98 ± 3a |
168 ± 4b |
166 ± 3b |
21-week-old |
109 ± 4a |
177 ± 5b |
158 ± 4c |
HR (bpm)
|
14-week-old |
395 ± 11a |
458 ± 6b |
458 ± 14b |
21-week-old |
404 ± 13a |
456 ± 8b |
446 ± 6b |
WKY-c rats gave measurements of SBP and DBP within the normotensive range, whereas SHR-c and SHR-o showed hypertensive values, both at 14 and 21 weeks of age (Table 1). The supplementation of AO for 7 weeks induced a decrease of 20 mmHg in SBP and 19 mmHg in DBP in the SHR-o compared to the SHR-c at the end of the experiment (Table 1). Moreover, the supplementation induced a non-significant decrease in heart rate (HR) of SHR-o with respect to SHR-c at the end of the 7 weeks of administration.
3.2. Malondialdehyde, angiotensin II, IL6 and TNF-α in plasma
Lipid peroxidation determined at 21 weeks of age was enhanced a 24% in plasma from SHR-c compared to WKY-c (p > 0.05). AO supplementation prevented the increase in the concentration of MDA in SHR-c yielding a decrease of 39% and a reduction of 20%, in WKY-c, as shown in Table 2 (p < 0.05). Plasma concentration of ANG II was higher in SHR-c compared with WKY-c (p < 0.05). However, ANG II concentration showed a reduction of 32% in rats administered with AO compared to SHR-c (p < 0.05) without reaching the values of WKY-c (Table 2). Quantification of IL6 and TNF-α showed similar plasma concentrations in control groups rats (WKY-c and SHR-c), and no differences were observed in rats supplemented with AO.
Table 2 Biomarkers of the development of hypertension in WKY and SHR animals after the supplementation of Arbequina table olives (AO) at a dose of 3.85 g kg−1 or water during the experiment
|
WKY-c |
SHR-c |
SHR-o |
Results are presented as mean ± SEM in the WKY-c (n = 8), SHR-c (n = 7) and SHR-o (n = 6) groups. TNF-α, tumour necrosis factor α; IL6, interleukin 6. Data were analysed by one-way ANOVA followed by multiple comparison Bonferroni test. Means without a common letter differ, p < 0.05. |
Malondialdehyde (μM)
|
21-week-old |
14.7 ± 1.8a |
19.3 ± 1.4a |
11.8 ± 0.5b |
Angiotensin II (pg mL
−1
)
|
21-week-old |
1277.5 ± 155.9a |
3704.8 ± 380.4b |
2517.0 ± 336.0c |
IL6 (pg mL
−1
)
|
21-week-old |
3.6 ± 0.02 |
3.7 ± 0.02 |
3.8 ± 0.03 |
TNF-α (pg mL
−1
)
|
21-week-old |
2.1 ± 0.2 |
2.9 ± 0.4 |
2.7 ± 0.3 |
3.3. Differences in faecal microbiota composition and age-related changes in WKY rats and SHR controls
After bacterial DNA sequencing, a total of 4
182
795 reads that passed quality filtering (PF) were generated, and each faecal sample produced an average of 154
918 ± 9041 PF reads. Similar alpha diversity (p > 0.05) was found for the WKY-c rats and SHR-c at 14 and 21 weeks of age (Fig. 1A). PCoA at the genus level also showed no differences, according to ANOSIM (R = 0.07; p = 0.134) and PERMANOVA (F = 1.41; p = 0.139), between the rat strains in beta diversity at both the ages examined (Fig. 1B).
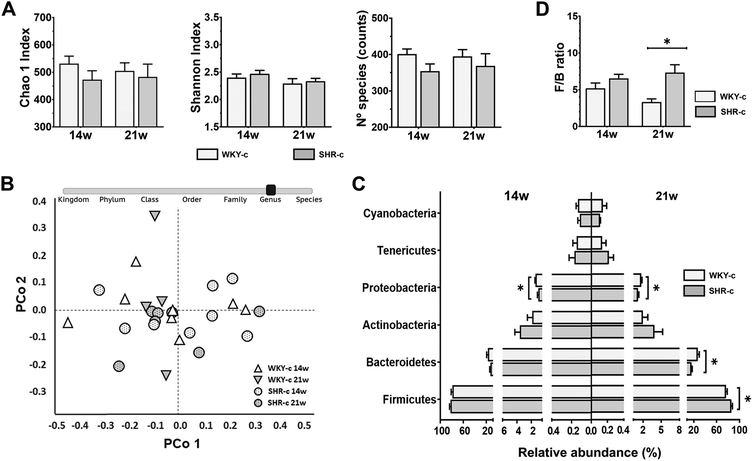 |
| Fig. 1 Differences between the faecal microbiota composition of Wistar-Kyoto (WKY-c) and spontaneously hypertensive rats (SHR-c) at 14 and 21 weeks of age. (A) Alpha diversity was evaluated with the Chao1 Index, the Shannon Index, and the number of species. (B) Beta diversity at the genus level was assessed by ANOSIM and PERMANOVA tests adjusted by Bonferroni post-hoc analysis and visualized by principal coordinate analysis (PCoA) plot. (C) Relative abundance at the phylum level; and (D) the Firmicutes/Bacteroidetes (F/B) ratio. Results are expressed as the mean ± SEM of the relative abundance in WKY-c (n = 8) and SHR-c (n = 7). Two-way ANOVA with Bonferroni post-hoc test was used to analyse alpha diversity, relative abundance at phylum level and F/B ratio between groups. *p < 0.05 indicate statistically significant differences between the strains of the same age. | |
Faecal microbiota composition was evaluated from the relative abundances of the different taxonomic levels (Fig. 1C). In both WKY-c rats and SHR-c, at 14 weeks of age, Firmicutes (WKY-14 weeks: 78.9% and SHR-14 weeks: 82.5%) was the main phylum, followed by Bacteroidetes (WKY-14 weeks: 17.1% and SHR-14 weeks: 12.3%), Actinobacteria (WKY-14 weeks: 1.89% and SHR-14 weeks: 3.60%), and Proteobacteria (WKY-14 weeks: 1.42% and SHR-14 weeks: 1.05%), with the remaining phyla accounting for less than 1% of the total faecal bacteria. No significant differences between strains were found at 14 weeks of age except for Proteobacteria, which were significantly increased in WKY-c rats (p = 0.024) (Fig. 1C) and remained higher at 21 weeks of age (WKY-21 weeks: 1.59% and SHR-21 weeks: 1.26% p = 0.016). The comparison of the faecal microbiota between WKY-c rats and SHR-c at 21 weeks of age (Fig. 1C) showed an increase in the abundance of Firmicutes in SHR-c (WKY-21 weeks: 72.5% and SHR-21 weeks: 82.0% p = 0.014) and a higher abundance of Bacteroidetes in WKY-c rats (WKY-21 weeks: 23.1% and SHR-21 weeks: 12.2% p = 0.003). At the phylum level, there was an increase in the Firmicutes to Bacteroidetes (F/B) ratio in SHR-c, which was 2-fold higher than that of WKY-c rats at 21 weeks of age (p = 0.026) (Fig. 1D).
Lactobacillus, Blautia, Ruminococcus, and Turicibacter were the most abundant genera in both WKY rats and SHR throughout the study period, without differences between groups (Fig. 2). No differences in the relative abundances of the different genera were found between strains at 14 weeks of age, except for Allobaculum which was superior (p < 0.05) in SHR-c with respect to WKY-c but showed no differences at 21 weeks of age (Fig. 2). However, important differences were found at 21 weeks of age, where the relative abundances of Phascolarctobacterium, Parabacteroides, Prevotella, Desulfovibrio, Sutterella, and Akkermansia were higher (p < 0.05) in WKY-c rats with respect to SHR-c.
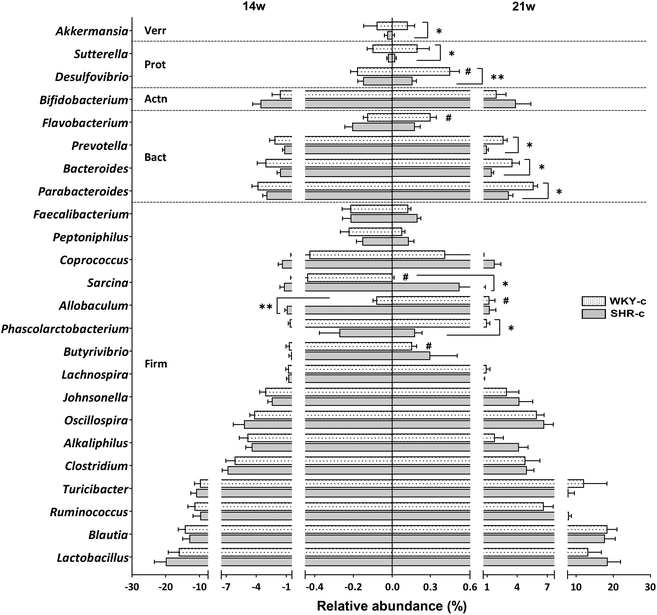 |
| Fig. 2 Relative abundance at the genus level of faecal microbiota of SHR-c and WKY-c rats at 14 and 21 weeks of age. The graph depicts the phyla Firmicutes (Firm), Bacteroidetes (Bact), Actinobacteria (Actn), Proteobacteria (Prot), and Verrucomicrobia (Verr). Results are expressed as the mean ± SEM of the relative abundance in WKY-c (n = 8) and SHR-c (n = 7). Two-way ANOVA with Bonferroni post-hoc test was used for comparison between groups. *p < 0.05 and **p < 0.01 indicate statistically significant differences between the strains of the same age; #p < 0.05 indicate the differences due to age within the same rat strain. | |
3.4. Key bacteria related to blood pressure
The association between the relative abundance of faecal microbiota and BP in WKY-c and SHR-c at 21 weeks of age was estimated by the analysis of Spearman rank correlation (Fig. 3). A direct association between the relative abundance of the phylum Firmicutes, including the Sarcina genus and the Lactobacillus acidophilus species were established with SBP and DBP (p < 0.05). Although this positive association was also set up for the genera Alkaliphilus and Peptoniphilus, the correlation was only significant with DBP (p < 0.05).
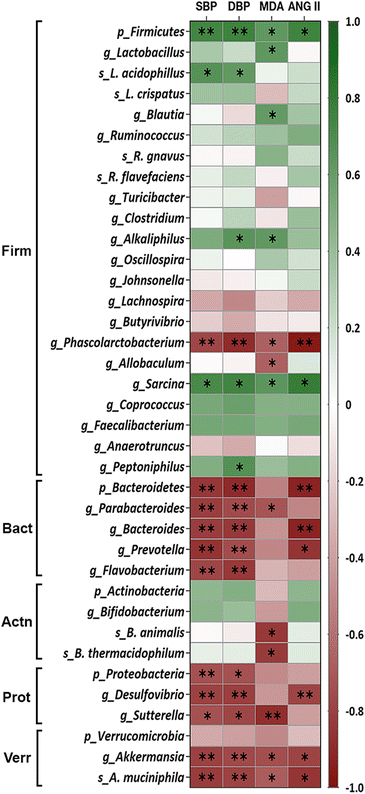 |
| Fig. 3 Heatmap of the Spearman rank correlation between the relative abundance of the bacterial faecal microbiota of SHR-c and WKY-c rats and systolic blood pressure (SBP), diastolic blood pressure (DBP), malondialdehyde (MDA) as well as angiotensin II (ANG II) at 21 weeks of age. The bacteria are grouped by phyla Firmicutes (Firm), Bacteroidetes (Bact), Actinobacteria (Actn), Proteobacteria (Prot), and Verrucomicrobia (Verr). Colours range from red (negative correlation) to green (positive correlation). *p < 0.05 and **p < 0.01 indicate statistically significant correlations. | |
On the other hand, an inverse significant association (p < 0.05) between the relative abundance of faecal microbiota was settled for the phylum Bacteroidetes, including Parabacteroides, Bacteroides, Prevotella, and Flavobacterium genera, the phylum Proteobacteria including the genera Desulfovibrio and Sutterella and SPB and DPB. Although the phylum Verrucomicrobia yielded a non-significant correlation with BP (p > 0.05), this inverse association was significant (p < 0.05) for the Akkermansia genus and Akkermansia muciniphila species (Fig. 3). Noteworthy that from the Firmicutes phylum, only the Phascolarctobacterium genus showed a negative relationship with BP (p < 0.05).
Spearman rank correlation analysis was also performed to estimate the association between gut microbiota and plasma concentrations of MDA and ANG II in hypertensive and normotensive rats. Fig. 3 shows that the phylum Firmicutes, along with the genera Lactobacillus, Blautia, Alkaliphilus, and Sarcina showed a direct association with MDA (p < 0.05); whereas only the phylum Firmicutes and Sarcina were correlated directly with ANG II (p < 0.05). Conversely, the genera Phascolarctobacterium, Allobaculum, Parabacteroides, Sutterella, and Akkermansia, along with the species Bifidobacterium animalis, Bifidobacterium thermacidophilum, and Akkermansia muciniphila were inversely correlated with lower values of lipid peroxidation (p < 0.05). While the phylum Bacteroidetes and the genera Phascolarctobacterium, Bacteroides, Prevotella, Desulfovibrio and Akkermansia showed an inverse association with ANG II (p < 0.05).
3.5. Effect of AO consumption on SHR faecal microbiota
The effect of AO on the diversity and abundance of SHR-c and SHR-o faecal microbiota was studied from a total of 1
950
997 PF reads with an average of 150
077 ± 15
909 PF reads per sample. Daily administration of AO for seven weeks did not affect the alpha diversity (p > 0.05) (Fig. 4A) and beta diversity of the microbial communities of SHR (ANOSIM: R = 0.17, p = 0.083 and PERMANOVA: F = 2.47, p = 0.077) (Fig. 4B). At 21 weeks of age, the main phyla, Firmicutes (SHR-c: 82.0% and SHR-o: 80.4%) and Bacteroidetes (SHR-c: 12.2% and SHR-o: 11.7%) showed similar abundances in both the untreated and treated groups, whereas only Actinobacteria (SHR-c: 3.09% and SHR-o: 5.99% p = 0.047) was significantly increased in the AO supplemented group (Fig. 4C). Moreover, AO intake did not modify the F/B ratio (Fig. 4D).
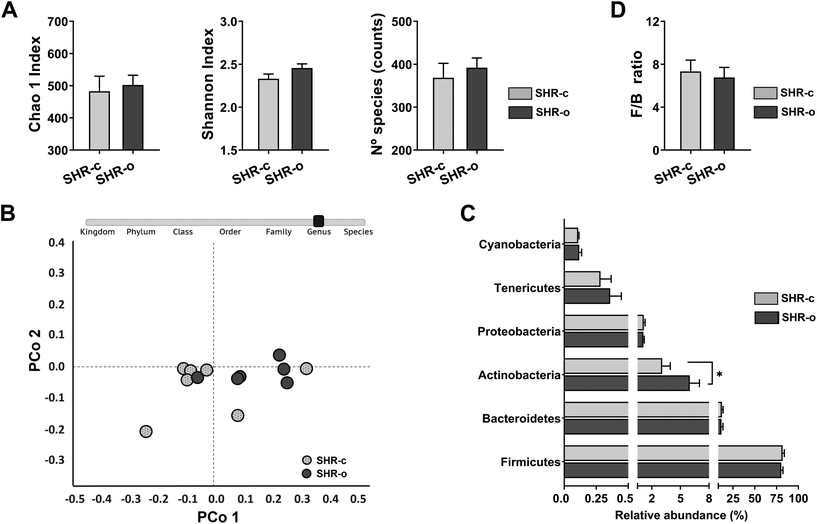 |
| Fig. 4 Effect of the daily intake of Arbequina table olives (AO) during 7 weeks in SHR. (A) Alpha diversity was evaluated with the Chao1 Index, the Shannon Index, and the number of species. (B) Beta diversity at the genus level was assessed using ANOSIM and PERMANOVA tests and visualized using a principal coordinate analysis (PCoA) plot. (C) Relative abundance at the phylum level; and (D) the Firmicutes/Bacteroidetes (F/B) ratio. Data are expressed as mean ± SEM, and differences in alpha diversity, relative abundance and F/B ratio between SHR-c (n = 7) and SHR-o (n = 6) were analysed by t-Student or the Mann–Whitney U test. | |
At the genus level, the most remarkable effect of AO consumption was the dramatic increase in the treated group of the growth of Allobaculum (SHR-c: 1.25% and SHR-o: 3.54% p = 0.031), Sutterella (SHR-c: 0.03% and SHR-o: 0.12% p = 0.038) and Akkermansia (SHR-c: 0.001% and SHR-o: 0.016% p = 0.013) (Fig. 5A). Moreover, AO elicited in the treated group a reduction in the growth of Peptoniphilus (SHR-c: 0.15% and SHR-o: 0.06% p = 0.017), Blautia (p = 0.049), Oscillospira (p = 0.048), and Ruminococcus (p = 0.049) (Fig. 5A).
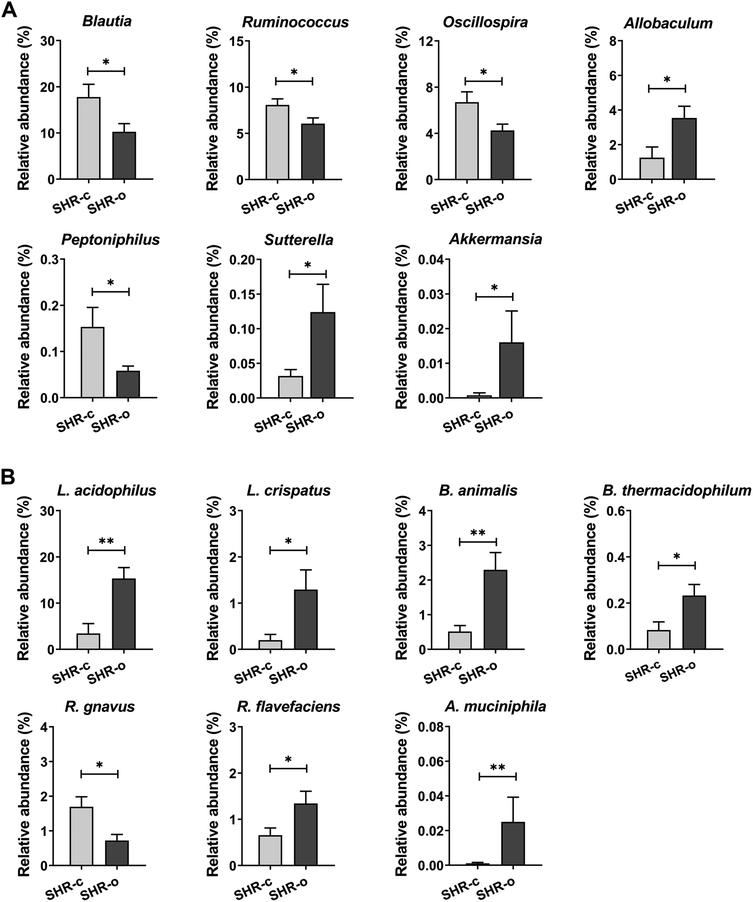 |
| Fig. 5 Effect of the daily consumption of Arbequina table olives (AO) for 49 days on (A) relative abundance at the genus level; and (B) species, in SHR faecal microbiota. Data are expressed as the mean ± SEM of the relative abundance and differences between SHR-c (n = 7) and SHR-o (n = 6) were analysed by t-Student or the Mann–Whitney U test. *p < 0.05 and **p < 0.01 indicate statistically significant differences. | |
Supplementation with AO also increased the abundance of Akkermansia muciniphila (p = 0.019), Lactobacillus acidophilus (p = 0.003), Lactobacillus crispatus (p = 0.008), Bifidobacterium animalis (p = 0.014), Bifidobacterium thermacidophilum (p = 0.028), and Ruminococcus flavefaciens (p = 0.041), but reduced the abundance of Ruminococcus gnavus (p = 0.019) (Fig. 5B).
3.6. Faecal microbiota co-occurrence networks
To evaluate interactions between bacterial taxa in the faecal microbiota of each group, we used Spearman rank correlation to create co-occurrence networks, where only significant correlations were considered (rho > 0.6 and p < 0.05). Positive correlations indicate cooperative or interdependent relationships between taxa, while negative correlations suggest a competitive relationship. The WKY-c group microbial network consists of 21 nodes (genera) and 53 edges (33 positive correlations and 20 negative correlations), where the mean number of relationships between bacterial taxa (degree) was 5.05, and the genera with the highest number of relationships were Coprococcus (degree 8), Bifidobacterium, and Bacteroides (degree 7). The SHR-c group network consisted of 23 nodes and 41 edges (16 positive and 25 negative correlations), where the mean number of relationships between taxa (3.56) was lower than that observed in WKY-c, and the most related genus was Oscillospira (degree 6), with a predominance of competitive relationships between bacterial genera. In the SHR-o group, after the supplementation with AO, the microbial network included 23 nodes and 52 edges (31 positive and 21 negative correlations), with an increase in the mean number of relationships between bacteria (4.52). Noteworthy that AO supplementation favoured the presence of Akkermansia within the network (degree 8) as well as the establishment of a higher number of positive correlations between taxa (Fig. 6).
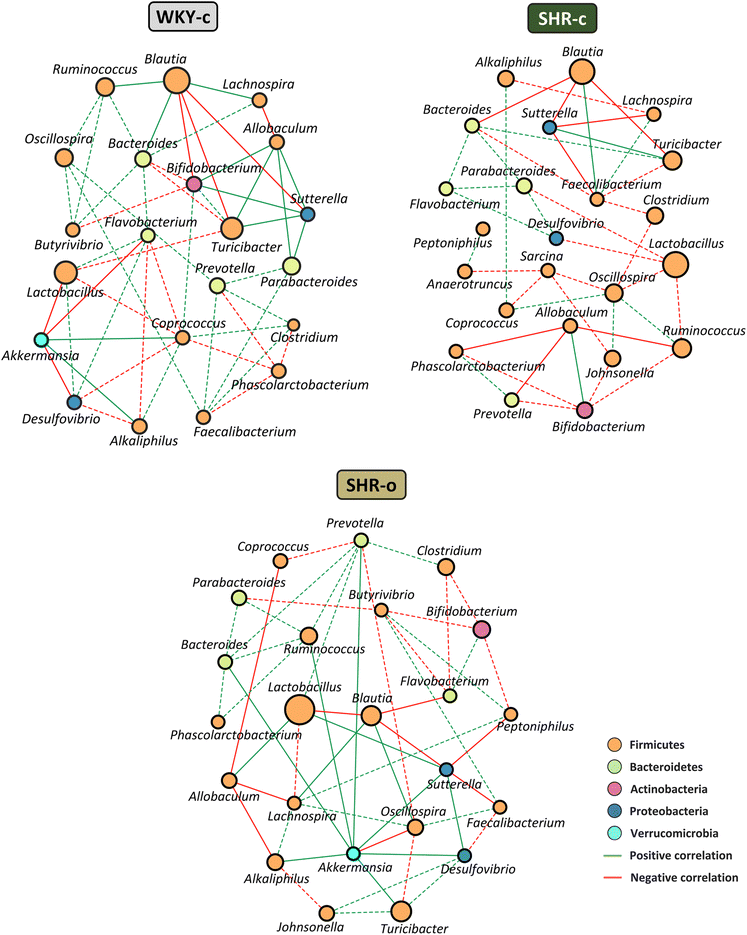 |
| Fig. 6 Co-occurrence network plots of the Spearman rank correlation among key genera. Faecal microbiota was analysed in WKY-c and SHR-c that were orally administered with water for 7 weeks as well as SHR-o supplemented by gavage with Arbequina table olives (AO) during the same experimental period. Genera are linked when the correlation is significant (p < 0.05). Node size indicates relative abundance. | |
4. Discussion
In a previous study, we found that the dietary supplementation with AO had anti-hypertensive effects in SHR but did not affect the BP in normotensive WKY rats.14 Recently, the development of cardiometabolic pathologies, including hypertension has been associated with changes in the microbiota.11,13 The mechanisms by which gut dysbiosis affects cardiovascular homeostasis may involve an imbalance in the production of the microbiota-derived metabolites, alteration of the immune system, increased sympathetic nervous system activity, alterations in gut barrier integrity, and intestinal inflammation.13 In addition, intestinal microbiota produces bioactive compounds that may have hypertensive or anti-hypertensive effects.13 Therefore, we have compared the profile of the microbiota of WKY-c with that of SHR-c to identify the species, genera, or phyla that correlate with BP. Furthermore, we have analysed the changes in the microbiota composition induced by the supplementation with AO in SHR.
The microbiota of SHR-c and WKY-c rats showed similar alpha and beta diversities at 14 and 21 weeks of age in agreement with the findings of Abboud et al.18 and Guo et al.19 in the same rat strains. Differences in species richness and diversity in SHR take place from 25 weeks of age,20,21 suggesting that the loss of diversity in the microbiota of hypertensive rats may occur in older animals than those used in the present study. At the phylum level, the faecal microbiota composition of WKY-c and SHR-c were similar at 14 weeks of age. However, at 21 weeks of age, the microbiota of SHR-c differs from that of age-matched WKY animals with a higher abundance of Firmicutes in SHR-c compared to WKY-c rats, a finding consistent with previous studies that described Firmicutes expansion as a characteristic of SHR gut dysbiosis.12
Given the differences in faecal microbiota composition observed between WKY-c and SHR-c at 21 weeks of age, a Spearman correlation analysis was conducted between the relative abundance of microorganisms and the measurements of BP. In the phylum Firmicutes, the genera Sarcina, Alkaliphilus, and Peptoniphilus as well as the species Lactobacillus acidophilus, exhibited a direct association with BP since their relative abundance increased in hypertensive animals. Conversely, a lower abundance of Phascolarctobacterium in SHR-c than in WKY-c was inversely associated with SBP and DBP, which is consistent with results reported by Guo et al.19 Similar results were found in hypertensive individuals and in patients with coronary artery disease, showing a lower abundance of Phascolarctobacterium than in healthy populations.22
Reduced abundance of the phylum Bacteroidetes has been described as a characteristic of the gut microbiota associated with hypertension.12 Our results show that the lower relative abundance of four genera of this phylum, namely Parabacteroides, Bacteroides, Prevotella, and Flavobacterium, was correlated with hypertension. This inverse association between Parabacteroides and arterial hypertension confirms previous reports.24 Moreover, the lower abundance of Parabacteroides, Bacteroides, and Flavobacterium was described in the gut microbiota of hypertensive rats.12,23 Other taxa found in less abundance in SHR-c with respect to WKY-c, and inversely associated with BP, were the phylum Proteobacteria along with the genera Desulfovibrio and Sutterella. From the phylum Verrucomicrobia an inverse correlation was found for the genera Akkermansia and the specie Akkermansia muciniphila. Diverging results have been reported for Desulfovibrio, since its abundance was decreased in 9-month-old SHR compared to WKY controls,23 and increased in rats transplanted with microbiota from spontaneously hypertensive stroke-prone rats24 as well as in hypertensive individuals.25 The inverse association of Sutterella with BP has also been established in SHR.19 This genus was also found to be reduced in hypertensive patients.26 Furthermore, a high relative abundance of Bifidobacterium animalis in Wistar rats has been associated with normotension.27 The drop in Akkermansia observed in SHR-c may also correlate with hypertension, as previously observed by Guo et al.19 and Robles-Vera et al.12 in the same strain.
Therefore, the analysis of faecal microbiota allowed the identification of taxa in SHR-c associated with high BP. Sixteen taxa were estimated to closely correlate with BP in rats, six of them showed a direct association with BP whereas ten exhibited an inverse relationship. Once the microbiota components associated with BP were identified, we conducted the second part of the study aimed at evaluating the effect of AO supplementation on SHR. We first confirmed the results of Franco-Ávila et al.14 showing that AO supplementation lowers BP in SHR, with a reduction of 20 mmHg of the SBP and 19 mmHg of the DBP. The dose of AO chosen (3.85 g kg−1) is equivalent to human consumption of 30-small-sized Arbequina olives which is about double the daily intake recommended by the MD pyramid.28 The dose of AO used did not affect body weight, probably because AO has a low-calorie density (211 kcal in 100 g of edible portion) which represent approximately an additional 5% of the daily energy intake in SHR-o with respect to SHR-c.
Some components of table olives are known to affect the composition of the gut microbiota. For example, oleic acid promotes the biodiversity of intestinal bacteria29 and stimulates the proliferation of species that produce SCFAs, which have anti-inflammatory activity and a role in the reduction of total cholesterol.30 In addition to oleic acid, the AO that was administered in our study contained approximately 1 g of polyphenols and 3.3 g of pentacyclic triterpenes per kg of the edible part of the olive that could influence the gut microbiota composition of SHR-o. In this sense, table olive polyphenols can act as prebiotics, inhibiting the growth of pathogenic bacteria such as Escherichia coli, and stimulating probiotic Bifidobacteria.30 Most of the ingested polyphenols are not absorbed in the small intestine and enter the large intestine, where they promote the growth of beneficial bacteria.31 Polyphenols can also be converted into active metabolites which may exert postbiotic effects.31 Similar properties have been described for pentacyclic triterpenes when interacting with the intestinal microbiota.32
In our results, the AO supplementation reduced the abundance of Peptoniphilus and increased Akkermansia (A. muciniphila) and Sutterella, which are changes that have been suggested to ameliorate hypertension.19,27,33 Concerning Akkermansia, this genus has been proposed as a biomarker of gut microbiota dysbiosis and some studies have reported an association between increased abundance and reduced prevalence of hypertension, obesity, and type-2 diabetes.33 In hypertensive rat models, the supplementation with quinoa19 or treatment with minocycline34 lowered BP which was accompanied by a higher abundance of Akkermansia compared to the control animals. In addition, this genus has been demonstrated to be influenced by dietary components, such as the polyphenol quercetin that was able to increase Akkermansia in Wistar rats consuming a high-fat sucrose diet.35Sutterella was also incremented after the supplementation with wasabi thus preventing the development of hypertension in a model of obesity and metabolic syndrome in Wistar rat.27 In accordance with our study, these authors also found an increase in the abundance of Allobaculum,27 an SCFA-producing genus.36Allobaculum is also associated with a reduction of BP in SHR19,20,27 and has been described to mediate the hypotensive effects of berberine.37 Furthermore, Allobaculum was associated with the increment of the expression of tight-junction proteins (which regulate epithelial permeability) in the large intestine38 and negatively correlated with proinflammatory cytokines present in the blood.39
We also observed that AO supplementation reduced the faecal abundance of Ruminococcus and Oscillospira, both related to the production of trimethylamine, involved in the development of atherosclerosis.40,41 The abundance of Ruminococcus is positively correlated with SBP27 and atrial fibrillation.42 On the other hand, the abundance of Oscillospira is positively correlated with hypertension, as shown in a Wistar rat model of obesity and metabolic syndrome27 and in the spontaneously hypertensive heart failure rat model.23 These results suggest that the effect of AO supplementation in reducing the abundances of Ruminococcus and Oscillospira may contribute to the prevention of cardiovascular disease.
AO promoted the growth of probiotic strains of Lactobacillus and Bifidobacterium. Its effects on increasing the abundance of Lactobacillus acidophilus may be relevant because, as shown in a clinical trial with elderly patients, this species was related to a reduction in BP and the restoration of plasma concentrations of total cholesterol, triglycerides, LDL, and HDL.43 In SHR, Hidalgo et al.29 showed that olive oil supplementation lowered systolic BP, an effect that correlated well with a higher abundance of Lactobacillus in the gut microbiota. Pentacyclic triterpenes from Olea europaea L. also promote the growth of Lactobacillus44 therefore, an effect of these bioactive compounds cannot be excluded. In our study, the increased abundance of Lactobacillus acidophilus was paralleled by an increase in Allobaculum, as described by Mendes et al.45
AO supplementation increased the abundance of probiotic Bifidobacterium, an effect also observed in animals supplemented with olive oil.46 The genus Bifidobacterium has also been associated with protective effects against hypertension in SHR.27 AO increased the abundance of Bifidobacterium animalis, which may be relevant because it upregulates the release of anti-inflammatory cytokines in the human intestinal HT-29 cell line.47 In addition, Bifidobacterium animalis can promote acetate production and regulate the Gpr43 receptor involved in BP regulation.48
The co-occurrence network has allowed us to study the interactions between microorganisms within the microbial community of each group, and to identify the number of relationships that a taxon establishes within the community, independently of its abundance.49 Genera with similar abundance have been observed in WKY-c and SHR-c, although the interaction between taxons was different. In SHR-c, bacteria belonging to the phylum Firmicutes, namely, Coprococcus, Oscillospira, Ruminococcus, Blautia, Lactobacillus, Allobaculum, and Alkaliphilus have fewer connections with other bacteria and mainly establish competitive relationships. In addition, bacteria from the phylum Bacteroidetes associated with normal BP values, show a lower number of interactions within the SHR-c microbiota. Likewise, it was observed that Sutterella, also associated with a normotensive state, establishes cooperative relationships in WKY-c, while in SHR-c its role is mainly competitive. AO supplementation for 7 weeks has generated changes in the interactions of the SHR-o microbiota. An increase in the number of bacteria with significant participation within the community as well as the cooperative relationships between taxa was found. On the other hand, in addition to changes in the relative abundance of some genera, AO supplementation has modified the relationship of some bacteria. For example, in the SHR-o group, Lactobacillus shows symbiotic relationships, while in SHR-c their interactions were clearly competitive. In addition to increasing the abundance of Akkermansia, the AO supplementation favoured a higher connection in the microbiota of the treated animals. The co-occurrence study indicates that AO supplementation promotes greater interaction between the genera associated with normal BP with the rest of the microorganisms in the bacterial community.
Since the antihypertensive effect of olive components is associated with the reduction of oxidative and inflammatory status,50,52 as well as with the regulation of the renin–angiotensin system (RAS) in SHR,50,51 we have included in our study the analysis of plasma concentrations of MDA, ANG II, IL6, and TNF-α.
Our results showed a higher concentration of MDA in SHR-c compared to WKY-c at 21 weeks of age, which is consistent with previous findings indicating that SHR develops high BP concomitantly with an increase of oxidative stress markers.50 We also observed that plasma concentration of ANG II in SHR-c is 3-fold higher than that in WKY-c as previously reported.54 Moreover, the supplementation with AO elicited a decrease in the plasmatic concentrations of MDA (39%) and ANG II (32%) in SHR-o compared to SHR-c. These results are consistent with other studies indicating that an antihypertensive effect in SHR rats is related to a decrease in oxidative stress biomarkers after the administration of an extra-virgin olive oil enriched with polyphenols51 and an oleuropein-enriched olive leaf extract.52
Given the implication of the gut microbiota on blood pressure,34 we performed a correlation analysis between bacterial taxa and the concentrations of MDA and ANG II. The relationship between bacterial taxa with MDA and ANG II showed a similar trend as that described for faecal microbiota and BP. Among the bacteria associated to MDA and ANG II, the AO supplementation reduced the relative abundance of Blautia and increased the relative abundance of the genera Allobaculum and Sutterella, as well as the species Bifidobacterium animalis and Bifidobacterium thermacidophilum, all inversely associated with MDA. In addition, the relative abundance of Akkermansia and the species Akkermansia muciniphila, inversely related to the concentrations of MDA and ANG II, increased in the supplemented group. It is noteworthy that an association between Akkermansia and the RAS system has been described previously.53
Among the effects of ANG II that are linked to hypertension, it is known that this hormone is able to activate the MAPK pathway through the ATR1 receptor, and initiate the signalling cascade leading to the production of proinflammatory cytokines.52,54 However, in our study, despite observing a higher plasma ANG II concentration in SHR-c, no differences in plasmatic concentration of IL6 and TNF-α were observed in any group of rats. Similar results were reported for ANG II and inflammatory cytokines in plasma from WKY and SHR rats by Vazquez et al.51
5. Conclusions
In conclusion, our results show that AO supplementation has prebiotic effects, inducing a microbiota profile compatible with its reported antihypertensive activity, which is accompanied by a reduction in plasma MDA and ANG II. Dietary AO also stimulates the growth of probiotic species of the genera Lactobacillus and Bifidobacterium as well as taxa, such as Akkermansia, Allobaculum, and Sutterella, known for their antihypertensive and cardioprotective properties. These results support the view that regular consumption of table olives may have beneficial health effects.
Author contributions
Joana M. Planas: Conceptualization, methodology, formal analysis, resources, writing-original draft, writing-review and editing, supervision, funding acquisition. Miquel Moretó: Conceptualization, methodology, formal analysis, resources, writing-original draft, writing-review and editing. Aldo Gómez-Contreras: Investigation, formal analysis, writing-original draft, writing-review and editing. M. Emília Juan: Investigation, formal analysis, writing-review and editing. Lluïsa Miró: Investigation, writing-review and editing. Talia Franco-Ávila: Investigation, writing-review and editing. All authors have read and agreed to the published version of the manuscript.
Data availability statement
Data for this paper, including body weight, food and water consumption, blood pressure, heart rate, malondialdehyde and 16S rRNA gene raw reads are available at the Science Data Bank at https://www.doi.org/10.57760/sciencedb.06495.
Conflicts of interest
The authors declared no conflict of interest.
Acknowledgements
This research was funded by the Ministerio de Economía y Competitividad (AGL2013-41188) and the Generalitat de Catalunya (2017SGR945). We thank Programa Nacional de Becas y Crédito Educativo (PRONABEC), Ministerio de Educación- (Perú) for supporting Aldo Gómez-Contreras with a pre-doctoral fellowship, and Consejo Nacional de Ciencia y Tecnología, CONACYT (México) for supporting Talia Franco-Ávila with a pre-doctoral fellowship. The authors thank Prof. Juan Carlos Laguna-Egea for providing the equipment to measure the BP. The Institut de Recerca en Nutrició i Seguretat Alimentària (INSA-UB) is Maria de Maeztu Unit of Excellence (grant CEX2021-001234-M) funded by Ministerio de Ciencia e Innovación (España).
References
- R. M. Carey, P. Muntner, H. B. Bosworth and P. K. Whelton, Prevention and control of hypertension: JACC Health Promotion Series, J. Am. Coll. Cardiol., 2018, 72, 1278–1293 CrossRef PubMed
.
- M. Guasch-Ferré and W. C. Willett, The Mediterranean diet and health: a comprehensive overview, J. Intern. Med., 2021, 290, 549–566 CrossRef PubMed
.
- M. A. Martínez-González, A. Gea and M. Ruiz-Canela, The Mediterranean diet and cardiovascular health, Circ. Res., 2019, 124, 779–798 CrossRef PubMed
.
- J. M. Lou-Bonafonte, C. Arnal, M. A. Navarro and J. Osada, Efficacy of bioactive compounds from extra virgin olive oil to modulate atherosclerosis development, Mol. Nutr. Food Res., 2012, 56, 1043–1057 CrossRef CAS PubMed
.
- S. Terés, G. Barceló-Coblijn, M. Benet, R. Alvarez, R. Bressani, J. E. Halver and P. V. Escribá, Oleic acid content is responsible for the reduction in blood pressure induced by olive oil, Proc. Natl. Acad. Sci. U. S. A., 2008, 105, 13811–13816 CrossRef PubMed
.
- M. Domènech, P. Roman, J. Lapetra, F. J. García de la Corte, A. Sala-Vila, R. de la Torre, D. Corella, J. Salas-Salvadó, V. Ruiz-Gutiérrez, R. M. Lamuela-Raventós, E. Toledo, R. Estruch, A. Coca and E. Ros, Mediterranean diet reduces 24-hour ambulatory blood pressure, blood glucose, and lipids: One-year randomized, clinical trial, Hypertension, 2014, 64, 69–76 CrossRef PubMed
.
- Regulation EU, 432/2012, Commission Regulation (EU) No 432/2012 of 16 May 2012 establishing a list of permitted health claims made on foods, other than those referring to the reduction of disease risk and to children's development and health, Off. J. Eur. Union, 2012, L136, 1–40 Search PubMed
.
- P. Otero, P. Garcia-Oliveira, M. Carpena, M. Barral-Martinez, F. Chamorro, J. Echave, P. Garcia-Perez, H. Cao, J. Xiao, J. Simal-Gandara and M. A. Prieto, Applications of by-products from the olive oil processing: Revalorization strategies based on target molecules and green extraction technologies, Trends Food Sci. Technol., 2021, 116, 1084–1104 CrossRef CAS
.
- T. B. Ribeiro, C. M. Costa, T. Bonifácio-Lopes, S. Silva, M. Veiga, A. R. Monforte, J. Nunes, A. A. Vicente and M. Pintado, Prebiotic effects of olive pomace powders in the gut: In vitro evaluation of the inhibition of adhesion of pathogens, prebiotic and antioxidant effects, Food Hydrocolloids, 2021, 112, 106312 CrossRef CAS
.
- L. Nissen, F. Casciano, E. Chiarello, M. Di Nunzio, A. Bordoni and A. Gianotti, Colonic in vitro model assessment of the prebiotic potential of bread fortified with polyphenols rich olive fiber, Nutrients, 2021, 13, 787 CrossRef CAS PubMed
.
- Y. Fan and O. Pedersen, Gut microbiota in human metabolic health and disease, Nat. Rev. Microbiol., 2021, 19, 55–71 CrossRef CAS PubMed
.
- I. Robles-Vera, M. Toral and J. Duarte, Microbiota and hypertension: role of the sympathetic nervous system and the immune system, Am. J. Hypertens., 2020, 33, 890–901 CrossRef CAS PubMed
.
- T. A. Cookson, Bacterial-induced blood pressure reduction: mechanisms for the treatment of hypertension via the gut, Front. Cardiovasc. Med., 2021, 8, 721393 CrossRef CAS PubMed
.
- T. Franco-Ávila, R. Moreno-González, M. E. Juan and J. M. Planas, Table olive elicits antihypertensive activity in spontaneously hypertensive rats, J. Sci. Food Agric., 2023, 103, 64–72 CrossRef PubMed
.
- H. Ohkawa, N. Ohishi and K. Yagi, Assay for lipid peroxides in animal tissues by thiobarbituric acid reaction, Anal. Biochem., 1979, 95, 351–358 CrossRef CAS PubMed
.
- Q. Wang, G. M. Garrity, J. M. Tiedje and J. R. Cole, Naïve bayesian classifier for rapid assignment of rRNA sequences into the new bacterial taxonomy, Appl. Environ. Microbiol., 2007, 73, 5261–5267 CrossRef CAS PubMed
.
- P. Shannon, A. Markiel, O. Ozier, N. S. Baliga, J. T. Wang, D. Ramage, N. Amin, B. Schwikowski and T. Ideker, Cytoscape: a software environment for integrated models of biomolecular interaction networks, Genome Res., 2003, 13, 2498–2504 CrossRef CAS PubMed
.
- F. M. Abboud, M. Z. Cicha, A. Ericsson, M. W. Chapleau and M. V. Singh, Altering early life gut microbiota has long-term effect on immune system and hypertension in spontaneously hypertensive rats, Front. Physiol., 2021, 12, 752924 CrossRef PubMed
.
- H. Guo, Y. Hao, X. Fan, A. Richel, N. Everaert, X. Yang and G. Ren, Administration with quinoa protein reduces the blood pressure in spontaneously hypertensive rats and modifies the fecal microbiota, Nutrients, 2021, 13, 2446 CrossRef CAS PubMed
.
- I. Robles-Vera, M. Toral, N. de La Visitacion, M. Sánchez, M. Gómez-Guzmán, R. Muñoz, F. Algieri, T. Vezza, R. Jiménez, J. Gálvez, M. Romero, J. M. Redondo and J. Duarte, Changes to the gut microbiota induced by losartan contributes to its antihypertensive effects, Br. J. Pharmacol., 2020, 177, 2006–2023 CrossRef CAS PubMed
.
- W. J. Xia, M. L. Xu, X. J. Yu, M. M. Du, X. H. Li, T. Yang, L. Li, Y. Li, K. B. Kang, Q. Su, J. X. Xu, X. L. Shi, X. M. Wang, H. B. Li and Y. M. Kang, Antihypertensive effects of exercise involve reshaping of gut microbiota and improvement of gut-brain axis in spontaneously hypertensive rat, Gut Microbes, 2021, 13, 1–24 CrossRef PubMed
.
- C. Wan, C. Zhu, G. Jin, M. Zhu, J. Hua and Y. He, Analysis of gut microbiota in patients with coronary artery disease and hypertension, J. Evidence-Based Complementary Altern. Med., 2021, 2021, 7195082 Search PubMed
.
- E. Gutiérrez-Calabrés, A. Ortega-Hernández, J. Modrego, R. Gómez-Gordo, A. Caro-Vadillo, C. Rodríguez-Bobada, P. González and D. Gómez-Garre, Gut microbiota profile identifies transition from compensated cardiac hypertrophy to heart failure in hypertensive rats, Hypertension, 2020, 76, 1545–1554 CrossRef PubMed
.
- S. Adnan, J. W. Nelson, N. J. Ajami, V. R. Venna, J. F. Petrosino, R. M. Bryan Jr. and D. J. Durgan, Alterations in the gut microbiota can elicit hypertension in rats, Physiol. Genomics, 2017, 49, 96–104 CrossRef CAS PubMed
.
- B. J. H. Verhaar, A. Prodan, M. Nieuwdorp and M. Muller, Gut microbiota in hypertension and atherosclerosis: A Review, Nutrients, 2020, 12, 2982 CrossRef CAS PubMed
.
- X. Dan, Z. Mushi, W. Baili, L. Han, W. Enqi, Z. Huanhu and L. Shuchun, Differential analysis of hypertension-associated intestinal microbiota, Int. J. Med. Sci., 2019, 16, 872–881 CrossRef CAS PubMed
.
- F. S. Thomaz, F. Altemani, S. K. Panchal, S. Worrall and M. Dekker Nitert, The influence of wasabi on the gut microbiota of high-carbohydrate, high-fat diet-induced hypertensive Wistar rats, J. Hum. Hypertens., 2021, 35, 170–180 CAS
.
- L. Serra-Majem, L. Tomaino, S. Dernini, E. M. Berry, D. Lairon, J. Ngo de la Cruz, A. Bach-Faig, L. M. Donini, F.-X. Medina, R. Belahsen, S. Piscopo, R. Capone, J. Aranceta-Bartrina, C. La Vecchia and A. Trichopoulou, Updating the Mediterranean diet pyramid towards sustainability: Focus on environmental concerns, Int. J. Environ. Res. Public Health, 2020, 17, 8758 CrossRef CAS PubMed
.
- M. Hidalgo, I. Prieto, H. Abriouel, A. B. Villarejo, M. Ramírez-Sánchez, A. Cobo, N. Benomar, A. Gálvez and M. Martínez-Cañamero, Changes in gut microbiota linked to a reduction in systolic blood pressure in spontaneously hypertensive rats fed an extra virgin olive oil-enriched diet, Plant Foods Hum. Nutr., 2018, 73, 1–6 CrossRef CAS PubMed
.
- G. Marcelino, P. A. Hiane, K. d. C. Freitas, L. F. Santana, A. Pott, J. R. Donadon and R. d. C. A. Guimarães, Effects of olive oil and its minor components on cardiovascular diseases, inflammation, and gut microbiota, Nutrients, 2019, 11, 1826 CrossRef CAS PubMed
.
- A. Cortés-Martín, M. V. Selma, F. A. Tomás-Barberán, A. González-Sarrías and J. C. Espín, Where to look into the puzzle of polyphenols and health? The postbiotics and gut microbiota associated with human metabotypes, Mol. Nutr. Food Res., 2020, 64, e1900952 CrossRef PubMed
.
- Q. Sun, M. He, M. Zhang, S. Zeng, L. Chen, L. Zhou and H. Xu, Ursolic acid: A systematic review of its pharmacology, toxicity and rethink on its pharmacokinetics based on PK-PD model, Fitoterapia, 2020, 147, 104735 CrossRef CAS PubMed
.
- Q. Zhou, G. Pang, Z. Zhang, H. Yuan, C. Chen, N. Zhang, Z. Yang and L. Sun, Association between gut Akkermansia and metabolic syndrome is dose-dependent and affected by microbial Interactions: A cross-sectional study, Diabetes, Metab. Syndr. Obes.: Targets Ther., 2021, 14, 2177–2188 CrossRef PubMed
.
- T. Yang, M. M. Santisteban, V. Rodriguez, E. Li, N. Ahmari, J. M. Carvajal, M. Zadeh, M. Gong, Y. Qi, J. Zubcevic, B. Sahay, C. J. Pepine, M. K. Raizada and M. Mohamadzadeh, Gut dysbiosis is linked to hypertension, Hypertension, 2015, 65, 1331–1340 CrossRef CAS PubMed
.
- U. Etxeberria, N. Arias, N. Boqué, M. T. Macarulla, M. P. Portillo, J. A. Martínez and F. I. Milagro, Reshaping faecal gut microbiota composition by the intake of trans-resveratrol and quercetin in high-fat sucrose diet-fed rats, J. Nutr. Biochem., 2015, 26, 651–660 CrossRef CAS PubMed
.
- H. L. Greetham, G. R. Gibson, C. Giffard, H. Hippe, B. Merkhoffer, U. Steiner, E. Falsen and M. D. Collins,
Allobaculum stercoricanis gen. nov., sp. nov., isolated from canine feces, Anaerobe, 2004, 10, 301–307 CrossRef CAS PubMed
.
- X. Zhang, Y. Zhao, M. Zhang, X. Pang, J. Xu, C. Kang, M. Li, C. Zhang, Z. Zhang, Y. Zhang, X. Li, G. Ning and L. Zhao, Structural changes of gut microbiota during berberine-mediated prevention of obesity and insulin resistance in high-fat diet-fed rats, PLoS One, 2012, 7, e42529 CrossRef CAS PubMed
.
- Y. Chen, B. Yang, C. Stanton, R. P. Ross, J. Zhao, H. Zhang and W. Chen,
Bifidobacterium pseudocatenulatum ameliorates DSS-induced colitis by maintaining intestinal mechanical barrier, blocking proinflammatory cytokines, inhibiting TLR4/NF-κB signaling, and altering gut microbiota, J. Agric. Food Chem., 2021, 69, 1496–1512 CrossRef CAS PubMed
.
- Y. Yue, Z. He, Y. Zhou, R. P. Ross, C. Stanton, J. Zhao, H. Zhang, B. Yang and W. Chen,
Lactobacillus plantarum relieves diarrhea caused by enterotoxin-producing Escherichia coli through inflammation modulation and gut microbiota regulation, Food Funct., 2020, 11, 10362–10374 RSC
.
- Z. Wang, A. B. Roberts, J. A. Buffa, B. S. Levison, W. Zhu, E. Org, X. Gu, Y. Huang, M. Zamanian-Daryoush, M. K. Culley, A. J. DiDonato, X. Fu, J. E. Hazen, D. Krajcik, J. A. DiDonato, A. J. Luisis and S. L. Hazen, Non-lethal inhibition of gut microbial trimethylamine production for the treatment of atherosclerosis, Cell, 2015, 163, 1585–1595 CrossRef CAS PubMed
.
- C. Ishii, Y. Nakanishi, S. Murakami, R. Nozu, M. Ueno, K. Hioki, W. Aw, A. Hirayama, T. Soga and M. Ito, A metabologenomic approach reveals changes in the intestinal environment of mice fed on American diet, Int. J. Mol. Sci., 2018, 19, 4079 CrossRef PubMed
.
- K. Zuo, J. Li, K. Li, C. Hu, Y. Gao, M. Chen, R. Hu, Y. Liu, H. Chi, H. Wang, Y. Qin, X. Liu, S. Li, J. Cai, J. Zhong and X. Yang, Disordered gut microbiota and alterations in metabolic patterns are associated with atrial fibrillation, GigaScience, 2019, 8, giz058 CrossRef PubMed
.
- A. F. G. Cicero, F. Fogacci, M. Bove, M. Giovannini and C. Borghi, Impact of a short-term synbiotic supplementation on metabolic syndrome and systemic inflammation in elderly patients: A randomized placebo-controlled clinical trial, Eur. J. Nutr., 2021, 60, 655–663 CrossRef CAS PubMed
.
- C. Xue, Y. Li, H. Lv, L. Zhang, C. Bi, N. Dong, A. Shan and J. Wang, Oleanolic acid targets the gut-liver axis to alleviate metabolic disorders and hepatic steatosis, J. Agric. Food Chem., 2021, 69, 7884–7897 CrossRef CAS PubMed
.
- M. C. S. Mendes, D. S. M. Paulino, S. R. Brambilla, J. A. Camargo, G. F. Persinoti and J. B. C. Carvalheira, Microbiota modification by probiotic supplementation reduces colitis associated colon cancer in mice, World J. Gastroenterol., 2018, 24, 1995–2008 CrossRef CAS PubMed
.
- Z. Zhao, A. Shi, Q. Wang and J. R. Zhou, High oleic acid peanut oil and extra virgin olive oil supplementation attenuate metabolic syndrome in rats by modulating the gut microbiota, Nutrients, 2019, 11, 3005 CrossRef CAS PubMed
.
- I. Presti, G. D'Orazio, M. Labra, B. La Ferla, V. Mezzasalma, G. Bizzaro, S. Giardina, A. Michelotti, F. Tursi, M. Vassallo and P. Di Gennaro, Evaluation of the probiotic properties of new Lactobacillus and Bifidobacterium strains and their in vitro effect, Appl. Microbiol. Biotechnol., 2015, 99, 5613–5626 CrossRef CAS PubMed
.
- H. Horiuchi, K. Kamikado, R. Aoki, N. Suganuma, T. Nishijima, A. Nakatani and I. Kimura,
Bifidobacterium animalis subsp. lactis, GCL2505 modulates host energy metabolism via the short-chain fatty acid receptor GPR43, Sci. Rep., 2020, 10, 4158 CrossRef CAS PubMed
.
- A. Barberán, S. T. Bates, E. O. Casamayor and N. Fierer, Using network analysis to explore co-occurrence patterns in soil microbial communities, ISME J., 2012, 6, 343–351 CrossRef PubMed
.
- M. Massaro, E. Scoditti, M. A. Carluccio, N. Calabriso, G. Santarpino, T. Verri and R. De Caterina, Effects of olive oil on blood pressure: epidemiological, clinical, and mechanistic evidence, Nutrients, 2020, 12, 1548 CrossRef CAS PubMed
.
- A. Vazquez, E. Sanchez-Rodriguez, F. Vargas, S. Montoro-Molina, M. Romero, J. A. Espejo-Calvo, P. Vilchez, S. Jaramillo, L. Olmo-García, A. Carrasco-Pancorbo, R. de la Torre, M. Fito, M. I. Covas, E. Martínez de Victoria and M. D. Mesa, Cardioprotective effect of a virgin olive oil enriched with bioactive compounds in spontaneously hypertensive rats, Nutrients, 2019, 11, 1728 CrossRef CAS PubMed
.
- M. Romero, M. Toral, M. Gómez-Guzmán, R. Jiménez, P. Galindo, M. Sánchez, M. Olivares, J. Gálvez and J. Duarte, Antihypertensive effects of oleuropein-enriched olive leaf extract in spontaneously hypertensive rats, Food Funct., 2016, 7, 584–593 RSC
.
- A. P. Lakshmanan, S. Murugesan, S. Al Khodor and A. Terranegra, The potential impact of a probiotic: Akkermansia muciniphila in the regulation of blood pressure-the current facts and evidence, J. Transl. Med., 2022, 20, 430 CrossRef PubMed
.
- D. Xie, Y. Shen, E. Su, L. Du, J. Xie and D. Wei, The effects of angiotensin I-converting enzyme inhibitory peptide VGINYW and the hydrolysate of α-lactalbumin on blood pressure, oxidative stress and gut microbiota of spontaneously hypertensive rats, Food Funct., 2022, 13, 2743–2755 RSC
.
|
This journal is © The Royal Society of Chemistry 2023 |
Click here to see how this site uses Cookies. View our privacy policy here.