Predicted aquatic exposure effects from a national urban stormwater study†
Received
9th December 2022
, Accepted 23rd March 2023
First published on 27th March 2023
Abstract
A multi-agency study of 438 organic and 62 inorganic chemicals measured in urban stormwater during 50 total runoff events at 21 sites across the United States demonstrated that stormwater discharges can generate localized, aquatic exposures to extensive contaminant mixtures, including organics suspected to cause adverse aquatic-health effects. The aggregated risks to multiple aquatic trophic levels (fish, invertebrates, plants) of the stormwater mixture exposures, which were documented in the national study, were explored herein by calculating cumulative ratios of organic-contaminant in vitro exposure–activity cutoffs (∑EAR) and health-benchmark-weighted cumulative toxicity quotients (∑TQ). Both risk assessment approaches indicated substantial (moderate to high) risk for acute adverse effects to aquatic organisms across multiple trophic levels (fish, macroinvertebrates, non-vascular/vascular plants) at or near stormwater discharge points across the United States. The results are interpreted as potential orders of magnitude underestimates of actual aquatic risk in stormwater control wetlands or in the immediate vicinity of such discharges to surface-water receptors, because the 438 organic-compound analytical space assessed in this study is orders of magnitude less than the 350
000 parent compounds estimated to be in current commercial use globally and the incalculable chemical-space of potential metabolites and degradates.
Water impact
Episodic stormwater discharges can cause high aquatic exposures to extensive contaminant mixtures, including organics known individually to cause adverse aquatic-health effects, in stormwater wetlands and at or near discharge points in urban streams. The results indicate substantial cumulative acute risks of adverse effects to multiple aquatic trophic levels from simultaneous exposures to the extensive contaminant mixtures reported in stormwater.
|
1 Introduction
Urban stormwater runoff and associated contaminants are well-recognized as principal drivers of the degraded biological, chemical, and physical conditions widely-observed in urban aquatic ecosystems1,2 and collectively labelled “urban stream syndrome”.3,4 Most stormwater treatment best-management practices target particulates and particulate-associated contaminants, but half or more of stormwater contaminant loading has been attributed to dissolved constituents,5 including poorly characterized mixtures of hydrophilic trace organics with known or suspected adverse ecological impacts, such as pesticides, plasticizers, and flame retardants.6 For example, the urban runoff mortality syndrome (URMS) impacting returning Pacific Northwest coho salmon (Oncorhynchus kisutch)7,8 and potentially other sensitive species9,10 has recently been attributed, at least in part, to a tire-rubber antioxidant (N-(1,3-dimethylbutyl)-N′-phenyl-p-phenylenediamine; 6PPD) quinone transformation product (6PPD-Q)11,12 released to aquatic environments by leaching of tire wear particles13 discharged in wastewater14,15 and stormwater.14,16 Likewise, recent documentation of per- and polyfluoroalkyl substances (PFAS) in street sweepings17 and road dust18,19 suggests stormwater runoff is an important pathway to aquatic ecosystems for this large class of highly persistent and toxic environmental contaminants.20,21
A broad-scope multi-agency study of organic and inorganic chemicals in urban stormwater from across the United States (US) determined that stormwater discharges can generate localized exposures to extensive and diverse contaminant mixtures, including organics suspected or known to cause adverse health effects in aquatic receptors.22 A standardized analytical toolbox, including a total of 438 organic analytes (i.e., biogenic hormones, halogenated chemicals, household/industrial chemicals, methylmercury, pesticides, pharmaceuticals, and semi-volatiles), was applied to samples collected immediately upgradient of respective discharge points at 21 sites in 17 states across the US, encompassing a total of 50 runoff events across all sites. This brief note explores the potential aggregated risks of stormwater mixture exposures to aquatic receptors for multiple aquatic trophic levels (fish, invertebrates, plants) by calculating cumulative ratios of organic-contaminant in vitro exposure–activity cutoffs and health-benchmark-weighted hazard indices based on the exposure results from the national stormwater study.22
2 Methods
2.1 Stormwater sampling and analyses
Sampling and analysis methods for the national stormwater reconnaissance study were described in detail, previously.22 Briefly, from August 2016 to December 2017, 50 flow-weighted composite storm samples (1–4 per site) from 21 sites in 17 states across the US were collected within the stormwater infrastructure just upgradient of the point of discharge to surface-water (13 or 62% of sites) or groundwater (8 or 38% of sites) receptors. Catchment areas ranged from 0.1 to 1000 km2 and encompassed residential, commercial, and industrial landscapes. Results of stormwater samples, which were collected from groundwater-infiltration stormwater control measures, are retained in the current assessment as useful exemplars of potential stormwater discharge exposures to surface aquatic habitats. Stormwater samples were analyzed for 438 organics (i.e., hormones, household and industrial chemicals, pesticides, pharmaceuticals, volatile and semi-volatile chemicals) and 62 inorganics (i.e., major ions, rare earth elements, trace elements). Among the latter, only copper (Cu) had aquatic effects benchmarks relevant to the current risk assessment. Stormwater contaminant mixture exposure results and data are available at Masoner et al.22 and Romanok et al.23
2.2 Aquatic risk screening
A screening-level exposure–activity ratio(s) (EAR) based risk assessment24–26 of potential vertebrate-centric, predominantly molecular-level effects of maximum mixed-organic contaminant exposures at each site was conducted as described.27,28 The toxEval version 1.2.0 (ref. 29) of the open source statistical software R30 was employed to sum (non-interactive concentration addition model31–33) individual ToxCast-based34,35 EAR (ratio of measured exposure concentration to activity concentration at cutoff (ACC) from the August 2020 invitroDBv3.5 release of the ToxCast database34) to predict cumulative EAR (∑EAR) under site-specific maximum exposure conditions (sum of maximum detected concentrations for all contaminants observed at a given site). Non-specific-endpoint, baseline, and unreliable response-curve assays were excluded.27,28 ∑EAR results and exclusions are summarized in Table S1a–d.†
An analogous benchmark-based toxicity quotient (TQ) assessment of aggregate organic contaminant risk to aquatic fish, invertebrates, vascular plants, and nonvascular plants also was conducted using toxEval version 1.2.0 (ref. 29) to sum (non-interactive concentration addition model31–33) individual TQ (ratio of estimated maximum exposure concentration to corresponding US Environmental Protection Agency (EPA) Office of Pesticide Programs (OPP) Aquatic Life Benchmark(s) (ALB) for acute effects to fish (AF), invertebrates (AI), vascular plants (AVP), and nonvascular plants (ANVP)36) to predict cumulative TQ (∑TQ)37 under site-specific maximum exposure conditions. Because discharge of stormwater contaminant mixtures to aquatic receptors are episodic events, TQ and ∑TQ were not calculated for chronic benchmarks. ∑TQ results and benchmarks are summarized in Table S2a–h.† Herein, the risk associated with non-detects was assumed to be negligible and excluded from ∑EAR and ∑TQ assessments.‡
3 Results and discussion
3.1 Organic contaminant mixtures – ∑EAR risk screening
ToxCast35 employs primarily vertebrate cell lines to assess exposure–response relations and activity thresholds for a broad range of biological endpoints, including endocrine disruption and neurological effects. ToxCast-based EAR values provide insight into potential sub-lethal effects and are arguably a more protective screening-level indicator of the probability of vertebrate biological effects at a measured concentration.28,38 The ToxCast EAR approach employed herein 1) leverages exposure–response relations for more than 9000 organic chemicals and approximately 1000 standardized vertebrate-cell-line bioassay endpoints,39,40 2) emphasizes molecular initiating events, which inform mechanistic understanding41–44 of possible organism-level adverse outcomes,41–43 and 3) supports estimation of cumulative effects (∑EAR) of mixed-contaminant exposures using the concentration-addition model methodology.44–47 Concentration-addition toxicities typically align with observed mixture toxicities within a factor of 2–4, regardless of mode of action.31–33 Limitations of the ToxCast EAR approach include: 1) lack of inorganic contaminant coverage, 2) notably incomplete analytical coverage of probable environmental organic exposures, 3) incomplete ToxCast coverage of detected organic analytes, 4) poorly understood translation of molecular-level effects to the organism level, and 5) unknown relevance of vertebrate-centric results to aquatic invertebrates.28,38
Risk screening based on ToxCast vertebrate-centric exposure–response data provides cumulative sub-lethal effects (∑EAR) estimation27,28,48 comparable to in vivo water-quality benchmark-based toxicity quotient (TQ) approaches,37 and is employed herein to assess the potential for molecular-scale effects to fish and other aquatic vertebrates within the 438-compound target-analyte space of the national stormwater study. ∑EAR results for estimated maximum exposure conditions are summarized in Fig. 1 and in Table S1b and d.† As described,27,48 given the diversity of organisms and corresponding contaminant vulnerabilities extant in surface-water aquatic foodwebs,49–52 we employed ∑EAR = 0.001 as a precautionary (protective) effects-screening level of concern27,37 and interpreted EAR or ∑EAR ≥ 1 as indicative of a high probability of molecular-level biological effects. We included only those compounds with individual EAR ≥ 0.00001 in the ∑EAR calculation.
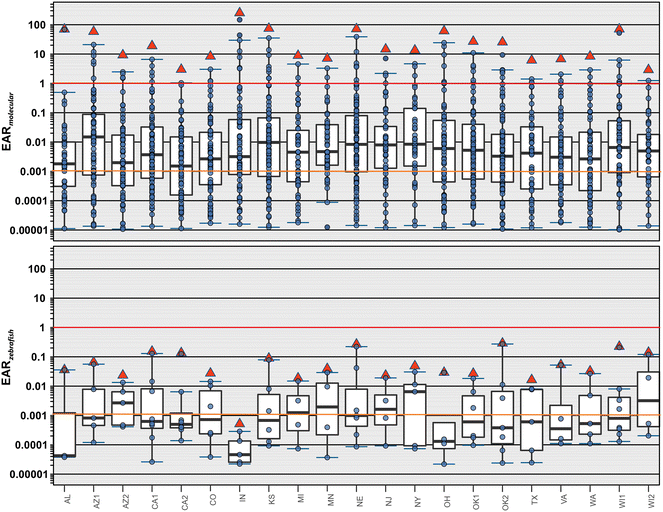 |
| Fig. 1 Individual (circles) and cumulative (red triangles) exposure–activity ratios (EAR) for vertebrate molecular endpoints (top plot) and zebrafish (Danio rerio) embryonic assay endpoints (bottom plot) for organic contaminants detected in the national stormwater study. Red (upper) and orange (lower) lines indicate concentrations shown to modulate effects in vitro (EAR = 1) and effects-screening-level thresholds (EAR = 0.001), respectively. Boxes, centerlines, and whiskers indicate interquartile range, median, and 5th and 95th percentiles, respectively, for both plots. X-Axis labels indicate state abbreviations and location numbers of stormwater sites. | |
Approximately 71% (152) of the 215 organic analytes detected at least once in this study had acceptable ToxCast data at the time of access. About 66% (142) of the detected organic analytes had individual EAR ≥ 0.00001 and were included in the ∑EAR calculation. Under the estimated maximum exposure conditions, all 21 sampled sites had one or more compounds with individual EAR greater than 1, indicating a high risk of molecular-level effects to exposed aquatic vertebrates (Fig. 1, top). Under maximum exposures, at least half of the detected analytes with ToxCast ACC data at each site had individual or cumulative EAR values equal to or greater than the 0.001 screening level of concern, with 1–7 compounds per site (median: 3) exceeding the EAR = 1 high probability of effects level.
As discussed above, a limitation of the ∑EAR approach is that most of the exposure–response relations archived in ToxCast are molecular endpoints for which adverse outcome pathways (AOP) to the organism and population scales45–47 are, with some exceptions (e.g., ref. 53 and 54), largely unknown. The zebra fish (ZF; Danio rerio) embryo high-content screening metrics in ToxCast are exceptions, informing early-life-cycle apical effects in fish and potentially providing insight into organism-level effects in other aquatic vertebrates.55,56 The zebra fish EAR results also indicated potential cumulative stormwater contaminant-mixture apical effects to fish, because all but one (95%) stormwater site in this national study had ∑EAR-max ZF equal to or greater than the 0.001 screening level of concern across all ZF endpoints and six sites (29%) had ∑EAR-max ZF ≥ 0.1, suggesting moderate risk of early-life-cycle apical effects (Fig. 1, bottom). Among the ZF endpoints predicted to be affected, activity and mortality are reasonably interpreted as adverse outcomes at organism to community levels of biological organization, as suggested previously.27
These ∑EAR results indicate that episodic stormwater exposures with a high probability of molecular effects to vertebrates and moderate risk of apical effects to early life-cycle fish are common in stormwater wetlands and at or near discharge points in urban streams across the US during stormwater events.
3.2 ∑TQ screening for fish, invertebrates, and plants
As discussed above, notable limitations of the ∑EAR risk screening approach are the lack of inorganic contaminant coverage, the predominantly vertebrate-centric ToxCast endpoints, and the poorly understood relevance to invertebrates or to vascular and nonvascular plants. As an additional line of evidence for potential risks to vertebrates and to inform the potential risks to lower aquatic trophic levels, analogous EPA OPP ALB-based ∑TQ risk approaches were employed, wherein the potential risks under the estimated maximum exposure conditions were assessed based on acute benchmarks for fish (Fig. 2, top), invertebrates (Fig. 2, bottom) and vascular/nonvascular plants (Fig. 3). We employed ∑TQ = 0.1 as a moderate risk effects-screening level and ∑TQ ≥ 1 as indicative of high risk of apical effects, as described.57,58 As for ∑EAR above, only those compounds with individual TQ ≥ 0.00001 were included in the ∑TQ calculation.
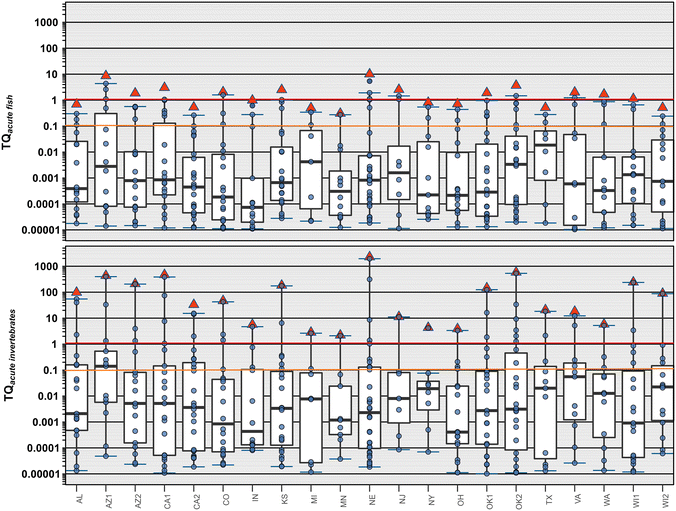 |
| Fig. 2 Environmental Protection Agency acute aquatic life benchmark (ALB) based individual (circles) and cumulative (red triangles) toxicity quotients (TQ) for fish (top plot) and invertebrates (bottom plot). Red (upper) and orange (lower) lines indicate benchmark equivalent concentrations (TQ = 1) and effects-screening-level threshold of concern (TQ = 0.1), respectively. Boxes, centerlines, and whiskers indicate interquartile range, median, and 5th and 95th percentiles, respectively, for both plots. X-Axis labels indicate state abbreviations and location numbers of stormwater sites. | |
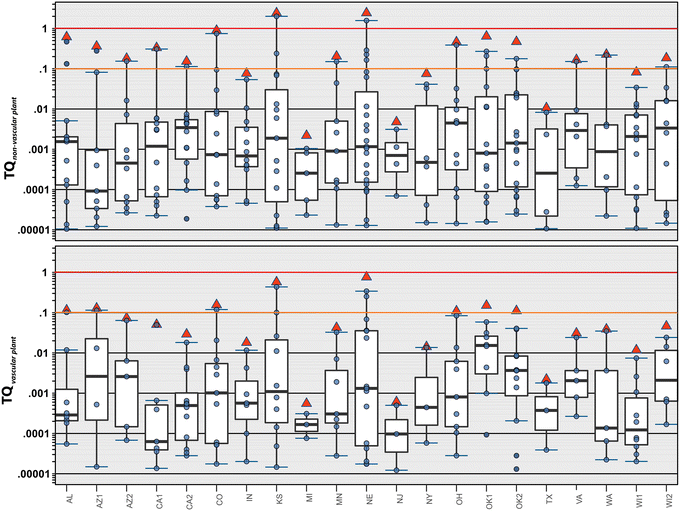 |
| Fig. 3 Environmental Protection Agency acute aquatic life benchmark (ALB) based individual (circles) and cumulative (red triangles) toxicity quotients (TQ) for non-vascular (top plot) and vascular (bottom plot) plants. Red (upper) and orange (lower) lines indicate benchmark equivalent concentrations (TQ = 1) and effects-screening-level threshold of concern (TQ = 0.1), respectively. Boxes, centerlines, and whiskers indicate interquartile range, median, and 5th and 95th percentiles, respectively, for both plots. X-Axis labels indicate state abbreviations and location numbers of stormwater sites. | |
In-stream organic contaminant concentrations are reported to exceed fish acute (or chronic) benchmarks only infrequently,27,59,60 due, at least in part, to the fact that currently available organic benchmarks are primarily for invertebrate and plant pesticides, which are evaluated for low non-target (e.g., vertebrate) potency during OPP registration.61,62 This was not the case for this stormwater study, however. About 20% (42 pesticides) of the 215 organic contaminants in the estimated maximum exposure dataset had at least one detection with an acute fish TQ (TQAF) ≥ 0.00001. Individual (TQAF) and cumulative (∑TQ-AF) TQ for acute fish toxicity exceeded the ∑TQ = 0.1 moderate risk level for acute apical effects from stormwater contaminant mixture exposures in samples from all 21 sites and the ∑TQ = 1 high risk level for acute apical effects in samples from 12 (57%) sites (Fig. 2, top). TQAF and corresponding ∑TQ-AF values were primarily driven by elevated copper, bifenthrin, lambda-cyhalothrin, and thiophanate exposures.
Twenty-two percent (47 pesticides) of the 215 organic contaminants in the estimated maximum exposure dataset had at least one detection with an acute invertebrate TQ (TQAI) ≥ 0.00001. Under these estimated maximum stormwater exposure conditions, every study site had at least one analyte (copper, lambda-cyhalothrin, cyfluthrin, fipronil) with an individual TQAI ≥ 1, indicating high risk of apical effects to aquatic invertebrates (Fig. 2, bottom). The number of contaminants equal to or exceeding TQ = 1 ranged 1–6 (median: 2) per site, with 1–10 (median: 4) contaminants per site exceeding the TQ = 0.1 screening level for moderate risk of apical effects in aquatic invertebrates. TQAI and corresponding ∑TQ-AI values were primarily driven by elevated copper, lambda-cyhalothrin, and cyfluthrin, with substantial contributions by chlorpyrifos, fipronil, and imidacloprid.
About 19% (40 pesticides) of the 215 organic contaminants in the estimated maximum exposure dataset had at least one detection with an acute non-vascular plant TQ (TQANVP) ≥ 0.00001. Under these estimated maximum stormwater exposure conditions, all but one site had at least one individual TQANVP ≥ 1 (copper, atrazine), indicating high risk of apical effects to aquatic nonvascular plants, and all sites had individual TQ > 0.1, indicating moderate risk of apical effects (Fig. 3, top). The number of contaminants exceeding the TQ = 0.1 screening level for moderate risk of apical effects in aquatic non-vascular plants ranged 1–3 (median: 2) per site. TQANVP and corresponding ∑TQ-ANVP values were primarily driven by copper and the herbicides atrazine, diuron, pendimethalin, and, to a lesser extent, acetochlor, bromacil, simazine, and oxyfluorfen.
Lastly, about 17% (37 pesticides) of the 215 organic contaminants in the estimated maximum exposure dataset had at least one detection with an acute vascular plant TQ (TQAVP) ≥ 0.00001 (Fig. 3, bottom). Eight sites had individual TQAVP and cumulative ∑TQ-AVP > 1 under the estimated maximum stormwater exposure conditions. Twelve (57%) sites had individual TQAVP > 0.1 and 13 (61%) sites had ∑TQ-AVP > 0.1, indicating moderate risk of apical effects to aquatic vascular plants. The number of contaminants exceeding the individual TQ = 0.1 screening level for moderate risk of apical effects ranged 0–4 (median: 1) per site. TQAVP and ∑TQ-AVP exceedances of the 0.1 screening level were due variously to the herbicides atrazine, diuron, pendimethalin, lamba-cyhalothrin, oxyfluorfen, and acetochlor.
These ∑TQ results indicate that stormwater discharges can result in, at a minimum, transient surface-water exposures with a substantial probability of toxic effects across multiple aquatic trophic levels, including fish, invertebrates, and aquatic plants (non-vascular and vascular) within stormwater wetlands and at or near discharge points in urban streams across the US during stormwater events.
Conclusions
Two risk assessment approaches with distinct insights and limitations were employed to evaluate the potential cumulative acute effects of estimated maximum stormwater contaminant-mixture exposure conditions to in-stream biota at multiple trophic levels. Both indicated substantial risk for adverse effects to aquatic organisms across multiple trophic levels (fish, invertebrates, non-vascular/vascular plants) within stormwater wetlands and at or near stormwater discharge points in urban streams across the US. There are several noteworthy limitations to the exploratory risk assessment approach employed in this note. To ensure a directly-comparable, expanded analyte, exposure dataset with national coverage, we explored aquatic risk based only on the national stormwater study. While the results provide compelling lines of evidence for substantial aquatic effects in stormwater wetlands and near urban-stream stormwater discharges across the US, the extent to which this limited number of sites (21) and stormwater discharge samples (50) represents the range in stormwater aquatic risk across the US is unknown. Similarly, potential risks to multiple aquatic trophic levels were explored herein based on acute EPA OPP ALB, assuming transient and localized stormwater impacts to surface-water receptors. However, the duration and frequency of stormwater events and the extent of respective stormwater impact zones are site/event-specific, are dependent on mixing and dilution in the surface-water receptor, and may be minimal for small discharges to large diluting receptors or in locations where storm events are rare. For aquatic communities in stormwater-dominated systems like stormwater control wetlands and many urban headwater streams, assessment based on chronic OPP ALB may be more appropriate and would generally result in substantially higher estimated risk. Lastly, the ∑EAR and ∑TQ approaches employed herein are based on target-chemical detections and thus intrinsically limited by the analytical detection limit (i.e., non-detects were assumed to have no risk) and by the compositional representativeness and relative environmental coverage of the target-analytical space. Thus the risk of aquatic effects estimated based on these stormwater exposure results may be reasonably interpreted as orders of magnitude underestimates, because below-detection-limit exposures may contribute to risk, and the 438 organic compound analytical space assessed in this study is orders of magnitude less than the 350
000 parent organic compounds estimated to be in current commercial use globally63 and the inestimable chemical-space of potential metabolites and degradates.64
Author contributions
PMB: conceptualization, methodology, formal analysis, writing – original draft, writing – review & editing. KMR: methodology, formal analysis, data curation, visualization, writing – review & editing. KLS: methodology, formal analysis, writing – review & editing. JRM: conceptualization, investigation, writing – review & editing. DWK: conceptualization, investigation, writing – review & editing. SEG: visualization, writing – review & editing.
Conflicts of interest
“There are no conflicts to declare”.
Acknowledgements
This research was conducted and funded by the Environmental Health Program of the U.S. Geological Survey Ecosystems Mission Area. Any use of trade, product, or firm names is for descriptive purposes only and does not imply endorsement by the U.S. Government. This report contains CAS Registry Numbers®, which is a registered trademark of the American Chemical Society. CAS recommends the verification of the CASRNs through CAS Client ServicesSM.
References
-
N. R. Council, Urban stormwater management in the United States, National Academies Press, 2009 Search PubMed.
- S. R. Weibel, R. J. Anderson and R. L. Woodward, Urban land runoff as a factor in stream pollution, J. - Water Pollut. Control Fed., 1964, 36, 914–924 CAS , https://www.jstor.org/stable/25035106.
- D. B. Booth, A. H. Roy, B. Smith and K. A. Capps, Global perspectives on the urban stream syndrome, Freshw. Sci., 2016, 35, 412–420, DOI:10.1086/684940.
- C. J. Walsh, A. H. Roy, J. W. Feminella, P. D. Cottingham, P. M. Groffman and R. P. Morgan, The urban stream syndrome: current knowledge and the search for a cure, J. North Am. Benthol. Soc., 2005, 24, 706–723, DOI:10.1899/04-028.1.
- G. H. LeFevre, K. H. Paus, P. Natarajan, J. S. Gulliver, P. J. Novak and R. M. Hozalski, Review of dissolved pollutants in urban storm water and their femoval and fate in bioretention cells, J. Environ. Eng., 2015, 141, 04014050, DOI:10.1061/(ASCE)EE.1943-7870.0000876.
- S. Spahr, M. Teixidó, D. L. Sedlak and R. G. Luthy, Hydrophilic trace organic contaminants in urban stormwater: occurrence, toxicological relevance, and the need to enhance green stormwater infrastructure, Environ. Sci.: Water Res. Technol., 2020, 6, 15–44, 10.1039/C9EW00674E.
- B. E. Feist, E. R. Buhle, P. Arnold, J. W. Davis and N. L. Scholz, Landscape ecotoxicology of Coho salmon spawner mortality in urban streams, PLoS One, 2011, 6, e23424, DOI:10.1371/journal.pone.0023424.
- N. L. Scholz, M. S. Myers, S. G. McCarthy, J. S. Labenia, J. K. McIntyre, G. M. Ylitalo, L. D. Rhodes, C. A. Laetz, C. M. Stehr, B. L. French, B. McMillan, D. Wilson, L. Reed, K. D. Lynch, S. Damm, J. W. Davis and T. K. Collier, Recurrent die-offs of adult Coho Salmon returning to spawn in Puget Sound lowland urban streams, PLoS One, 2011, 6, e28013, DOI:10.1371/journal.pone.0028013.
- K. Hiki and H. Yamamoto, The tire-derived chemical 6PPD-quinone Is lethally toxic to the White-Spotted Char Salvelinus leucomaenis pluvius but not to two other salmonid species, Environ. Sci. Technol. Lett., 2022, 9(12), 1050–1055, DOI:10.1021/acs.estlett.2c00683.
- M. Brinkmann, D. Montgomery, S. Selinger, J. G. P. Miller, E. Stock, A. J. Alcaraz, J. K. Challis, L. Weber, D. Janz, M. Hecker and S. Wiseman, Acute Toxicity of the Tire Rubber-Derived Chemical 6PPD-quinone to Four Fishes of Commercial, Cultural, and Ecological Importance, Environ. Sci. Technol. Lett., 2022, 9, 333–338, DOI:10.1021/acs.estlett.2c00050.
- Z. Tian, H. Zhao, K. T. Peter, M. Gonzalez, J. Wetzel, C. Wu, X. Hu, J. Prat, E. Mudrock, R. Hettinger, A. E. Cortina, R. G. Biswas, F. V. C. Kock, R. Soong, A. Jenne, B. Du, F. Hou, H. He, R. Lundeen, A. Gilbreath, R. Sutton, N. L. Scholz, J. W. Davis, M. C. Dodd, A. Simpson, J. K. McIntyre and E. P. Kolodziej, A ubiquitous tire rubber-derived chemical induces acute mortality in coho salmon, Science, 2021, 371, 185–189, DOI:10.1126/science.abd6951.
- Z. Tian, M. Gonzalez, C. A. Rideout, H. N. Zhao, X. Hu, J. Wetzel, E. Mudrock, C. A. James, J. K. McIntyre and E. P. Kolodziej, 6PPD-quinone: revised toxicity assessment and quantification with a commercial standard, Environ. Sci. Technol. Lett., 2022, 9, 140–146, DOI:10.1021/acs.estlett.1c00910.
- J. K. McIntyre, J. Prat, J. Cameron, J. Wetzel, E. Mudrock, K. T. Peter, Z. Tian, C. Mackenzie, J. Lundin, J. D. Stark, K. King, J. W. Davis, E. P. Kolodziej and N. L. Scholz, Treading water: Tire wear particle leachate recreates an urban runoff mortality syndrome in Coho but not Chum Salmon, Environ. Sci. Technol., 2021, 55, 11767–11774, DOI:10.1021/acs.est.1c03569.
- B. Seiwert, M. Nihemaiti, M. Troussier, S. Weyrauch and T. Reemtsma, Abiotic oxidative transformation of 6-PPD and 6-PPD quinone from tires and occurrence of their products in snow from urban roads and in municipal wastewater, Water Res., 2022, 212, 118122, DOI:10.1016/j.watres.2022.118122.
- A. Gosset, Y. Ferro and C. Durrieu, Methods for evaluating the pollution impact of urban wet weather discharges on biocenosis: A review, Water Res., 2016, 89, 330–354, DOI:10.1016/j.watres.2015.11.020.
- K. T. Peter, F. Hou, Z. Tian, C. Wu, M. Goehring, F. Liu and E. P. Kolodziej, More than a first flush: Urban creek storm hydrographs demonstrate broad contaminant pollutographs, Environ. Sci. Technol., 2020, 54, 6152–6165, DOI:10.1021/acs.est.0c00872.
- A. Ahmadireskety, B. F. Da Silva, N. M. Robey, T. E. Douglas, J. Aufmuth, H. M. Solo-Gabriele, R. A. Yost, T. G. Townsend and J. A. Bowden, Per- and polyfluoroalkyl substances (PFAS) in street sweepings, Environ. Sci. Technol., 2022, 56, 6069–6077, DOI:10.1021/acs.est.1c03766.
- M. Murakami and H. Takada, Perfluorinated surfactants (PFSs) in size-fractionated street dust in Tokyo, Chemosphere, 2008, 73, 1172–1177, DOI:10.1016/j.chemosphere.2008.07.063.
- B. K. Pramanik, R. Roychand, S. Monira, M. Bhuiyan and V. Jegatheesan, Fate of road-dust associated microplastics and per- and polyfluorinated substances in stormwater, Process Saf. Environ. Prot., 2020, 144, 236–241, DOI:10.1016/j.psep.2020.07.020.
- E. M. Sunderland, X. C. Hu, C. Dassuncao, A. K. Tokranov, C. C. Wagner and J. G. Allen, A review of the pathways of human exposure to poly- and perfluoroalkyl substances (PFASs) and present understanding of health effects, J. Exposure Sci. Environ. Epidemiol., 2019, 29, 131–147, DOI:10.1038/s41370-018-0094-1.
- G. T. Ankley, P. Cureton, R. A. Hoke, M. Houde, A. Kumar, J. Kurias, R. Lanno, C. McCarthy, J. Newsted, C. J. Salice, B. E. Sample, M. S. Sepúlveda, J. Steevens and S. Valsecchi, Assessing the ecological risks of per- and polyfluoroalkyl substances: Current state-of-the science and a proposed path forward, Environ. Toxicol. Chem., 2021, 40(3), 564–605, DOI:10.1002/etc.4869.
- J. R. Masoner, D. W. Kolpin, I. M. Cozzarelli, L. B. Barber, D. S. Burden, W. T. Foreman, K. J. Forshay, E. T. Furlong, J. F. Groves, M. L. Hladik, M. E. Hopton, J. B. Jaeschke, S. H. Keefe, D. P. Krabbenhoft, R. Lowrance, K. M. Romanok, D. L. Rus, W. R. Selbig, B. H. Williams and P. M. Bradley, Urban stormwater: An overlooked pathway of extensive mixed contaminants to surface and groundwaters in the United States, Environ. Sci. Technol., 2019, 53, 10070–10081, DOI:10.1021/acs.est.9b02867.
-
K. M. Romanok, J. R. Masoner and P. M. Bradley, Concentrations of inorganic and organic analytes in a national reconnaissance of urban stormwater runoff to surface water and groundwater in the United States, Report U.S. Geological Survey Data Release, 2019, DOI:10.5066/P96LY6US.
-
U.S. Environmental Protection Agency, EPA's National-scale Air Toxics Assessment, An Overview of Methods for EPA's National-Scale Air Toxics Assessment, 2011, https://www.epa.gov/sites/production/files/2015-10/documents/2005-nata-tmd.pdf Search PubMed.
-
U.S. Environmental Protection Agency, Framework for Cumulative Risk Assessment, Report EPA/630/P-02/001F, 2003 Search PubMed.
- M. Goumenou and A. Tsatsakis, Proposing new approaches for the risk characterisation of single chemicals and chemical mixtures: The source related Hazard Quotient (HQS) and Hazard Index (HIS) and the adversity specific Hazard Index (HIA), Toxicol. Rep., 2019, 6, 632–636, DOI:10.1016/j.toxrep.2019.06.010.
- P. M. Bradley, C. A. Journey, K. Romanok, S. Breitmeyer, D. T. Button, D. Carlisle, B. J. Huffman, B. Mahler, L. H. Nowell, S. L. Qi, K. Smalling, I. R. Waite and P. C. VanMetre, Multi-region assessment of chemical mixture exposures and predicted cumulative effects in USA wadeable urban/agriculture-gradient streams, Sci. Total Environ., 2021, 773, 145062, DOI:10.1016/j.scitotenv.2021.145062.
- B. R. Blackwell, G. T. Ankley, S. R. Corsi, L. A. De Cicco, K. A. Houck, R. S. Judson, S. Li, M. T. Martin, E. Murphy and A. Schroeder, An “EAR” on environmental surveillance and monitoring: A case study on the use of exposure-activity ratios (EARs) to prioritize sites, chemicals, and bioactivities of concern in Great Lakes waters, Environ. Sci. Technol., 2017, 51, 8713–8724, DOI:10.1021/acs.est.7b01613.
-
L. De Cicco, S. R. Corsi, D. Villeneuve, B. R. Blackwell and G. T. Ankley, toxEval: Evaluation of measured concentration data using the ToxCast high-throughput screening database or a user-defined set of concentration benchmarks, R package version 1.0.0, https://owi.usgs.gov/R/gran.html, (accessed May 1, 2018) Search PubMed.
-
R Development Core Team, R: A Language and Environment for Statistical Computing, Version 3.5.2, R Foundation for Statistical Computing, Vienna Austria, 2019 Search PubMed.
- N. Cedergreen, A. M. Christensen, A. Kamper, P. Kudsk, S. K. Mathiassen, J. C. Streibig and H. Sørensen, A review of independent action compared to concentration addition as reference models for mixtures of compounds with different molecular target sites, Environ. Toxicol. Chem., 2008, 27, 1621–1632, DOI:10.1897/07-474.1.
- R. Altenburger, M. Scholze, W. Busch, B. I. Escher, G. Jakobs, M. Krauss, J. Krüger, P. A. Neale, S. Ait-Aissa and A. C. Almeida, Mixture effects in samples of multiple contaminants–An inter-laboratory study with manifold bioassays, Environ. Int., 2018, 114, 95–106, DOI:10.1016/j.envint.2018.02.013.
- D. Stalter, E. O'Malley, U. von Gunten and B. I. Escher, Mixture effects of drinking water disinfection by-products: implications for risk assessment, Environ. Sci.: Water Res. Technol., 2020, 6, 2341–2351, 10.1039/C9EW00988D.
-
U.S. Environmental Protection Agency National Center for Computational Toxicology, ToxCast Database invitroDBv3.3, 2020, DOI:10.23645/epacomptox.6062623.v5.
-
U.S. Environmental Protection Agency National Center for Computational Toxicology, ToxCast Database InvitroDBv3.1, 2019, DOI:10.23645/epacomptox.6062623.v3.
-
U.S. Environmental Protection Agency, Office of Pesticide Programs-Aquatic Life Benchmarks and Ecological Risk Assessments for Registered Pesticides, https://www.epa.gov/pesticide-science-and-assessing-pesticide-risks/aquatic-life-benchmarks-and-ecological-risk, (accessed October 26, 2020) Search PubMed.
- S. R. Corsi, L. A. De Cicco, D. L. Villeneuve, B. R. Blackwell, K. A. Fay, G. T. Ankley and A. K. Baldwin, Prioritizing chemicals of ecological concern in Great Lakes tributaries using high-throughput screening data and adverse outcome pathways, Sci. Total Environ., 2019, 686, 995–1009, DOI:10.1016/j.scitotenv.2019.05.457.
- R. A. Becker, K. P. Friedman, T. W. Simon, M. S. Marty, G. Patlewicz and J. C. Rowlands, An exposure: activity profiling method for interpreting high-throughput screening data for estrogenic activity—Proof of concept, Regul. Toxicol. Pharmacol., 2015, 71, 398–408, DOI:10.1016/j.yrtph.2015.01.008.
- R. Kavlock, K. Chandler, K. Houck, S. Hunter, R. Judson, N. Kleinstreuer, T. Knudsen, M. Martin, S. Padilla and D. Reif, Update on EPA's ToxCast program: providing high throughput decision support tools for chemical risk management, Chem. Res. Toxicol., 2012, 25, 1287–1302, DOI:10.1021/tx3000939.
- D. L. Filer, P. Kothiya, R. W. Setzer, R. S. Judson and M. T. Martin, tcpl: the ToxCast pipeline for high-throughput screening data, Bioinformatics, 2017, 33, 618–620, DOI:10.1093/bioinformatics/btw680.
- A. M. Richard, R. S. Judson, K. A. Houck, C. M. Grulke, P. Volarath, I. Thillainadarajah, C. Yang, J. Rathman, M. T. Martin and J. F. Wambaugh, ToxCast chemical landscape: paving the road to 21st century toxicology, Chem. Res. Toxicol., 2016, 29, 1225–1251, DOI:10.1021/acs.chemrestox.6b00135.
- A. L. Schroeder, G. T. Ankley, K. A. Houck and D. L. Villeneuve, Environmental surveillance and monitoring—The next frontiers for high-throughput toxicology, Environ. Toxicol. Chem., 2016, 35, 513–525, DOI:10.1002/etc.3309.
- S. Auerbach, D. Filer, D. Reif, V. Walker, A. C. Holloway, J. Schlezinger, S. Srinivasan, D. Svoboda, R. Judson and J. R. Bucher, Prioritizing environmental chemicals for obesity and diabetes outcomes research: A screening approach using toxcast high throughput data, Environ. Health Perspect., 2016, 124, 1141–1154, DOI:10.1289/ehp.1510456.
- R. Judson, K. Houck, M. Martin, T. Knudsen, R. S. Thomas, N. Sipes, I. Shah, J. Wambaugh and K. Crofton, In vitro and modelling approaches to risk assessment from the US Environmental Protection Agency ToxCast programme, Basic Clin. Pharmacol. Toxicol., 2014, 115, 69–76, DOI:10.1111/bcpt.12239.
- R. B. Conolly, G. T. Ankley, W. Cheng, M. L. Mayo, D. H. Miller, E. J. Perkins, D. L. Villeneuve and K. H. Watanabe, Quantitative adverse outcome pathways and their application to predictive toxicology, Environ. Sci. Technol., 2017, 51, 4661–4672, DOI:10.1021/acs.est.6b06230.
- G. T. Ankley, R. S. Bennett, R. J. Erickson, D. J. Hoff, M. W. Hornung, R. D. Johnson, D. R. Mount, J. W. Nichols, C. L. Russom and P. K. Schmieder, Adverse outcome pathways: a conceptual framework to support ecotoxicology research and risk assessment, Environ. Toxicol. Chem., 2010, 29, 730–741, DOI:10.1002/etc.34.
- D. L. Villeneuve, D. Crump, N. Garcia-Reyero, M. Hecker, T. H. Hutchinson, C. A. LaLone, B. Landesmann, T. Lettieri, S. Munn and M. Nepelska, Adverse outcome pathway (AOP) development I: strategies and principles, Toxicol. Sci., 2014, 142, 312–320, DOI:10.1093/toxsci/kfu199.
- P. M. Bradley, K. M. Romanok, J. R. Duncan, W. A. Battaglin, J. M. Clark, M. L. Hladik, B. J. Huffman, L. R. Iwanowicz, C. A. Journey and K. L. Smalling, Exposure and potential effects of pesticides and pharmaceuticals in protected streams of the US National Park Service Southeast Region, Sci. Total Environ., 2020, 704, 135431, DOI:10.1016/j.scitotenv.2019.135431.
- M. L. McKinney, Urbanization as a major cause of biotic homogenization, Biol. Conserv., 2006, 127, 247–260, DOI:10.1016/j.biocon.2005.09.005.
- C. Lydeard and R. L. Mayden, A diverse and endangered aquatic ecosystem of the southeast United States, Conserv. Biol., 1995, 9, 800–805, DOI:10.1046/j.1523-1739.1995.09040800.x.
- M. L. Warren Jr, B. M. Burr, S. J. Walsh, H. L. Bart Jr, R. C. Cashner, D. A. Etnier, B. J. Freeman, B. R. Kuhajda, R. L. Mayden and H. W. Robison, Diversity, distribution, and conservation status of the native freshwater fishes of the southern United States, Fisheries, 2000, 25, 7–31, DOI:10.1577/1548-8446(2000)025<0007:DDACSO>2.0.CO;2.
- M. C. Scott, Winners and losers among stream fishes in relation to land use legacies and urban development in the southeastern US, Biol. Conserv., 2006, 127, 301–309, DOI:10.1016/j.biocon.2005.07.020.
-
D. Villeneuve, Adverse Outcome Pathway on Aromatase Inhibition Leading to Reproductive Dysfunction (in Fish), OECD Series on Adverse Outcome Pathways, OECD Publishing, Paris, 2016, no. 4, DOI:10.1787/5jlsv05mx433-en.
- D. Knapen, L. Vergauwen, D. L. Villeneuve and G. T. Ankley, The potential of AOP networks for reproductive and developmental toxicity assay development, Reprod. Toxicol., 2015, 56, 52–55, DOI:10.1016/j.reprotox.2015.04.003.
- S. Padilla, D. Corum, B. Padnos, D. L. Hunter, A. Beam, K. A. Houck, N. Sipes, N. Kleinstreuer, T. Knudsen, D. J. Dix and D. M. Reif, Zebrafish developmental screening of the ToxCast™ Phase I chemical library, Reprod. Toxicol., 2012, 33, 174–187, DOI:10.1016/j.reprotox.2011.10.018.
- L. Truong, D. M. Reif, L. St Mary, M. C. Geier, H. D. Truong and R. L. Tanguay, Multidimensional in vivo hazard assessment using zebrafish, Toxicol. Sci., 2013, 137, 212–233, DOI:10.1093/toxsci/kft235.
- E. Carazo-Rojas, G. Pérez-Rojas, M. Pérez-Villanueva, C. Chinchilla-Soto, J. S. Chin-Pampillo, P. Aguilar-Mora, M. Alpízar-Marín, M. Masís-Mora, C. E. Rodríguez-Rodríguez and Z. Vryzas, Pesticide monitoring and ecotoxicological risk assessment in surface water bodies and sediments of a tropical agro-ecosystem, Environ. Pollut., 2018, 241, 800–809, DOI:10.1016/j.envpol.2018.06.020.
- M. Kapsi, C. Tsoutsi, A. Paschalidou and T. Albanis, Environmental monitoring and risk assessment of pesticide residues in surface waters of the Louros River (N.W. Greece), Sci. Total Environ., 2019, 650, 2188–2198, DOI:10.1016/j.scitotenv.2018.09.185.
- L. H. Nowell, P. W. Moran, T. S. Schmidt, J. E. Norman, N. Nakagaki, M. E. Shoda, B. J. Mahler, P. C. Van Metre, W. W. Stone and M. W. Sandstrom, Complex mixtures of dissolved pesticides show potential aquatic toxicity in a synoptic study of Midwestern US streams, Sci. Total Environ., 2018, 613–614, 1469–1488, DOI:10.1016/j.scitotenv.2017.06.156.
- S. A. Covert, M. E. Shoda, S. M. Stackpoole and W. W. Stone, Pesticide mixtures show potential toxicity to aquatic life in U.S. streams, water years 2013–2017, Sci. Total Environ., 2020, 745, 141285, DOI:10.1016/j.scitotenv.2020.141285.
-
U.S. Environmental Protection Agency, Overview of the Ecological Risk Assessment Processin the Office of Pesticide Programs, U.S. Environmental Protection Agency, Report EPA 822-F-22-002, U.S. Environmental Protection Agency, 2004, https://www.epa.gov/sites/default/files/2014-11/documents/ecorisk-overview.pdf Search PubMed.
-
U.S. Environmental Protection Agency, Data Requirements for Pesticide Registration, (accessed February 22, 2023, https://www.epa.gov/pesticide-registration/data-requirements-pesticide-registration#Intro) Search PubMed.
- Z. Wang, G. W. Walker, D. C. G. Muir and K. Nagatani-Yoshida, Toward a global understanding of chemical pollution: a first comprehensive analysis of national and regional chemical inventories, Environ. Sci. Technol., 2020, 54, 2575–2584, DOI:10.1021/acs.est.9b06379.
- C. M. Dobson, Chemical space and biology, Nature, 2004, 432, 824–828, DOI:10.1038/nature03192.
|
This journal is © The Royal Society of Chemistry 2023 |