The formation of furan-like disinfection byproducts from phenolic precursors†
Received
18th October 2022
, Accepted 20th December 2022
First published on 22nd December 2022
Abstract
In this study, it was hypothesised that UV-absorbing disinfection byproducts (DBPs) may include compounds, such as halofuranones, prioritized as candidates for explaining the increased risk of bladder cancer associated with the consumption of chlorinated water highlighted by epidemiological studies. Hence, UV spectroscopy was used as a screening method to identify conditions forming stable UV-absorbing DBPs from 10 phenolic precursors at various pH levels, chlorine and bromide doses. Subsequently, high performance liquid chromatography coupled to high resolution mass spectrometry (Orbitrap™) was used to elucidate the chemical formulas of 30 stable DBPs, 12 of which were tentatively identified as furan-like structures, including trichlorofuran-2-carboxylic acid, dichlorofuran-2-carboxylic acid, 3,4-dichlorofuran-2,5-dicarbaldehyde, 4-chloro-5-(dichloromethyl)furan-2,3-dione, 5-formyl-2-furancarboxylic acid, chloro-5-methyl-2-furancarboxylic acid, 2-acetylchlorofuran-5-one and bromo-2-furancarboxylic acid. Eleven of the furan-like structures are previously unknown as DBPs. A novel pathway was proposed to explain their formation, involving the opening of the oxidised phenolic ring followed by the formation of a 5-membered ring by intramolecular nucleophilic addition. Of the 12 furan-like DBPs identified, eight and three were respectively predicted to be mutagens and bladder carcinogens, using a quantitative structure–activity relationship theoretical model. The findings indicate the formation of furan-like DBPs from natural organic matter surrogates is more widespread than previously appreciated. Moreover, this class of DBPs may be toxicologically significant for the urinary bladder.
Water impact
Epidemiological studies have consistently reported an association between consumption of chlorinated water and an increased risk of developing urinary bladder cancer. In this study it was hypothesised that UV-absorbing disinfection byproducts may include compounds, such as halofurans, which potentially explain this association. Twelve furan-like structures were tentatively identified following the chlorination of 10 phenolic model precursors. This class of byproducts may be toxicologically significant for the urinary bladder.
|
1. Introduction
Undesirable disinfection byproducts (DBPs) are formed during drinking water treatment when the disinfectant added to prevent waterborne diseases reacts with natural organic matter, anthropogenic contaminants, bromide and iodide. DBPs are diverse and vary depending on the natural organic matter profile of the raw water as well as disinfection conditions. Over 700 individual DBPs have been identified to date in drinking water, wastewater and synthetic samples.1 The public health risks associated with DBP formation have been investigated for more than 40 years. Epidemiological studies have repeatedly reported an association between the long-term consumption of chlorinated water (chlorine being the most common disinfectant), and an increased risk of developing urinary bladder cancer.2–7 Since chlorine itself has not been found to be carcinogenic, the formation of DBPs in chlorinated water has been implicated in this enhanced risk.8,9 A recent study accounting for 26 countries in the European Union estimated that 4.9% of the total annual bladder cancer cases are attributable to the exposure to the regulated DBPs trihalomethanes (THMs), with THM levels in drinking water used as a marker of DBP exposure.10 However, it is widely recognized that the regulated DBPs are not necessarily the most toxicologically important,11,12 and the individual compounds responsible for the increased risk of developing bladder cancer are unknown.
Therefore, there remains a need to identify plausible bladder carcinogens that are formed during chlorination of drinking water in sufficient concentrations to account for the risk observed in epidemiological studies.13 Bull et al.9 noted only limited efforts to fill this gap in DBP research. DeMarini14 highlighted the need to identify additional unknown DBPs using diverse analytical techniques, and recommended the application of disinfectant-reaction chemistry to precursors of known structure to understand better the relationship between the formation of DBPs in chlorinated water and the risk of developing cancer. Of the 76 compounds listed as bladder carcinogens in the Carcinogenic Potency Database, 83% are nitrogenous.15 However, as organic nitrogen content in water is only between 0.5 and 10% by weight of natural organic matter,16 nitrogenous DBPs will typically form in lower concentrations than many non-nitrogenous DBPs in chlorinated waters. As a result, nitrogenous DBPs would have to be very potent carcinogens if they were responsible for the association with bladder cancer. Therefore, this research focused on non-nitrogenous DBPs.
Bull et al.9 predicted the formation of halobenzoquinones and halocyclopentenoic acids as toxicologically important DBPs with the potential for being bladder carcinogens. Subsequently, researchers addressing this gap have focused on the detection, occurrence and toxicity of halobenzoquinones.17–19 In their review of the literature, Diana et al.15 noted that the furan-like compounds halocyclopentenoic acids and halofuranones as potential candidate DBPs for explaining the risk of bladder cancer observed in epidemiological studies. Various furans and associated compounds are known to be generated following the chlorination waters of natural organic matter extracts and drinking water.20–27 The best known of these is mutagen X (MX; 3-chloro-4-(dichloromethyl)-5-hydroxy-2(5H)furanone). MX is among the most potent carcinogenic DBPs15 and it has been shown to target the urinary bladder in some species.9 Its brominated analogues 4-(bromochloromethyl)-3-chloro-5-hydroxy-2(5H)-furanone (BMX-1), 3-chloro-4-(dibromomethyl)-5-hydroxy-2(5H)-furanone (BMX-2) and 3-bromo-4-(dibromomethyl)-5-hydroxy-2(5H)-furanone (BMX-3) were prioritized due to their carcinogenic potential.28 Structures of furan-type chlorination byproducts previously identified in literature are given in the ESI,† Table ESI-4.†
In this study, it was hypothesised that UV-absorbing byproducts may include overlooked compounds, such as halofuranones, prioritized as candidates for explaining the increased risk of bladder cancer associated with the consumption of chlorinated water highlighted by epidemiological studies. Its aim was to identify DBPs generated during the chlorination of phenolic precursors representative of natural organic matter with the potential to act as bladder carcinogens, through complementary application of ultraviolet (UV) spectroscopy to optimise conditions and high performance liquid chromatography with high resolution mass spectrometry (HPLC-HRMS) for product identification. Identifying potentially critical DBPs, as well as the pathways for their formation during chlorination, will contribute to DBP research by suggesting compounds that should be prioritized in future occurrence and toxicology studies.
2. Materials and methods
Sodium hypochlorite (6–14% active chlorine, EMPLURA®), phenol (BioXtra ≥99.5%), 4-hydroxybenzoic acid (ReagentPlus® ≥99%), 4-hydroxycinnamic acid (for synthesis), trans-ferulic acid (99%), 1,4-benzoquinone (certified reference material), sinapic acid (≥98%), benzoic acid (ACS reagent ≥99.5%), hydroquinone (ReagentPlus® ≥99%), pyrocatechol (≥99%), resorcinol (ACS reagent ≥99%), potassium bromide (ACS reagent), sodium phosphate monobasic monohydrate (ACS reagent ≥99%), sodium phosphate dibasic anhydrous (≥98%), ammonium acetate (ACS reagent ≥97%) and 5-formyl-2-furancarboxylic acid (AldrichCPR) were purchased from Sigma-Aldrich UK. Acetonitrile (LC-MS grade), water (LC-MS grade) and 5-bromofuran-2-carboxylic acid (99%) were purchased from Fisher Scientific UK. Stock solutions of the precursors, potassium bromide and phosphate buffers were prepared in ultrapure water (18.2 MΩ cm) and kept at 4 °C. The stock solution of chlorine was prepared daily in ultrapure water from a solution of sodium hypochlorite. Free chlorine concentrations were measured with the Hach Method 8021 using diethylparaphenylenediamine (DPD) powder sachets and a Hach meter. Chlorinated samples were prepared by diluting sodium hypochlorite, potassium bromide, sodium phosphate pH buffers and precursor stock solutions in ultrapure water. Since the focus of the study was on product or peak identification, rather than quantification, replicates were not analysed during the study.
2.1 UV absorbance screening experiments
Since halofuranones, haloquinones and halocyclopentenoic acids all absorb UV radiation, UV spectroscopy was used as a screening technique to establish chlorination conditions forming stable UV-absorbing byproducts – which may include critical byproducts for explaining the risk of bladder cancer. Phenolic compounds are a key precursor group for a broad spectrum of carbonaceous DBPs, including trihalomethanes, halophenols and halofuranones.25,29 The 10 phenolic precursors (Table ESI-1†) have all previously been investigated as model precursors to the formation of THMs29–34 and encompass a range of functionalities found in natural organic matter, including lignin substructures. The kinetics of the reactions between phenolic compounds of this type and chlorine have been studied extensively in the past, e.g. ref. 35. The initial concentrations of each precursor ranged from 25–300 μmol L−1 (or 3.2–33 mg L−1) (Table ESI-1†) and were selected in order to produce a maximum UV absorbance in the range 0.3–0.6 absorbance units, so that changes in the initial absorbance could be observed. Experimental conditions for the UV absorbance experiments are given in Table ESI-2.† The UV absorbance of the chlorinated samples was monitored every 1 nm from 200 to 400 nm with a Lambda 750 (Perkin Elmer) UV/vis spectrophotometer in quartz cuvettes of 1 cm pathlength for different pH (6–8), bromide to chlorine ratio (0 or 1 mol mol−1 for each chlorine dose) and contact times (15 seconds–7 days) (Table ESI-2†). Peaks present over the duration of these experiments are also likely to be stable under water treatment conditions. Chlorine doses (5–20 mol mol−1 of precursor) were selected to have values below and above the experimental chlorine demand of the precursors. Initial chlorine and bromide concentrations at the lowest chlorine dose of 5 mol mol−1 of precursor ranged from 10.7–106.5 mg L−1 as Cl2 and 12.0–119.9 mg L−1 as Br− respectively. The UV absorbance of the chlorinated samples was monitored for a total of 97 conditions for the 10 model precursors (Table ESI-2†), with those forming stable UV-absorbing DBPs selected for subsequent experimental stages (Table 1).
Table 1 Chlorinated samples (S1–S6) selected for DBP identification
Sample |
Precursor |
pH |
[Cl2]/[precursor] (mol/mol) |
[Br]/[precursor] (mol/mol) |
Contact time t0 |
UV spectrum |
S1 |
Phenol (PHE) |
8 |
5 |
0 |
1 d |
|
S2 |
4-Hydroxybenzoic acid (4-HBA)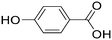 |
8 |
5 |
1 |
4 d |
|
S3 |
4-Hydroxybenzoic acid (4-HBA)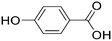 |
8 |
5 |
0 |
1 d |
|
S4 |
4-Hydroxycinnamic acid (4-HCA)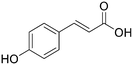 |
6 |
5 |
0 |
1 d |
|
S5 |
trans-Ferulic acid (TFA)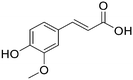 |
8 |
5 |
0 |
3 h |
|
S6 |
trans-Ferulic acid (TFA)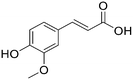 |
6 |
5 |
1 |
1 d |
|
2.2 Identification of byproducts from phenolic precursors by HPLC-HRMS
HPLC-HRMS was used to tentatively identify DBPs generated, as it is better suited for the analysis of polar and hydrophilic compounds than gas chromatography with mass spectrometry (GC-MS). Moreover, water samples can be directly analysed by liquid chromatography with mass spectrometry (LC-MS), assuming DBP concentrations are sufficiently high, whereas they have to be extracted in a solvent before being analysed by GC-MS. During extraction, some compounds may be lost, especially hydrophilic ones. Chlorinated samples selected were prepared with an initial precursor concentration of 10 mg L−1. This concentration can be considered typical of a drinking water rich in natural organic matter, for example, total organic carbon (TOC) concentrations in source waters from 23 cities in the USA and Canada ranged from 0.7–16.1 mg L−1.36 After adding 100 μL of acetonitrile to 900 μL of each sample, these were kept at −21 °C until analysis. Blanks of acetonitrile and chlorinated ultrapure water were analyzed at the beginning of each run, as well as non-chlorinated controls containing the precursors in the same conditions as the chlorinated samples (contact time, pH and presence or absence of bromide). To ensure that the peaks detected in the chlorinated samples corresponded to chlorination byproducts, only those absent in the blank and controls were considered. The non-targeted analysis of stable UV-absorbing DBPs was conducted using LC-Orbitrap-HRMS, which consisted of an Aria TLX-1 chromatographic system coupled to a Velos LTQ Orbitrap™ mass spectrometer (Thermo Scientific, San José, CA, USA) with a hybrid linear ion trap (LTQ) – Orbitrap analyzer. The conditions used were optimised following preliminary tests and are based on the non-targeted detection of DBPs in drinking water.37 20 μL of sample were injected on a Zorbax Eclipse XDB-C18 analytical column (150 × 4.6 mm, 5 μm particle size; Agilent Technologies, Santa Clara, CA, USA). The separation was achieved with an elution gradient using acetonitrile (eluent A) and water with 0.1% ammonium acetate (eluent B) as mobile phases at a flow rate of 0.5 mL min−1. The gradient program was as follows: 5% of A at 0 min; kept for 1 min; increased to 95% A at 10 min; kept for 3 min; decreased to 5% A at 15 min; kept for 1 min. Ionization was carried out with a heated electrospray ionization source (H-ESI) in both negative (ESI−) and positive (ESI+) mode. The electrospray sheath gas flow and auxiliary gas flow rates were set to 35 and 15 arbitrary units, respectively. Spray voltage was 3.5 kV. The capillary and heater temperatures were 350 °C and 300 °C. The main full-scan mode was performed with a resolution of 60
000 full width at half maximum (FWHM) at 200 m/z and the MS/MS (tandem mass spectrometry) events were acquired with a resolution of 15
000 FWHM at 200 m/z. Fragmentation was performed with a collision energy of 30 eV. The software Xcalibur 2.1 (Thermo Scientific) was used for data acquisition and quantification.
The spectra were first acquired in data dependent scan mode, and fragmentation was performed for the 3 top ions of each scan. The software Compound Discoverer v 3.1 (Thermo Scientific) was used to deconvolute the chromatographic peaks present in each sample and to propose chemical formulas. Empirical formulas were tentatively annotated considering a restricted count of elements (C1–90H1–190O0–18 N0–10S0–5P0–3Cl0–4Br0–3), a restricted H/C ratio (0.1–3.5), and a ring double bond equivalent (RDBE) ranging from 0 to 40. When the relative mass error was <3 ppm for a formula proposed, and the isotopic pattern present on the mass spectrometry (MS) spectrum matched the number of halogen atoms, the chemical formula was tentatively selected. After the first run, an inclusion list was created for each sample with the accurate m/z of DBPs detected. The samples were injected again and the ions corresponding to the m/z in the inclusion list were fragmented to obtain their MS/MS spectra, which means ions with relatively low abundance may be missed. The latter were analyzed using Metfrag with the Pubchem library.38 This software calculated a normalized score indicating how well the experimental MS/MS spectra matched those predicted for compounds with the same chemical formula in the Pubchem library. Compounds with a score of 1.00 best matched the experimental MS/MS spectra. Since some DBPs are absent from the Pubchem database, where appropriate, additional compounds matching the chemical formulas elucidated were proposed based on mechanistic analysis of likely reaction products.
For the confirmation of the detected DBPs with standards, the chlorinated samples were prepared with an initial precursor concentration of 50 mg L−1 and the same conditions as prior to LC-Orbitrap-HRMS analysis. 5-Formyl-2-furancarboxylic acid and 5-bromofuran-2-carboxylic acid standards were prepared at 10 mg L−1. After adding 100 μL of acetonitrile to 900 μL of each sample and standard, these were kept in the freezer (−21 °C) until analysis. The analysis of the chlorinated samples and the confirmation standards was conducted using a LC-qTOF-HRMS which consisted of an Agilent 1290 chromatographic system coupled to an Agilent 6550 iFunnel quadrupole time-of-flight (qToF) mass spectrometer. 10 μL of sample were injected into a Poroshell 120 EC18 analytical column (100 × 3.0 mm, 2.7 μm particle size; Agilent). The same eluents and elution gradient were used as for the non-targeted analysis by LC-Orbitrap-HRMS. Ionization was carried out with a H-ESI source in negative polarity. The electrospray drying gas and sheath gas flow rates were set to 15 and 10 mL min−1 respectively. The gas and sheath gas temperatures were 200 °C and 350 °C respectively. The MS/MS spectra were acquired with a collision energy of 35 eV. The software MassHunter Workstation Data Acquisition and MassHunter Qualitative Analysis 10.0 (Agilent) were used for data acquisition and quantification respectively.
2.3 Prediction of mutagenicity and carcinogenicity for the urinary bladder of the identified DBPs
Mutagenicity is a commonly used indicator of carcinogenicity.39 The US EPA's T.E.S.T. software40 was used for predicting the mutagenicity of selected DBPs. Their probability to be active carcinogens for the urinary bladder was calculated by an online rodent organ-specific carcinogenicity prediction tool, which uses a quantitative structure–activity relationship (QSAR) model, developed using 1011 organic compounds collated in the Carcinogenic Potency Database (CPDB), which were evaluated using a standard two-year rodent carcinogenicity bioassay.41 The probability of being active (Pa) or inactive (Pi) was calculated relative to the structure of molecules within training sets that are known active or inactive rodent carcinogens for a specific target organ. In this study mean values for male and female rats were used. DBPs having a Pa > 2 Pi for the urinary bladder were considered to be potential bladder carcinogens.
3. Results
In this study, initially UV spectroscopy was used to highlight experimental conditions under which stable UV-absorbing byproducts were generated during the chlorination of 10 phenolic precursors (section 3.1). Subsequently, the specific byproducts formed under these conditions were identified using HPLC-HRMS (section 3.2), with the structure of unknowns elucidated using analysis of MS/MS spectra (section 3.3) and mechanistic pathways (section 3.4). Subsequently, the level of confidence in the identified byproducts is discussed in section 3.5 and their predicted toxicity in section 3.6.
3.1 UV spectroscopy to highlight conditions forming stable DBPs
The formation of UV-absorbing DBPs from the selected precursors was monitored for 97 different chlorination conditions (combination of different precursors, pH, chlorine doses, and bromide doses, Table ESI-2) (Fig. ESI-1–ESI-12†). The pH, chlorine and bromide doses were found to influence the nature, the amount and the stability of the byproducts formed. In general, at higher pH and chlorine doses, the formation of DBPs was faster and these were formed in higher amounts. Some DBPs formed were stable at lower chlorine doses only.
The six samples of interest in this work (that generated stable byproducts, including furan-like compounds) are displayed in Table 1. Many of the peaks generated during chlorination were rather broad (Table 1), which may indicate they relate to a mixture of stable byproducts, rather than individual compounds. When several sets of conditions led to the formation of the same peaks, the conditions that formed the highest peaks (i.e. more DBPs) were selected. A chlorine dose of 5 mol mol−1 of precursor was selected for the chlorinated samples in subsequent experimental work because it is below the experimental chlorine demands for the precursors in the selected six samples (Table 1), which have previously been reported as 7–9 mol mol−1 of precursor in literature.34,42,43 This was to prevent the presence of chlorine residual and the need to add quenching agents. Indeed, these may degrade the byproducts formed and lead to an incorrect identification of the target compounds.44–46 These conditions (Table 1) constituted the six chlorinated samples selected for subsequent DBP identification. Their UV spectra were consistent over 7 days, indicating that the DBPs formed were stable.
3.2 Chemical formulas of DBPs detected using HPLC-HRMS
Chemical formulas for thirty DBPs were identified using LC-Orbitrap-HRMS in the six samples, all in ESI− mode. Thirteen unknown DBPs were selected as particular interest in this work and are collated in Table 2. Chemical formulas for unknown DBPs were tentatively elucidated with relative mass errors <3 ppm (Table 2). The isotopic patterns present on the MS spectra were all consistent with the number of halogen atoms in the chemical formulas elucidated for the unknown DBPs. The monoisotopic molecular weights of these 13 DBPs ranged from 140 to 214 g mol−1, and 11 were halogenated. All had either 5 or 6 carbon atoms and 4 or 5 degrees of unsaturation, suggesting that they were cyclic compounds. The Pubchem compounds database was consulted to investigate the isomers matching the chemical formulas elucidated. The number of candidate isomers for each unknown DBP ranged between 6 and 152; 40% of them were heterocyclic compounds with a 5-membered ring. Meanwhile, the 17 additional DBPs are shown in Table ESI-2.† The latter group include well-known DBPs such as dichloroacetic acid, 2,4,6-trichlorophenol and 2,4,6-tribromophenol.
Table 2 Chemical formulas elucidated for selected DBPs detected in chlorinated samples
Precursor |
Sample |
DBP ID |
Retention time (min) |
Molecular weight (g mol−1) |
Chemical formula |
Relative mass error |
Number of isomers in Pubchem |
PHE |
S1 |
01 |
7.35 |
213.89966 |
C5HCl3O3 |
2.30 |
6 |
02 |
6.51 |
179.93843 |
C5H2Cl2O3 |
1.77 |
11 |
03 |
14.10 |
191.93852 |
C6H2Cl2O3 |
1.98 |
12 |
4-HBA |
S2 |
04 |
3.37 |
205.92123 |
C5H3BrO4 |
−1.14 |
7 |
S3 |
05 |
2.52 |
207.93282 |
C6H2Cl2O4 |
−1.92 |
18 |
06 |
6.53 |
179.93793 |
C5H2Cl2O3 |
−2.03 |
11 |
07 |
7.37 |
213.89888 |
C5HCl3O3 |
−0.28 |
6 |
08 |
8.01 |
213.89886 |
C5HCl3O3 |
−0.57 |
6 |
4-HCA |
S4 |
09 |
2.60 |
156.00566 |
C6H4O5 |
−1.53 |
112 |
10 |
2.55 |
140.01063 |
C6H4O4 |
−2.10 |
152 |
TFA |
S5 |
11 |
5.82 |
159.99246 |
C6H5ClO3 |
−1.29 |
99 |
12 |
6.39 |
159.99246 |
C6H5ClO3 |
−1.29 |
99 |
S6 |
13 |
5.99 |
189.92603 |
C5H3BrO3 |
−2.43 |
20 |
3.3 Elucidating the structure of unknown DBPs from their MS/MS spectra
In order to gain information about the structure of the 13 selected unknown DBPs, and to narrow down the number of candidate isomers for each DBP, their MS/MS spectra were acquired. The fragment ions obtained gave an indication of the functional groups present in DBPs formed in the chlorinated samples. The MS/MS spectra acquired for byproducts 01 and 02 (Table 2) from phenol both exhibited the loss of a CO2 fragment, indicating that these byproducts were either esters or carboxylic acids (Fig. ESI-13.a and ESI-13.b†). The best candidate isomers for these byproducts according to their Metfrag scores were halofuroic acids and a furancarbonochloridate (Fig. ESI-13.a and ESI-13.b†). Byproduct 03 from phenol exhibited loss of CO and the best candidate isomer was another halofuran. The MS/MS spectra obtained for the byproducts of 4-hydroxybenzoic acid (Fig. 1) showed that CO and CO2 fragments (or both) were lost during fragmentation. Among the best candidate isomers for these byproducts, based on their Metfrag scores, were various furan-like compounds including halofuroic acids, a furancarbonochloridate, a halofuranone and a halofuran hydrogen carbonate (Fig. 1 and ESI-13.c†). Byproducts 09 and 10 formed from 4-hydroxycinnamic acid also exhibited the loss of CO2 and CO fragments (Fig. ESI-13.d and ESI-13.e†). Several furans or furan-like compounds were among the best candidate isomers for these byproducts. The MS/MS spectra of byproducts 11 and 12 from trans-ferulic acid showed the loss of a methyl fragment (Fig. ESI-13.f and ESI-13.g†). Since this was a common moiety among the isomers found in Pubchem for these chemical formulas, 9 and 5 candidates obtained Metfrag scores higher than 0.98 for byproducts 11 and 12 respectively (Fig. ESI-13.f and ESI-13.g†). For byproduct 13, the MS/MS spectrum exhibited the loss of a CO2 fragment indicating that this compound was either an ester or a carboxylic acid (Fig. ESI-13.h†). The best candidates for this byproduct were a brominated furoic acid and brominated furanformate (Fig. ESI-13.h†).
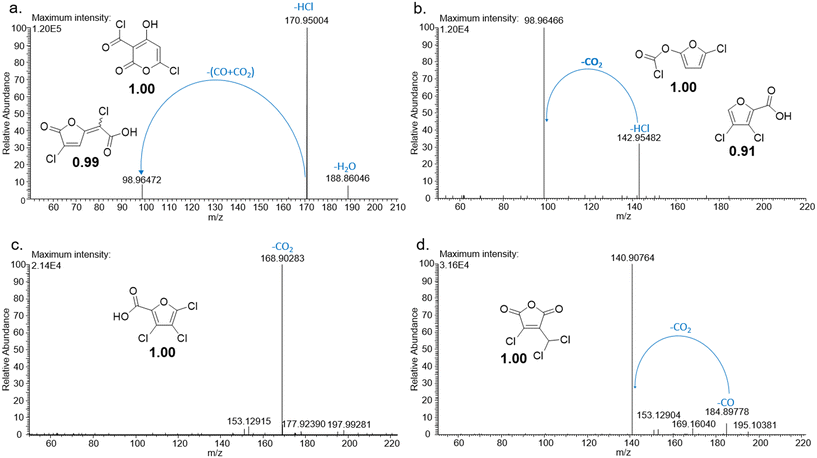 |
| Fig. 1 MS/MS spectra of disinfection byproducts from 4-hydroxybenzoic acid. a. DBP 04 (C6H2Cl2O4). b. DBP 05 (C5H2Cl2O3). c. DBP 06 (C5HCl3O3). d. DBP 07 (C5HCl3O3). The fragments lost are labelled in blue. The candidate structural isomers that obtained the best scores in Metfrag are illustrated, with their normalized Metfrag scores (for the sake of clarity, only one of the positional isomers is illustrated). | |
Analyzing the fragmentation spectra of the byproducts detected considerably reduced the number of candidate isomers for each DBP detected in the samples. For most of the byproducts (except 09, 11 and 12), the number of probable structural isomers was reduced to 1 or 2 (Fig. 1 and ESI-13†). Since many of the 13 unknown DBPs appeared to be furan-type compounds, their exact masses (Table 2) were compared with the monoisotopic masses of 57 furan-type DBPs and associated compounds reported in literature (Table ESI-3†20–24,28,47–51), however, the only match was with trichlorofuran-2-carboxylic acid, which has been tentatively identified using high resolution mass spectrometry in authentic chlorinated drinking water samples during two separate studies.49,52
3.4 Pathways for the formation of furan-like DBPs
To further reduce the number of candidate isomers matching the chemical formulas elucidated and the MS/MS spectra of the DBPs detected in the chlorinated samples, the plausibility of their formation was investigated. Among the 35 candidate isomers for the 13 DBPs (Table 2), 27 were furans or furan-like compounds (Fig. 1 and ESI-13†). Literature mechanisms for the formation of furan-like DBPs9,22,53 were unable to explain the formation of these candidates. Instead, a mechanism consisting of electrophilic aromatic substitution of chlorine, oxidation, ring opening, decarboxylation and eventually ring formation to provide a five-membered furan structure was proposed (Fig. ESI-14†), which builds upon previous work.22,53
The proposed pathway can explain the formation of a range of furan-like compounds including furans, furanols, furanones, furoic acids, and furanediones. The opening of the ring requires the presence of a carbonyl group as one of the substituents (Fig. ESI-15.a†). This is not the case for any of the selected precursors. However, they all possess hydroxyl groups that can be oxidized into carbonyl groups. A new ring can then be formed by intramolecular nucleophilic addition according to different mechanisms (Fig. ESI-15.b–d†). To enable the formation of a furan-like product, the carbon chain must possess carbonyl, carboxyl or hydroxyl groups in a 1,4 arrangement. A combination of electrophilic aromatic substitutions, chlorine substitutions and oxidations are essential to the formation of carbonyl, carboxyl and hydroxyl groups to enable the opening of the 6-membered ring and the subsequent formation of the 5-membered ring. This pathway can also explain the formation of furan-like DBPs from trans-ferulic acid and 4-hydroxycinnamic acid after they are converted to 4-chloro-1,2-benzenediol and 4-chlorophenol intermediates respectively (Fig. ESI-16†). The proposed general pathway could explain the formation of at least one of the candidate isomers proposed for the 13 DBPs detected by LC-Orbitrap-HRMS in the 6 chlorinated samples (Fig. 2 and ESI-17–ESI-21†).
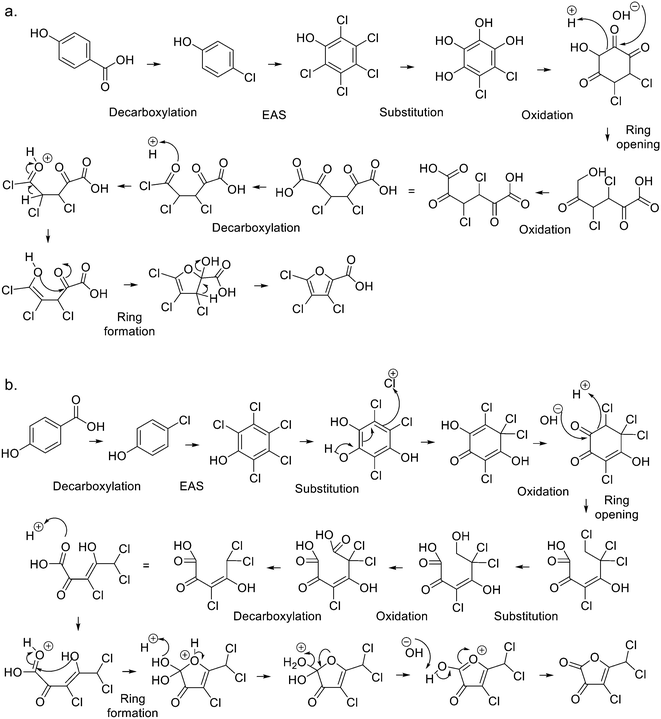 |
| Fig. 2 Proposed pathways for the formation of a halofuroic acid and a halofuranone from 4-hydroxybenzoic acid. a. Pathway for the proposed candidate for DBP 06. b. Pathway for the proposed candidate for DBP 07. | |
The proposed pathway is more flexible than related mechanisms,22,53 since substitution of OH into the precursor's six-membered ring facilitates formation of a greater range of subsequent structures. Moreover, the ring formation step can involve either carbonyl, carboxyl or hydroxyl groups in a 1,4-arrangement, rather than just the first two of these functionalities. It can be extended to account for the formation of five-membered homocyclic rings or six-membered heterocyclic rings, the latter generated when cleavage of the initial ring liberates an aliphatic structure with carbonyl, carboxyl or hydroxyl groups in a 1,5-arrangement (Fig. ESI-14†). According to this mechanism, many phenolic compounds can form furan structures upon chlorination, rather than just precursors containing oxygenated substituents in the requisite 1,4 arrangement. It was observed during the UV screening experiments that higher pH generally promoted the formation of the stable UV-absorbing DBPs, which is consistent with the initiating effect of hydroxyl in the mechanisms proposed (Fig. ESI-14–ESI-21†).
Only one of the proposed candidate structural isomers was mechanistically plausible for DBPs 01 and 02 formed from phenol (Fig. ESI-17†). Therefore, these were tentatively identified as the halofuroic acids trichlorofuran-2-carboxylic acid and dichlorofuran-2-carboxylic acid respectively (Tables 3 and ESI-4†). For the latter, the chlorine atoms can be on any of the available carbon atoms in the ring and therefore three positional isomers 3,4-; 3,5- and 4,5-dichlorofuran-2-carboxylic acid are mechanistically plausible (Table ESI-4†).
Table 3 Summary of furan-like DBPs identified, the level of confidence in their identification and their predicted mutagenicity and carcinogenic activity for the urinary bladder
Precursor |
DBP ID |
Level of confidence in DBP identification |
Plausible structures |
Predicted mutagen |
Pa |
Pi |
Only one positional isomer is illustrated in the table for clarity and brevity. Mechanistically plausible positional isomers are indicated by bonds were drawn with a dashed line. Level of confidence in DBP identification based on the level system proposed by Schymanski et al. (2014):55– Level 5: chemical formula not elucidated– Level 4: several structural isomers proposed– Level 3: only one candidate structural isomer but several positional isomers plausible– Level 2: only one positional isomer proposed– Level 1: structure confirmed with a standardPa: probability to be an active carcinogen for the urinary bladder in the rat; Pi: probability to be inactive for the urinary bladder in the rat. Pa and Pi correspond to the average of female and male rat values (standard deviations between female and male values ≤0.02). Pa values in bold: Pa > 2 Pi. Pa < Pi in female rat so the value for male rat is indicated. |
PHE; 4-HBA |
01 = 07 |
|
|
No |
0.45
|
0.19 |
02 = 06 |
|
|
Yes |
Pa < Pi |
PHE |
03 |
|
|
No |
Pa < Pi |
4-HBA |
04 |
|
|
No |
0.58
|
0.09 |
|
|
|
No |
0.34a |
0.30a |
05 |
|
|
Yes |
Pa < Pi |
|
|
|
Yes |
Pa < Pi |
08 |
|
|
Yes |
Pa < Pi |
4-HCA |
09 |
|
|
Yes |
Pa < Pi |
|
|
|
No |
0.35a |
0.29a |
|
|
|
No |
0.36 |
0.30 |
10 |
|
|
Yes |
Pa < Pi |
TFA |
11 |
|
|
Yes |
0.35 |
0.32 |
12 |
|
|
No |
0.35 |
0.32 |
13 |
|
|
Yes |
0.51
|
0.13 |
Byproducts 06 and 07 generated from 4-hydroxybenzoic acid were the same as byproducts 02 and 01 from phenol respectively (same molecular weights and same retention times) and may have formed according to the same pathway following the decarboxylation of 4-hydroxybenzoic acid (Fig. 2a and ESI-18.a†). As a result, they were also tentatively identified as dichlorofuran-2-carboxylic acid (either 3,4-; 3,5- or 4,5-dichloro isomer) and trichlorofuran-2-carboxylic acid respectively (Table ESI-4†). Similarly, DBP 03 was tentatively identified as 3,4-dichlorofuran-2,5-dicarbaldehyde (Tables 3 and ESI-4†). Only one isomer, a 6-membered heterocyclic compound, was mechanistically plausible among the candidate isomers proposed following the Metfrag analysis for DBP 04 (Fig. ESI-18.b, Table ESI-4†). However, mechanistic analysis of 4-hydroxybenzoic acid, suggested that the formation of a 4-bromo-5-hydroxyfuran-2-carboxylic acid (Fig. ESI-18.c, Tables 3 and ESI-4†) was also plausible. It is absent from the Pubchem library, thus, was not proposed as a candidate during the Metfrag analysis. Both candidate isomers, which themselves each have multiple positional isomers (Tables 3 and ESI-4†) proposed for DBP 05 were mechanistically plausible (Fig. ESI-19†). According to the proposed pathway, the regioselective substitution and oxidation of the hydroxyl groups of the tetrachlorophenol intermediate influences the formation of one byproduct or other. The only candidate structural isomer proposed for DBP 08 from 4-hydroxybenzoic acid is an oxidized form of the mutagenic DBP MX (Fig. 1.d). However, the positional isomer illustrated is not mechanistically plausible. Instead, a pathway was proposed for one of its positional isomers (Fig. 2.b), halofuranone 4-chloro-5-(dichloromethyl)furan-2,3-dione or its positional isomer 3-chloro-5-(dichloromethyl)furan-2,4-dione (Table ESI-4†), both of which are mechanistically plausible.
Three of the five candidate isomers proposed for DBP 09 from 4-hydroxycinnamic acid are mechanistically plausible (Fig. ESI-20†). These include 2 furan-like compounds and a 6-membered heterocyclic compound (Tables 3 and ESI-4†). The furoic acid 5-formyl-2-furancarboxylic acid was the only mechanistically plausible candidate for DBP 10 formed from 4-hydroxycinnamic acid (Tables 3 and ESI-4†).
The only candidate structural isomer proposed for DBP 12 from trans-ferulic acid was mechanistically plausible (Fig. ESI-21†) and was tentatively identified as 2-acetyl-2-chlorofuran-5-one or one of two positional isomers (Tables 3 and ESI-4†). DBPs 11 and 12 have the same mass, but different retention times, so are isomers (Table 2). Two of the 9 candidate isomers proposed for DBP 11 from trans-ferulic acid were mechanistically plausible (Fig. ESI-13 and ESI-21†). As one of them, 2-acetyl-2-chlorofuran-5-one, was identified as DBP 12, DBP 11 was tentatively identified as the other mechanistically plausible candidate: 4-chloro-5-methyl-2-furancarboxylic acid (Table ESI-4†). Its positional isomer 3-chloro-5-methyl-2-furancarboxylic acid is also mechanistically plausible (Tables 3 and ESI-4†). DBP 13 from trans-ferulic acid was identified as 5-bromo-2-furancarboxylic acid, the only mechanistically plausible candidate, or one of two positional isomers (Tables 3, ESI-4†). For the 13 DBPs detected in the chlorinated samples (Table 2), the number of candidate structural isomers was reduced from 35 to 15 in total (Table 3) by evaluating their mechanistic plausibility. As mentioned above, trichlorofuran-2-carboxylic acid, DBP 01 and 07 in Table 3, has previously been tentatively identified in chlorinated drinking water.49,52 However, to the best of the authors' knowledge, none of the other 11 furan-like structures identified in this work (Table 3) have previously been reported to form as DBPs in literature.
3.5 Level of confidence in DBPs identified
Among the 15 candidate structural isomers (Table 3), only 2 were commercially available: 5-formyl-2-furancarboxylic acid (candidate for DBP 10) and 5-bromofuran-2-carboxylic acid (candidate for DBP 13). These were analysed by the LC-qTOF-HRMS and compared with the DBPs in the chlorinated samples. No peak was observed at the m/z and retention time of 5-formyl-2-furancarboxylic acid in the chlorinated sample of 4-hydroxycinnamic acid. It is possible that an isomer of 5-formyl-2-furancarboxylic acid, not present in the Pubchem compounds database, corresponded to DBP 09 instead. 5-Bromofuran-2-carboxylic acid and DBP 13 eluted at the same retention time of 2.0 minutes (Fig. ESI-22†). They exhibited similar MS spectra but different MS/MS spectra (Fig. ESI-22†), which suggests that a positional isomer of 5-bromofuran-2-carboxylic acid, for example, 3-bromo- or 4-bromofuran-2-carboxylic acid, was generated instead. It is known that positional isomers often elute at very similar retention times, for example, 3 positional isomers of fluorofentanyl and fluoromethcathinone had retention times varying between 0.00 and 0.16 minutes on different instruments.54 Their MS spectra exhibited the same ions, but the ratios of the abundance values were different for various ions.54
For DBPs 01, 03, 07, 08 and 10, only one candidate positional isomer remained plausible after mechanistic analysis (Table 3). Therefore, these were identified with a confidence level 2 (probable structure) according to the system described by Schymanski et al.55 For DBPs 02, 06, 11, 12 and 13 investigating the plausibility of the formation of the candidate isomers could reduce their number to only one structural isomer (Table 3). However, 2 to 4 positional isomers were plausible and therefore, these DBPs were identified with a confidence level of 3 (tentative identification). As for DBPs 04, 05 and 09, the number of candidates was reduced to either 2 or 3 structural isomers (and their positional isomers) and these were therefore identified with a confidence level of 4 (Table 3).
In summary, 12 furans or furan-like candidate isomers were mechanistically plausible products from the four phenolic precursors phenol, 4-hydroxybenzoic acid, 4-hydroxycinnamic acid and trans-ferulic acid (Table 3). These candidates relate to chemical formulas for 13 different DBPs. Note that two pairs of these DBPs, generated from different precursors, are duplicates (01 = 07 and 02 = 06), as they had the same mass, retention time and molecular formula. Previously, MX and its linear isomer, E-MX, have been reported as products of various phenolic and aromatic natural organic matter surrogates,25,56 but the present work indicates that the formation of furan-type DBPs from natural organic matter surrogates encompasses a wider variety of structures than appreciated hitherto.
3.6 Predicted toxicity of the DBPs tentatively identified
The probabilities for the 15 mechanistically plausible structures, 12 of which were furan-like DBPs, to be mutagens and active carcinogens for the urinary bladder were calculated (Table 3). The Pa values relate the probability to be active for the urinary bladder whereas Pi is the probability to be inactive. Compounds having Pa > 2 Pi were considered predicted bladder carcinogens, as their probability to be active is at least twice of being inactive. Eight of the 15 plausible structures were predicted mutagens. Three halofuroic acids were predicted bladder carcinogens: trichlorofuran-2-carboxylic acid (DBP 01 and 07 from phenol and 4-hydroxybenzoic acid), 4-bromo-5-hydroxyfuran-2-carboxylic acid (DBP 04 from 4-hydroxybenzoic acid) and 5-bromofuran-2-carboxylic acid (DBP 13 from trans-ferulic acid) (Table 3). The latter two, both brominated furoic acids, had Pa values >0.50. For comparison, the same probabilities were calculated for 57 furan-type DBPs reported in literature (including trichlorofuran-2-carboxylic acid), as well as seven nitrosamines known as DBPs (Table ESI-5†). Note that carcinogenicity values cannot be predicted by this method for simpler DBPs such as THMs or NDMA (N-nitrosodimethylamine), as such structures are absent from the training sets used to establish the relevant QSAR models. Of the 57 literature furan-type DBPs, 44 are predicted mutagens, but only ten are potential bladder carcinogens (i.e. Pa > 2 Pi) (Table ESI-5†). The nitrosamines are a group of recognised carcinogens and of the seven analysed in Table ESI-5,† six are predicted mutagens and five potential bladder carcinogens.
4. Discussion: implications for drinking water treatment
It is important to note that the byproducts reported in this study were tentatively identified from the chlorination of natural organic matter surrogates. In the future it will be important to confirm the presence of the halofurans identified in authentic drinking water samples and of intermediates to support the mechanistic pathways proposed. Moreover, in natural waters iodinated analogues of the DBPs identified, which were not considered here, are possible products of the chlorination of natural organic matter, of note since iodinated DBPs may act as forcing agents for the cytotoxicity of drinking water.57 A related focus for research efforts is likely to be controlling the concentrations of toxicologically-significant DBPs in drinking water. In their study, Evlampidou et al.10 estimated that reducing the mean THM levels (used as a surrogate for DBPs exposure) to the European Union average for 13 countries with higher exposures would reduce the estimated number of attributable bladder cancer cases by 44%. Identifying the specific DBPs responsible for bladder cancer and targeting their control could significantly reduce the risk of bladder cancer arising from the consumption of chlorinated water. This includes looking for carcinogens within the pool of nitrogenous disinfection byproducts, many known to be more cytotoxic and genotoxic than non-nitrogenous analogues when assessed by Chinese hamster ovary (CHO) cell bioassays.58,59 With respect to minimizing the formation of furan-like DBPs, the removal of phenolic precursors prior to chlorination will be a profitable approach. Processes, such as activated carbon and membranes, which target the residual aromatic/hydrophobic/phenolic natural organic matter remaining after the application of conventional drinking water treatment processes (i.e. coagulation–flocculation–sedimentation–filtration) are predicted to be effective in this regard, as previously demonstrated for micropollutants including antibiotics, e.g. ref. 60. The application of chemical oxidation processes, such as ozone, requires a cautious assessment of byproduct toxicity relative to the starting material. Chevrier et al.61 observed a reduced bladder cancer risk from ozone disinfected drinking water in comparison with chlorinated water, while furans were recently reported to be highly reactive towards ozone.62 However, highly toxic α,β-unsaturated dicarbonyls are a significant byproduct of these reactions.62
5. Conclusions
In this work, UV spectroscopy was used to identify conditions forming stable UV-absorbing DBPs from 10 phenolic precursors. Subsequently, HPLC-HRMS was used to measure the masses of DBPs generated. Key contributions to knowledge are as follows:
• Chemical formulas for 30 stable DBPs formed were elucidated. Among them, 12 mechanistically-plausible structures, relating to 13 different DBPs, were identified as furan-like compounds, including trichlorofuran-2-carboxylic acid, dichlorofuran-2-carboxylic acid, 3,4-dichlorofuran-2,5-dicarbaldehyde, 4-chloro-5-(dichloromethyl)furan-2,3-dione, 5-formyl-2-furancarboxylic acid, chloro-5-methyl-2-furancarboxylic acid, 2-acetylchlorofuran-5-one and bromo-2-furancarboxylic acid. Eleven of these have never before been reported in literature as DBPs.
• Among the 12 plausible furan-like structures identified, eight were predicted to be mutagenic and three were predicted to be bladder carcinogens, which suggests that this class of DBPs may be toxicologically significant for the urinary bladder.
• The study indicates that the formation of halogenated furan-like DBPs from phenolic precursors often used as surrogates of NOM is more widespread than previously appreciated.
Abbreviations
BMX-1 | 4-(Bromochloromethyl)-3-chloro-5-hydroxy-2(5H)-furanone |
BMX-2 | 3-Chloro-4-(dibromomethyl)-5-hydroxy-2(5H)-furanone |
BMX-3 | 3-Bromo-4-(dibromomethyl)-5-hydroxy-2(5H)-furanone |
DBP | Disinfection byproduct |
DPD | Diethylparaphenylenediamine |
GC-MS | Gas chromatography with mass spectrometry |
H-ESI | Heated electrospray ionisation |
HPLC-HRMS | High performance liquid chromatography with high resolution mass spectrometry |
LC-MS | Liquid chromatography with mass spectrometry |
MS | Mass spectrometry |
MS/MS | Tandem mass spectrometry |
MX | Mutagen X – 3-chloro-4-(dichloromethyl)-5-hydroxy-2(5H)furanone |
NDMA |
N-Nitrosodimethylamine |
qToF | Quadrupole time-of-flight qToF |
QSAR | Quantitative structure–activity relationship |
PHE | Phenol |
RDBE | Ring double bond equivalent |
red-MX | 3-Chloro-4-(dichloromethyl)-2(5H)-furanone |
TFA |
trans-Ferulic acid |
THM | Trihalomethane |
UV | Ultra-violet |
Author contributions
Marine Diana: investigation, writing – original draft preparation, formal analysis. Maria José Farré: methodology, writing – reviewing and editing, investigation, supervision. Josep Sanchís: methodology, writing – reviewing and editing, investigation, supervision. Rakesh Kanda: methodology, writing – reviewing and editing. Mónica Felipe-Sotelo: methodology, writing – reviewing and editing, supervision. Tom Bond: conceptualization, resources, supervision, writing – original draft, writing – review & editing, supervision, project administration, funding acquisition.
Conflicts of interest
There are no conflicts of interest to declare.
Acknowledgements
The Department of Civil and Environmental Engineering at the University of Surrey is gratefully acknowledged for funding this project. ICRA researchers thank funding from the CERCA program. MJF acknowledges her Ramón y Cajal fellowship (RyC-2015-17108), from the AEI-MICIU.
References
-
M. Thun, M. S. Linet, J. R. Cerhan, C. A. Haiman and D. Schottenfeld, Cancer Epidemiology and Prevention, Oxford University Press: Oxford, UK, 4th edn, 2017 Search PubMed.
- C. M. Villanueva, F. Fernández, N. Malats, J. O. Grimalt and M. Kogevinas, Meta-Analysis of Studies on Individual Consumption of Chlorinated Drinking Water and Bladder Cancer, J. Epidemiol. Community Health, 2003, 57(3), 166–173, DOI:10.1136/JECH.57.3.166.
- W. D. King and L. D. Marrett, Case-Control Study of Bladder Cancer and Chlorination by-Products in Treated Water (Ontario, Canada), Cancer Causes Control, 1996, 7(6), 596–604, DOI:10.1007/BF00051702.
- M. A. Mcgeehin, J. S. Reif, J. C. Becher and E. J. Mangione, Case-Control Study of Bladder Cancer and Water Disinfection Methods in Colorado, Am. J. Epidemiol., 1993, 138(7), 492–501, DOI:10.1093/OXFORDJOURNALS.AJE.A116883.
- J. R. Wilkins and G. W. Comstock, Source of Drinking Water at Home and Site-Specific Cancer Incidence in Washington County, Maryland, Am. J. Epidemiol., 1981, 114(2), 178–190, DOI:10.1093/OXFORDJOURNALS.AJE.A113181.
- M. Koivusalo, T. Hakulinen, T. Vartiainen, E. Pukkala, J. J. K. Jaakkola and J. Tuomisto, Drinking Water Mutagenicity and Urinary Tract Cancers: A Population-Based Case-Control Study in Finland, Am. J. Epidemiol., 1998, 148(7), 704–712, DOI:10.1093/AJE/148.7.704.
- C. M. Villanueva, K. P. Cantor, S. Cordier, J. J. K. Jaakkola, W. D. King, C. F. Lynch, S. Porru and M. Kogevinas, Disinfection Byproducts and Bladder Cancer: A Pooled Analysis, Epidemiology, 2004, 15(3), 357–367, DOI:10.1097/01.EDE.0000121380.02594.FC.
-
M. Lippmann, Environmental Toxicants: Human Exposures and Their Health Effects, ed. M. Lippmann, Wiley, 3rd edn, 2009, DOI:10.1002/9781119438922.
-
R. J. Bull, D. A. Reckhow, V. Rotello, O. M. Bull and J. Kim, Use of Toxicological and Chemical Models to Prioritize DBP Research, Denver, CO, USA, 2006 Search PubMed.
- I. Evlampidou, L. Font-Ribera, D. Rojas-Rueda, E. Gracia-Lavedan, N. Costet, N. Pearce, P. Vineis, J. J. K. Jaakkola, F. Delloye, K. C. Makris, E. G. Stephanou, S. Kargaki, F. Kozisek, T. Sigsgaard, B. Hansen, J. Schullehner, R. Nahkur, C. Galey, C. Zwiener, M. Vargha, E. Righi, G. Aggazzotti, G. Kalnina, R. Grazuleviciene, K. Polanska, D. Gubkova, K. Bitenc, E. H. Goslan, M. Kogevinas and C. M. Villanueva, Trihalomethanes in Drinking Water and Bladder Cancer Burden in the European Union, Environ. Health Perspect., 2020, 128(1), 1–14, DOI:10.1289/EHP4495.
- S. D. Richardson and M. J. Plewa, To Regulate or Not to Regulate? What to Do with More Toxic Disinfection by-Products?, J. Environ. Chem. Eng., 2020, 8(4), 103939, DOI:10.1016/j.jece.2020.103939.
- T. Bond, W. Chu, U. Von Gunten and M. J. Farré, Themed Issue on Drinking Water Oxidation and Disinfection Processes, Environ. Sci.: Water Res. Technol., 2020, 2252–2256, 10.1039/d0ew90042g.
- D. Stalter, E. O'Malley, U. Von Gunten and B. I. Escher, Mixture Effects of Drinking Water Disinfection By-Products: Implications for Risk Assessment, Environ. Sci.: Water Res. Technol., 2020, 6(9), 2341–2351, 10.1039/C9EW00988D.
- D. M. DeMarini, A Review on the 40th Anniversary of the First Regulation of Drinking Water Disinfection By-Products, Environ. Mol. Mutagen., 2020, 61(6), 588–601, DOI:10.1002/EM.22378.
- M. Diana, M. Felipe-Sotelo and T. Bond, Disinfection Byproducts Potentially
Responsible for the Association between Chlorinated Drinking Water and Bladder Cancer: A Review, Water Res., 2019, 162, 492–504, DOI:10.1016/J.WATRES.2019.07.014.
- P. Westerhoff and H. Mash, Dissolved Organic Nitrogen in Drinking Water Supplies: A Review, J. Water Supply: Res. Technol.--AQUA, 2002, 51(8), 415–448, DOI:10.2166/AQUA.2002.0038.
- J. Li, W. Wang, B. Moe, H. Wang and X. F. Li, Chemical and Toxicological Characterization of Halobenzoquinones, an Emerging Class of Disinfection Byproducts, Chem. Res. Toxicol., 2015, 28(3), 306–318, DOI:10.1021/TX500494R.
- Y. Zhao, F. Qin, J. M. Boyd, J. Anichina and X. F. Li, Characterization and Determination of Chloro- and Bromo-Benzoquinones as New Chlorination Disinfection Byproducts in Drinking Water, Anal. Chem., 2010, 82(11), 4599–4605, DOI:10.1021/AC100708U.
- H. Du, J. Li, B. Moe, C. F. McGuigan, S. Shen and X. F. Li, Cytotoxicity and Oxidative Damage Induced by Halobenzoquinones to T24 Bladder Cancer Cells, Environ. Sci. Technol., 2013, 47(6), 2823–2830, DOI:10.1021/ES303762P.
- L. Kronberg, B. Holmbom, M. Reunanen and L. Tikkanen, Identification and Quantification of the Ames Mutagenic Compound 3-Chloro-4-(Dichloromethyl)-5-Hydroxy-2(5H)-Furanone and of Its Geometric Isomer (E)-2-Chloro-3-(Dichloromethyl)-4-Oxobutenoic Acid in Chlorine-Treated Humic Water and Drinking Water Extract, Environ. Sci. Technol., 1988, 22(9), 1097–1103, DOI:10.1021/ES00174A016.
- L. Kronberg, R. F. Christman, R. Singh and L. M. Ball, Identification of Oxidized and Reduced Forms of the Strong Bacterial Mutagen (Z)-2-Chloro-3-(Dichloromethyl)-4-Oxobutenoic Acid (MX) in Extracts of Chlorine-Treated Water, Environ. Sci. Technol., 1991, 25(1), 99–104, DOI:10.1021/ES00013A009.
- L. Kronberg and R. Franzen, Determination of Chlorinated Furanones, Hydroxyfuranones, and Butenedioic Acids in Chlorine-Treated Water and in Pulp Bleaching Liquor, Environ. Sci. Technol., 1993, 27(9), 1811–1818, DOI:10.1021/ES00046A008.
- R. Franzen and L. Kronberg, Determination of Chlorinated 5-Methyl-5-Hydroxyfuranones in Drinking Water, in Chlorinated Humic Water, and in Pulp Bleaching Liquor, Environ. Sci. Technol., 1994, 28(12), 2222–2227, DOI:10.1021/ES00061A035.
- A. Smeds, R. Franzen and L. Kronberg, Occurrence of Some Chlorinated Enol Lactones and Cyclopentene-1,3-Diones in Chlorine-Treated Waters, Environ. Sci. Technol., 1995, 29(7), 1839–1844, DOI:10.1021/ES00007A022.
- V. A. Långvik, O. Hormi, L. Tikkanen and B. Holmbom, Formation of the Mutagen 3-Chloro-4-(Dichloromethyl)-5-Hydroxy-2(5H)-Furanone and Related Compounds by Chlorination of Phenolic Compounds, Chemosphere, 1991, 22(5–6), 547–555, DOI:10.1016/0045-6535(91)90066-M.
- S. W. Krasner, H. S. Weinberg, S. D. Richardson, S. J. Pastor, R. Chinn, M. J. Sclimenti, G. D. Onstad and A. D. Thruston, Occurrence of a New Generation of Disinfection Byproducts, Environ. Sci. Technol., 2006, 40(23), 7175–7185, DOI:10.1021/ES060353J/SUPPL_FILE/ES060353JSI20060430_023458.PDF.
-
H. S. Weinberg, S. W. Krasner, S. D. Richardson and A. D. Thruston, Jr, The Occurrence of Disinfection By-Products (DBPs) of Nationwide Health Concern in Drinking Water: Results of a DBP Occurrence Study, Athens, GA, 2002 Search PubMed.
- Y. T. Woo, D. Lai, J. L. McLain, M. K. Manibusan and V. Dellarco, Use of Mechanism-Based Structure-Activity Relationships Analysis in Carcinogenic Potential Ranking for Drinking Water Disinfection by-Products, Environ. Health Perspect., 2002, 110(Suppl 1), 75, DOI:10.1289/EHP.02110S175.
- T. Bond and N. Graham, Predicting Chloroform Production from Organic Precursors, Water Res., 2017, 124, 167–176, DOI:10.1016/j.watres.2017.07.063.
- J. de Laat, N. Merlet and M. Dore, Chloration de Composés Organiques: Demande En Chlore et Réactivite Vis-a-Vis de La Formation Des Trihalométhanes. Incidence de l'azote Ammoniacal, Water Res., 1982, 16(10), 1437–1450, DOI:10.1016/0043-1354(82)90242-1.
- R. A. Larson and A. L. Rockwell, Chloroform and Chlorophenol Production
by Decarboxylation of Natural Acids during Aqueous Chlorination, Environ. Sci. Technol., 1979, 13(3), 325–329, DOI:10.1021/ES60151A014/ASSET/ES60151A014.FP.PNG_V03.
- J. J. Rook, Chlorination Reactions of Fulvic Acids in Natural Waters, Environ. Sci. Technol., 1977, 11(5), 478–482, DOI:10.1021/ES60128A014/ASSET/ES60128A014.FP.PNG_V03.
- D. L. Norwood, J. D. Johnson, R. F. Christman, J. R. Hass and M. J. Bobenrieth, Reactions of Chlorine With Selected Aromatic Models of Aquatic Humic Material, Environ. Sci. Technol., 1980, 14(2), 187–190, DOI:10.1021/ES60162A012/ASSET/ES60162A012.FP.PNG_V03.
- T. Bond, O. Henriet, E. H. Goslan, S. A. Parsons and B. Jefferson, Disinfection Byproduct Formation and Fractionation Behavior of Natural Organic Matter Surrogates, Environ. Sci. Technol., 2009, 43(15), 5982–5989, DOI:10.1021/ES900686P/SUPPL_FILE/ES900686P_SI_001.PDF.
- M. Deborde and U. von Gunten, Reactions of Chlorine with Inorganic and Organic Compounds during Water Treatment—Kinetics and Mechanisms: A Critical Review, Water Res., 2008, 42(1–2), 13–51, DOI:10.1016/J.WATRES.2007.07.025.
- S. D. Richardson, F. Fasano, J. J. Ellington, F. G. Crumley, K. M. Buettner, J. J. Evans, B. C. Blount, L. K. Silva, T. J. Waite, G. W. Luther, A. B. Mckague, R. J. Miltner, E. D. Wagner and M. J. Plewa, Occurrence and Mammalian Cell Toxicity of Iodinated Disinfection Byproducts in Drinking Water, Environ. Sci. Technol., 2008, 42(22), 8330–8338, DOI:10.1021/ES801169K/SUPPL_FILE/ES801169K_SI_001.PDF.
- J. Sanchís, P. E. Redondo-Hasselerharm, C. M. Villanueva and M. J. Farré, Non Targeted Screening of Nitrogen Containing Disinfection By-Products in Formation Potential Tests of River Water and Subsequent Monitoring in Tap Water Samples, Chemosphere, 2022, 303, 135087, DOI:10.1016/J.CHEMOSPHERE.2022.135087.
- C. Ruttkies, E. L. Schymanski, S. Wolf, J. Hollender and S. Neumann, MetFrag Relaunched: Incorporating Strategies beyond in Silico Fragmentation, J. Cheminf., 2016, 8(1), 1–16, DOI:10.1186/S13321-016-0115-9/TABLES/7.
- P. Pradeep, R. J. Povinelli, S. J. Merrill, S. Bozdag and D. S. Sem, Novel Uses of In Vitro Data to Develop Quantitative Biological Activity Relationship Models for in Vivo Carcinogenicity Prediction, Mol. Inf., 2015, 34(4), 236–245, DOI:10.1002/MINF.201400168.
-
US EPA, T.E.S.T. (Version 5.1) (Toxicity Estimation Software Tool): A Program to Estimate Toxicity from Molecular Structure, 2020.
- A. Lagunin, A. Rudik, D. Druzhilovsky, D. Filimonov and V. Poroikov, ROSC-Pred: Web-Service for Rodent Organ-Specific Carcinogenicity Prediction, Bioinformatics, 2018, 34(4), 710–712, DOI:10.1093/BIOINFORMATICS/BTX678.
- T. Bond, E. H. Goslan, S. A. Parsons and B. Jefferson, A Critical Review of Trihalomethane and Haloacetic Acid Formation from Natural Organic Matter Surrogates, Environ. Technol. Rev., 2012, 1(1), 93–113, DOI:10.1080/09593330.2012.705895.
- H. Gallard and U. von Gunten, Chlorination of Phenols: Kinetics and Formation of Chloroform, Environ. Sci. Technol., 2002, 36(5), 884–890, DOI:10.1021/ES010076A.
- J. P. Croue and D. A. Reckhow, Destruction of Chlorination Byproducts with Sulfite, Environ. Sci. Technol., 2002, 23(11), 1412–1419, DOI:10.1021/ES00069A014.
- Y. Yu and D. A. Reckhow, Formation and Occurrence of N-Chloro-2,2-Dichloroacetamide, a Previously Overlooked Nitrogenous Disinfection Byproduct in Chlorinated Drinking Waters, Environ. Sci. Technol., 2017, 51(3), 1488–1497, DOI:10.1021/ACS.EST.6B04218/SUPPL_FILE/ES6B04218_SI_001.PDF.
- J. Liu and X. Zhang, Effect of Quenching Time and Quenching Agent Dose on Total Organic Halogen Measurement, Int. J. Environ. Anal. Chem., 2013, 93(11), 1146–1158, DOI:10.1080/03067319.2012.727807.
- H. Gong, H. Wang, Z. You, H. Zou and X. Shen, Molecular Structure of a New Chlorinated Disinfection By-Product in Drinking Water, J. Mol. Struct., 2005, 748(1–3), 71–76, DOI:10.1016/J.MOLSTRUC.2005.03.013.
- C. Li, D. Wang, X. Xu, M. Xu and Z. Wang, Spatial Variations in the Occurrence of Potentially Genotoxic Disinfection By-Products in Drinking Water Distribution Systems in China, Environ. Pollut., 2017, 231, 1463–1468, DOI:10.1016/J.ENVPOL.2017.09.008.
- C. Postigo, A. Andersson, M. Harir, D. Bastviken, M. Gonsior, P. Schmitt-Kopplin, P. Gago-Ferrero, L. Ahrens, L. Ahrens and K. Wiberg, Unraveling the Chemodiversity of Halogenated Disinfection By-Products Formed during Drinking Water Treatment Using Target and Non-Target Screening Tools, J. Hazard. Mater., 2021, 401, 123681, DOI:10.1016/J.JHAZMAT.2020.123681.
- S. D. Richardson, M. J. Plewa, E. D. Wagner, R. Schoeny and D. M. DeMarini, Occurrence, Genotoxicity, and Carcinogenicity of Regulated and Emerging Disinfection by-Products in Drinking Water: A Review and Roadmap for Research, Mutat. Res., 2007, 636(1–3), 178–242, DOI:10.1016/J.MRREV.2007.09.001.
- M. Yang, X. Zhang, Q. Liang and B. Yang, Application of (LC/)MS/MS Precursor Ion Scan for Evaluating the Occurrence, Formation and Control of Polar Halogenated DBPs in Disinfected Waters: A Review, Water Res., 2019, 158, 322–337, DOI:10.1016/J.WATRES.2019.04.033.
- M. Gonsior, P. Schmitt-Kopplin, H. Stavklint, S. D. Richardson, N. Hertkorn and D. Bastviken, Changes in Dissolved Organic Matter during the Treatment Processes of a Drinking Water Plant in Sweden and Formation of Previously Unknown Disinfection Byproducts, Environ. Sci. Technol., 2014, 48(21), 12714–12722, DOI:10.1021/ES504349P/SUPPL_FILE/ES504349P_SI_001.PDF.
- V.-A. Langvik and O. Hormi, Possible Reaction Pathways for the Formation of 3-Chloro-4-(Dichloromethyl)-5-Hydroxy-2(5H)-Furanone (MX), Chemosphere, 1994, 28(6), 1111–1117 CrossRef CAS.
- J. Bonetti, Mass Spectral Differentiation of Positional Isomers Using Multivariate Statistics, Forensic Chem., 2018, 9, 50–61, DOI:10.1016/J.FORC.2018.06.001.
- E. L. Schymanski, J. Jeon, R. Gulde, K. Fenner, M. Ruff, H. P. Singer and J. Hollender, Identifying Small Molecules via High Resolution Mass Spectrometry: Communicating Confidence, Environ. Sci. Technol., 2014, 48(4), 2097–2098, DOI:10.1021/ES5002105.
- Z. Huixian, L. Junhe, C. Zhuo, Y. Chengyong, Z. Jinqi and Z. Wen, Screening the Precursors of Strong Mutagen [3-Chloro-4-(Dichloromethyl)-5-Hydroxy-2(5H)-Furanone] MX from Chlorinated Water, Water Res., 2000, 34(1), 225–229, DOI:10.1016/S0043-1354(99)00106-2.
- J. M. Allen, M. J. Plewa, E. D. Wagner, X. Wei, K. Bokenkamp, K. Hur, A. Jia, H. K. Liberatore, C. F. T. Lee, R. Shirkhani, S. W. Krasner and S. D. Richardson, Drivers of Disinfection Byproduct Cytotoxicity in U.S. Drinking Water: Should Other DBPs Be Considered for Regulation?, Environ. Sci. Technol., 2022, 56(1), 392–402, DOI:10.1021/ACS.EST.1C07998/SUPPL_FILE/ES1C07998_SI_001.PDF.
- M. G. Muellner, E. D. Wagner, K. Mccalla, S. D. Richardson, Y. T. Woo and M. J. Plewa, Haloacetonitriles vs. Regulated Haloacetic Acids: Are Nitrogen-Containing DBFs More Toxic?, Environ. Sci. Technol., 2007, 41(2), 645–651, DOI:10.1021/ES0617441/SUPPL_FILE/ES0617441SI20060728_010501.PDF.
- X. F. Li and W. A. Mitch, Drinking Water Disinfection Byproducts (DBPs) and Human Health Effects: Multidisciplinary Challenges and Opportunities, Environ. Sci. Technol., 2018, 52(4), 1681–1689, DOI:10.1021/ACS.EST.7B05440.
- M. Wahab, M. Zahoor, S. Muhammad Salman, A. W. Kamran, S. Naz, J. Burlakovs, A. Kallistova, N. Pimenov and I. Zekker, Adsorption-Membrane Hybrid Approach for the Removal of Azithromycin from Water: An Attempt to Minimize Drug Resistance Problem, Water, 2021, 13(14), 1969, DOI:10.3390/W13141969/S1.
- C. Chevrier, B. Junod and S. Cordier, Does Ozonation of Drinking Water Reduce the Risk of Bladder Cancer?, Epidemiology, 2004, 15(5), 605–614, DOI:10.1097/01.EDE.0000134866.61780.28.
- G. A. Zoumpouli, Z. Zhang, J. Wenk and C. Prasse, Aqueous Ozonation of Furans: Kinetics and Transformation Mechanisms Leading to the Formation of α,β-Unsaturated Dicarbonyl Compounds, Water Res., 2021, 203, 117487, DOI:10.1016/J.WATRES.2021.117487.
|
This journal is © The Royal Society of Chemistry 2023 |
Click here to see how this site uses Cookies. View our privacy policy here.