Removal of Cl(−I) from acidic industrial wastewater through oxidation: a review on methods and mechanisms
Received
15th August 2022
, Accepted 8th November 2022
First published on 11th November 2022
Abstract
An abundance of acidic wastewater containing high concentrations of Cl(−I) is produced annually in industries. The traditional precipitation, ion exchange and physical separation methods for Cl(−I) removal possess the major limitation of low efficiency, making it necessary to develop new approaches with high efficiency. Recently, the technology of advanced oxidation process (AOPs) and ligand-to-metal charge transfer process (LMCT) has attracted considerable attention for the oxidation removal of Cl(−I), where the various intermediate active species with extremely high oxidability exhibit the ability to oxidize Cl(−I). In this article, the efficiency, mechanisms, advantages, limitations, developmental trends and potential improvements of the electrolysis, UV-induced and strong-oxidant-dominated oxidation approaches for Cl(−I) removal are reviewed in detail. The oxidation approaches exhibit the common advantage of high efficiency. However, the potential secondary pollution of Cl2 gas and the high requirement for wastewater quality limit the actual application. Before the electrolysis and UV-induced oxidation methods can be applied, the energy consumption must be further reduced. Additionally, the slow release and stability of the Na2S2O8, NaBiO3 and PbO2 agents should be further studied in the strong-oxidant-dominated oxidation approaches. This study provides the effective removal of Cl(−I) from acidic industrial wastewater with the theoretical foundation and technical support.
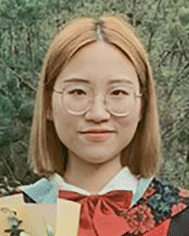 Wenyue Dou | Wenyue Dou (corresponding author) works at Xuzhou University. Her main research interests involve environmental water chemistry of chloride pollutants, the control of water pollution, and resource recovery based on adsorption, oxidation and reduction, coagulation and separation processes of chloride pollutants in water environments. |
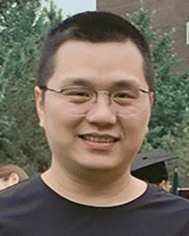 Linghao Kong | Linghao Kong works at the Research Center for Eco-Environmental Sciences, Chinese Academy of Sciences. His main research interests involve the development of technology for heavy metal detection, the control of heavy metal pollution and the reuse of industrial wastewater. |
Water impact
A large quantity of acidic wastewater containing high concentrations of Cl(−I) is produced in industries annually. Cl(−I), the most stable form of the element chlorine in aqueous conditions, is hard to remove by common physicochemical methods. Recently, technology based on AOPs and LMCT processes has attracted considerable attention for the oxidation removal of Cl(−I) from acidic industrial wastewater. In this article, oxidation approaches for Cl(−I) were divided into electrolytic, UV-induced and strong-oxidant-dominated methods. The efficiency, mechanisms, advantages and limitations of the traditional electrolysis methods and the newly-developed Cu(0)/Cu(II)/UV, Bi2O3/UV, Na2S2O8, NaBiO3 and PbO2 methods were reviewed in detail. Finally, the developmental trends and potential improvements for these oxidation-removal approaches of different kinds were discussed separately, providing the theoretical foundation and technical support for the effective removal of Cl(−I) and the purification of industrial wastewater.
|
1. Introduction
Cl(−I), the most stable form of the element chlorine, widely exists in surface waters. Except for the natural sources of seawater intrusion and mineral corrosion, the artificial source of industrial wastewater is also a major source for Cl(−I) in nature.1–3 In industries, numerous materials and additive agents containing Cl(−I) are frequently employed, leading to the generation of acidic wastewater with high concentrations of Cl(−I) and large quantities.4–8 For example, the use of a kind of major additive agent, hydrochloric acid (HCl), results in a high content of Cl(−I) in acidic wastewater produced from the rare earth extraction process.9 The content of the Cl(−I) impurity in the mineral materials has been greatly enhanced in recent years with the continuous exploitation of mineral resources. Thus, the amount of Cl(−I) released during the hydrometallurgical process has also increased. In the strongly acidic wastewater produced from nonferrous smelting industries, the concentration of Cl(−I) can even reach over ten thousand milligrams per liter.
Without the appropriate treatment on the above-mentioned acidic industrial wastewater containing high concentrations of Cl(−I), a sharp increase in the Cl(−I) content of surface waters will be induced. Furthermore, the environment and organisms will be damaged to varying degrees. On the one hand, the discharge of Cl(−I)-containing wastewater may influence the normal growth of organisms. For instance, the accumulation of Cl(−I) in soils can lead to salinization problems, which will then inhibit plant growth and induce crop death due to poisoning.10 Additionally, drinking water with high Cl(−I) concentrations may affect the normal metabolism of animals and lead to dramatic poisoning symptoms.11,12 On the other hand, for the living facilities and industrial settings, Cl(−I) is also extremely unfavorable because of its corrosion function. For example, Cl(−I) in the concrete can lead to the corrosion of steel, the expansion of concrete and the decrease in the compressive capacity, ultimately inducing damages to building structures.13–16 Meanwhile, Cl(−I) possesses the ability of an inter-crystalline, crevice and pitting corrosion on metals through destroying the passive films composed of metallic oxide. As a result, the abnormal operations of equipment and security risks may occur.17–19 Therefore, to avoid the environmental and biological risks caused by the discharge of Cl(−I), the effective removal of Cl(−I) from various acidic industrial wastewater is of particular significance.
Cl(−I) cannot be utilized by microorganisms effectively. Worse still, a high concentration of Cl(−I) will enhance the osmotic pressure, destroy the cell membrane, and inhibit the normal growth of microorganisms. Given this, the removal of Cl(−I) through biological methods seems to be nearly impossible, and physicochemical methods are commonly employed for the removal of Cl(−I). At present, the widely-studied methods for Cl(−I) removal under aqueous conditions can be divided into precipitation, ion exchange, physical separation and electrolysis. Cl(−I) has the strong ability to combine with some specific metal ions or organic compounds to generate precipitates, making precipitation become the most widely used Cl(−I)-removal approach. The major precipitants employed for the removal of Cl(−I) include copper slag, bismuth oxide (Bi2O3), silver nitrate (AgNO3), calcium–aluminum salts and thiourea.17,20–28 Meanwhile, the negative ion charge makes it possible for Cl(−I) to be removed through ion exchange approach using ion exchange resins or layered double hydroxides (LDHs).29–35 In addition, because of the extremely high stability of Cl(−I), removing Cl(−I) without any changes to its ionic form through physical separation methods, including electrosorption, electrodialysis, evaporation concentration and solvent extraction,36–46 has also been widely studied.
As we mentioned above, Cl(−I) possesses extremely high stability because of its fully saturated outer electrons, which indicates that the valence changes of Cl(−I) are rather difficult. At the same time, the electrode potential (φ0) of Cl(−I)/Cl2 is up to approximately 1.36 eV,47 further demonstrating that through general oxidation methods, Cl(−I) is hard to transform into Cl2 gas. Naturally, the electrolysis approach, where the redox reactions can easily occur under the function of a current, was studied for the oxidation removal of Cl(−I) in the form of Cl2 gas in the past few decades.48–51
Although various Cl(−I)-removal methods have been studied and proposed, the common disadvantage of low efficiency in these approaches makes it necessary to develop new Cl(−I)-removal methods with higher efficiency. In addition, the limitations of different Cl(−I)-removal technologies, for instance, the high dosage of precipitants in the precipitation method, the high regeneration frequency of ion exchangers in the ion exchange method, and the demand of the low Cl(−I) concentration in the physical separation method, also show the importance of further research studies on Cl(−I) removal from wastewater.
It can be determined from the φ0 of Cl(−I)/Cl2 that, as long as φ0 of the dosed oxidant and its corresponding reduction product exceeds 1.36 eV, it is probable that Cl(−I) will be oxidized to produce Cl2 gas by this kind of oxidant. Nowadays, along with the rapid development of water treatment technologies, various active species with extremely high oxidability (for instance, hydroxyl (˙OH) radical, sulfate (˙SO4−) radical, photo-induced holes (h+), Pb(IV) and Bi(V)) have begun to attract much attention for the oxidation-removal process of Cl(−I) with high concentration. Accordingly, the technology of advanced oxidation process (AOPs) and ligand-to-metal charge transfer process (LMCT) was systematically studied for the removal of Cl(−I) from acidic industrial wastewater, and was proved to be effective.
In this article, the oxidation approaches for Cl(−I) removal are divided into electrolysis, ultraviolet (UV)-induced and strong-oxidant-dominated methods, as shown in Fig. 1. The research studies on the oxidation-removal approaches of Cl(−I) from acidic industrial wastewaters, including the traditional electrolysis methods, the newly developed Cu(0)/Cu(II)/UV, Bi2O3/UV, sodium persulfate (Na2S2O8), sodium bismuthate (NaBiO3) and lead dioxide (PbO2) methods, were reviewed in detail. The corresponding technology, mechanisms, Cl(−I)-removal efficiency, advantages and limitations were all systematically analyzed. Finally, the developmental trends and potential improvements for the oxidation-removal approaches of Cl(−I) in acidic industrial wastewater were discussed separately, providing the effective removal of Cl(−I) under aqueous conditions and the purification of industrial wastewater with a theoretical foundation and technical support.
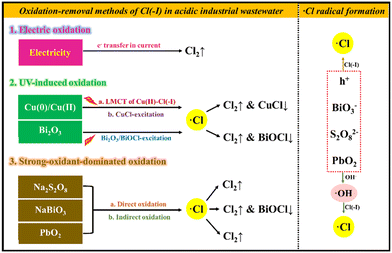 |
| Fig. 1 Oxidation approaches for Cl(−I) removal from acidic wastewater. | |
2. Oxidation-removal methods of Cl(−I)
During the treatment of acidic Cl(−I)-containing wastewater using the oxidation-removal approaches, Cl(−I) can be oxidized to generate Cl2 gas under the function of an electric current or strong oxidants. Additionally, the chlorine (˙Cl) radical, a kind of intermediate species produced with the help of various active species, including h+, ˙OH radical, ˙SO4− radical, Pb(IV) and Bi(V), exhibits the ability to directly combine with some coexisting solids to produce precipitates, resulting in the effective removal of Cl(−I) from acidic industrial wastewaters through two paths of oxidation and precipitation.
2.1. Electrolysis oxidation method
As shown in Fig. 2, during the treatment of wastewater containing Cl(−I) conducted in an electrolytic cell, the directional movement of Cl(−I) to the anode under the driving of potential difference (PD) firstly occurs between the cathode and anode. Then, Cl(−I) will be oxidized to produce Cl2 gas on the anode according to eqn (1). Simultaneously, many H+ ions in the solutions will move to the cathode and be reduced, leading to the formation of H2 gas as shown in eqn (2). For the wastewater containing a high concentration of metal ions (Mx+), Mx+ will replace H+ to be reduced by electrons (e−), and the generated zero-valent metal will deposit gradually on the cathode according to eqn (3).49 | 2Cl(−I) − 2e− → Cl2↑ (on the anode) | (1) |
| 2H+ + 2e− → H2↑ (on the cathode) | (2) |
| Mx+ + xe− → M↓ (on the cathode) | (3) |
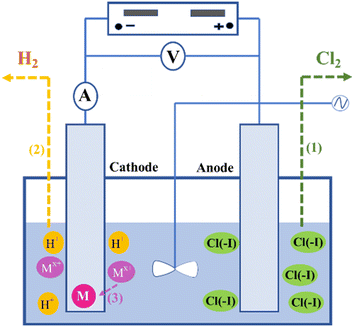 |
| Fig. 2 Mechanisms for Cl(−I) removal through the electrolysis oxidation process. | |
During the treatment using the electrolysis method, the removal efficiency of Cl(−I) depends on the electrolytic conditions of the wastewater composition, electrolytic cell structure, electrode material, current density and power duration, among which the electrode material is an important factor for the Cl(−I)-removal process. The study on the removal of Cl(−I) from zinc sulfate aqueous solutions showed that, after 180 min of dichlorination conducted under the applied voltage of 0.6 V and the ultrasonic agitation power of 50 W, 54.5% of Cl(−I) with the initial concentration of 300 mg L−1 can be removed by the copper electrode.50 In addition, a kind of ruthenium–titanium–activated carbon (Ru–Ti–AC) combined anode electrode was confirmed to exhibit excellent Cl(−I)-removal performance during the electrolysis process. For the desulfurization wastewater containing 12
000 mg L−1 of Cl(−I), 80% of Cl(−I) can be removed under the current density of 400 mA after 60 min.51 Recently, a kind of porous Ni–Ce-doped lead dioxide (Ni–Ce-doped PbO2) electrode was applied on the electrolysis removal process of Cl(−I), and was verified to have excellent performance. Under a current intensity of 50 mA, a Cl(−I)-removal efficiency of approximately 87.4% can be achieved after 90 min of treatment on the simulated wastewater with an initial Cl(−I) concentration of 4000 mg L−1.49
Owing to the high Cl(−I)-removal efficiency, strong stability, wide suitable acidity range and the avoidance of introducing secondary impurities, the electrolysis method can be well applied to the treatment of acidic wastewater with high Cl(−I) concentrations. However, the long-time duration of power consumption directly leads to the high cost, thus limiting the application scope to the treatment of wastewater at a small volume. Additionally, coexisting ions with higher reducibility than Cl(−I) in the wastewater, including Fe(II), I(−I) and Br(−I), can be oxidized on the anode preferentially. Thus, the electrolysis approach is not suitable for acidic wastewater containing multiple impurities.
2.2. UV-induced oxidation methods
In the traditional Cl(−I)-removal precipitation methods using copper slag and Bi2O3, the involved solids of cuprous chloride (CuCl), Bi2O3 and bismuth oxychloride (BiOCl) are all excellent photocatalysts that can be excited under UV light to produce active species.52–59 These active species with strong oxidability make it possible for Cl(−I) to be oxidized and removed using copper slag and Bi2O3 under UV light. In view of this, the mechanisms and efficiency of Cl(−I) removal from acidic industrial wastewater under UV light began to attract much attention.
2.2.1 Cu(0)/Cu(II)/UV oxidation & precipitation method.
In the traditional copper slag precipitation method for Cl(−I) removal, comproportionation first happens between Cu(II) and Cu(0) contained in the copper slag with the presence of Cl(−I). Then, the generated Cu(I) will precipitate with Cl(−I) to produce CuCl solid according to eqn (4), ultimately leading to the removal of Cl(−I) through the precipitation process.20–22 The copper slag precipitation method has been widely used in zinc electrolysis and metallurgical industries for Cl(−I) removal. Despite this, the limited efficiency, high dosage of copper slag and the high residual concentration of Cu(II) have always resulted in the rather high cost, agent waste and the risks of secondary pollution, indicating that further improvement on this approach is necessary. | Cu(0) + Cu(II) + 2Cl(−I) → 2CuCl (Solid) | (4) |
In recent research, an improved approach described as Cu(0)/Cu(II)/UV oxidation & precipitation method was proposed for Cl(−I) removal. Because of the involvement of UV light, the mechanisms change completely for Cl(−I) removal, where the ˙Cl radical is a kind of critical intermediate substance. According to the proposed mechanisms shown in Fig. 3, the ˙Cl radical can form in two pathways. One pathway is that the LMCT occurs in the complex compound of Cu(II) and Cl(−I) ([Cu(II)Clx]2−x) under UV light to produce the ˙Cl radical (eqn (5)). The other is that the ˙Cl radical forms from the oxidization reaction of Cl(−I) by h+ and ˙OH radical, the products of CuCl photocatalysis process under UV light (eqn (6)–(9)). Next, the generated ˙Cl radical can directly combine with Cu(0) to produce CuCl as shown in eqn (10), leading to the removal of Cl(−I) in the form of CuCl precipitate. Meanwhile, the ˙Cl radical can also rapidly combine to produce Cl2 gas (eqn (11)), which is another form of the removed Cl(−I).60 Overall, in this improved approach, owing to the formation of the ˙Cl radical in the LMCT of [Cu(II)Clx]2−x and the photocatalysis process of CuCl, Cl(−I) can be removed in the form of CuCl precipitate and Cl2 gas through oxidation and precipitation processes.
|  | (5) |
|  | (6) |
| Cl(−I) + ˙OH → OH− + ˙Cl | (8) |
| Cu(0) + ˙Cl → CuCl (Solid) | (10) |
| ˙Cl + ˙Cl → Cl2 (Gas) | (11) |
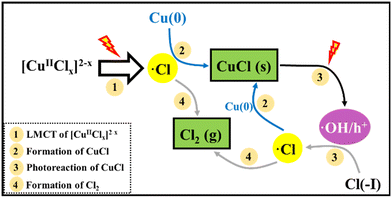 |
| Fig. 3 Mechanisms for Cl(−I) removal through the Cu(0)/Cu(II)/UV oxidation & precipitation process. | |
As reported in the relevant study, after 40 min of treatment under UV light provided by a Hg lamp (28 W), 87.9% of Cl(−I) was effectively removed from the actual acidic zinc smelting wastewater with an initial Cl(−I) concentration of 428.8 mg L−1.60 Compared with the traditional method, the mole ratio of Cu(II)/Cl(−I) was reduced from 8
:
1 to 2
:
1, indicating that the dosage of copper slag and the residual Cu(II) can be greatly reduced. Despite the high efficiency and low copper slag dosage, the high energy consumption and complex operation make this approach far from practical. For instance, the layout and maintenance of UV lamps, the handling of the generated Cl2 gas and the further removal of residual Cu(II) will bring some difficulties to practical operation.
2.2.2 Bi2O3/UV oxidation & precipitation method.
In the traditional Bi2O3 precipitation method for Cl(−I) removal, Bi2O3 is firstly dissolved to release Bi(III) in the presence of H+ (eqn (12)). Then, Bi(III) continues to react with Cl(−I) and H2O to produce BiOCl (eqn (13)), finally resulting in the removal of Cl(−I) in the form of BiOCl precipitate.23–25 Bi2O3 exhibits excellent Cl(−I)-removal performance. However, this approach needs a rather strong acidity of wastewater owing to the dissolution process of Bi2O3, the most critical reaction determining the Cl(−I)-removal efficiency. Moreover, the high dosage of Bi2O3 also limits the further application of this method during actual wastewater treatment. Therefore, an improvement was proposed to solve the abovementioned problems. | Bi2O3 + 6H+ → 2Bi(III) + 3H2O | (12) |
| Bi(III) + H2O + Cl(−I) → 2H+ + BiOCl (Solid) | (13) |
Owing to the excellent photocatalysis performance of the Bi2O3 agent and BiOCl product, new reactions occur under UV light and help the removal of Cl(−I). During the treatment of wastewater through the recently proposed Bi2O3/UV oxidation & precipitation method as shown in Fig. 4, the dissolved Bi(III) is firstly produced from Bi2O3 under the action of both sulphuric acid (H2SO4) and UV light. Next, Bi(III) combines with Cl(−I) and hydrolyzes to BiOCl on the surface of Bi2O3, leading to the formation of the Bi2O3/BiOCl heterojunction, which transforms to Bi2O3(e−)/BiOCl(h+) under the excitation by UV light. Then, h+ in the VB of BiOCl oxidizes OH− to ˙OH radical. Simultaneously, the ˙Cl radical is produced from Cl(−I) under the oxidation function of both ˙OH radical and h+ according to eqn (7)–(9). Finally, BiOCl will form directly from Bi2O3, e− and ˙Cl radical as shown in eqn (14).61 After the wastewater treatment, Bi2O3 is regenerated from BiOCl using sodium hydroxide (NaOH) solution as shown in eqn (15). As a result, Cl(−I) is ultimately transferred from the wastewater to the sodium chloride/sodium hydroxide (NaCl/NaOH) concentrate. In general, because of the photocatalysis process of the Bi2O3/BiOCl heterojunction under UV light, the ˙Cl radical can be produced effectively and play an important role in removing Cl(−I) in the form of Cl(−I)-containing concentrate.
| Bi2O3 + 6H+ → 2Bi(III) + 3H2O | (14) |
| 2BiOCl + 2NaOH → Bi2O3 + 2NaCl + H2O | (15) |
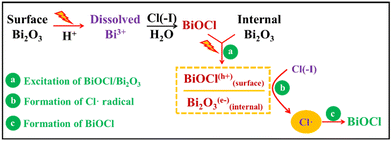 |
| Fig. 4 Mechanisms for Cl(−I) removal through the Bi2O3/UV oxidation & precipitation process. | |
For the actual acidic nonferrous smelting wastewater containing 10
400 mg L−1 of Cl(−I), a high efficiency of 98.1% was reached after 80 min of treatment under UV light with the energy consumption being calculated to be 11.2 kW h m−3. After further treatment on BiOCl using NaOH solution, approximately 97.4% of Bi2O3 was regenerated and reused in the next batch of Cl(−I)-removal experiments.61 Because of the addition of UV light, the mole ratio of dosed Bi2O3 to Cl(−I) was lowered from 1.5
:
1 to 0.5
:
1. Meanwhile, the required initial H2SO4 concentration of the wastewater was also lowered from 70 to 40 g L−1, signifying that the traditional Bi2O3 precipitation method has been significantly improved. Additionally, the regeneration of Bi2O3 greatly lowered the cost of the agent. However, the high energy consumption and complex operation brought by the employment of UV lamps limited further research on this approach.
2.3. Strong-oxidant-dominated oxidation methods
To avoid the complex operation and high energy consumption brought from the employment of UV light, it becomes meaningful to search for a kind of new strong oxidant exhibiting the ability to directly oxidize Cl(−I) or to induce the generation of an active species for Cl(−I) removal without the addition of UV light.
2.3.1 Na2S2O8 oxidation method.
The φ0 of SO42−/S2O82− is 2.01 eV,62,63 which is much higher than that of Cl(−I)/Cl2. In addition, Na2S2O8 can be activated by heating, acid or metal ions to generate ˙SO4− radical with a redox potential of 2.5–3.1 eV,64–67 indicating that the oxidation of Cl(−I) by Na2S2O8 can be realized theoretically. In the study on the Cl(−I) removal by Na2S2O8, the involved mechanisms (Fig. 5) were described as follows. Firstly, S2O82− decomposes to produce the ˙SO4− radical under the activation of heating according to eqn (16). Then, the sulfate (˙HSO4) radical will form in the combination reaction between the ˙SO4− radical and H+ and oxidize OH− to form the ˙OH radical (eqn (17) and 18). Under the function of the ˙SO4− and ˙OH radicals, the ˙Cl and chlorine (˙Cl2−) radicals will thus form from Cl(−I) as shown in eqn (8), (19) and (20).68 Finally, owing to the self-combination process, Cl2 gas can be produced from the ˙Cl and ˙Cl2− radicals according to eqn (11) and (21), resulting in the removal of Cl(−I). |  | (16) |
| ˙HSO4 + H2O → ˙OH + HSO4− + H+ | (18) |
| Cl(−I) + ˙SO4− → ˙Cl + SO42− | (19) |
| ˙Cl2− + ˙Cl2− → 2Cl− + Cl2 (Gas) | (21) |
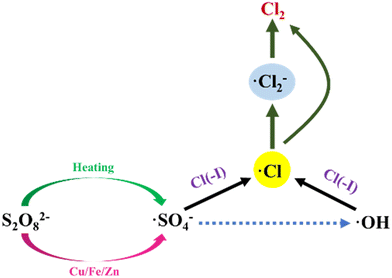 |
| Fig. 5 Mechanisms for Cl(−I) removal through Na2S2O8 oxidation process. | |
In the treatment of actual strongly acidic wastewater containing various metal ions and 1000 mg L−1 of Cl(−I), where the mole ratio of dosed Na2S2O8 to Cl(−I) was 0.6
:
1, a high efficiency of 96–98% can be achieved at 25 min under 70 °C with the residual Cl(−I) concentration below 158 mg L−1. Additionally, the removal efficiency of Cl(−I) for the actual wastewater was 8–10% higher than that for the simulated wastewater. This probably resulted from the activation function of metal ions on Na2S2O8 to produce the ˙SO4− radical as shown in eqn (22). This novel Cl(−I)-removal approach using Na2S2O8 shows the advantages of high efficiency and the avoidance to introducing foreign ions. However, Na2S2O8 can easily decompose with the presence of H2O as shown in eqn (23), indicating that the dry, airtight and dark storage conditions for Na2S2O8 are required. Meanwhile, once Na2S2O8 is added to the wastewater, the violent reaction between Na2S2O8 and H2O makes the decomposition of Na2S2O8, leading to the waste of the agent.
| S2O82− + Mx+ → Mx+1 + ˙SO4− + SO42− | (22) |
| 2Na2S2O8 + 2H2O → 2Na2SO4 + 2H2SO4 + O2 (Gas) | (23) |
2.3.2 NaBiO3 oxidation & precipitation method.
The compounds of Bi(V) possess extremely high oxidability under acidic conditions. Furthermore, the φ0 of Bi(III)/bismuthate (Bi(III)/BiO3−) is 1.59 eV,69 which is higher than that of Cl(−I)/Cl2, signifying the possibility for Cl(−I) to be oxidized by NaBiO3 under acidic conditions. According to the proposed mechanisms for Cl(−I) removal from strongly acidic wastewater using NaBiO3 shown in Fig. 6, Cl(−I) can be removed in the form of Cl2 gas and BiOCl precipitate.70 After being dosed, NaBiO3 will be dissolved gradually to produce BiO3−, which further oxidizes Cl(−I) and OH− to generate ˙Cl and ˙OH radicals, respectively (eqn (24) and (25)). Simultaneously, the ˙Cl radical can also form from the oxidation of Cl(−I) by the ˙OH radical (eqn (8)). Thus, the abundant ˙Cl radical will self-combine to produce Cl2 gas (eqn (11)). In addition, dissolved Bi(III), the reduction product of BiO3−, will react with Cl(−I) and H2O to produce the BiOCl solid as shown in eqn (13). As a result, Cl(−I) will be efficiently removed through two paths of oxidation by BiO3− and precipitation by Bi(III). | BiO3− + 2Cl(−I) + 6H+ → Bi(III) + 2˙Cl + 3H2O | (24) |
| BiO3− + 4H+ → Bi(III) + 2˙OH + H2O | (25) |
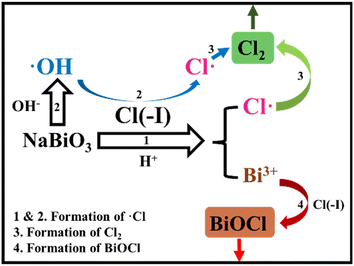 |
| Fig. 6 Mechanisms for Cl(−I) removal through the NaBiO3 oxidation & precipitation process. | |
Through the treatment on actual strongly acidic smelting wastewater (10 L) containing 2128 mg L−1 of Cl(−I) using NaBiO3, approximately 98.5% of Cl(−I) was effectively removed at a NaBiO3 to Cl(−I) mole ratio of 1
:
3 at 180 min under 30 °C. Furthermore, the generated Cl2 gas was continuously absorbed by NaOH solution to avoid the secondary pollution and produce sodium hypochlorite (NaClO) for the regeneration of NaBiO3 (eqn (26)). After the regeneration process of NaBiO3 from BiOCl using the absorption solution for Cl2 gas, 94.3% of the dosed NaBiO3 agent was regenerated and reused, and Cl(−I) was finally transferred from acidic wastewater into the NaCl/NaOH concentrate.70 This approach has the major advantages of high efficiency and low cost for the regenerative agent. Owing to the regenerative ability, NaBiO3 shows greater potential for the realization of practical application on Cl(−I) removal in a short time than Na2S2O8. In view of the decomposability under high temperature and the reactivity with H2O of NaBiO3, the strict cryogenic, dry and dark storage conditions of the agent are also necessary to this approach.
| BiOCl + 2NaOH + NaClO → 2NaCl + NaBiO3 + H2O | (26) |
2.3.3 PbO2 oxidation method.
Pb(IV) with strong oxidability possesses a Pb(IV)/Pb(II) φ0 value of 1.69 eV under acidic conditions,71 which is higher than the 1.36 eV φ0 value of Cl(−I)/Cl2. In the newly proposed approach for Cl(−I) removal using a PbO2 agent,72 the ˙Cl radical can form through two pathways of surface oxidation and ˙OH radical oxidation according to the following mechanisms (Fig. 7). In the former surface oxidation process, the
Pb(IV)–Cl(−I) complex firstly forms because of the absorption of Cl(−I) on the surface of PbO2 as shown in eqn (27). The two-step LMCT then happens in the
Pb(IV)–Cl(−I) complex, where two e− transfer from Cl(−I) to Pb(IV) along the covalent bond successively, leading to the generation of the ˙Cl radical and
Pb(II) (eqn (28) and (29)). Meanwhile, in the ˙OH radical oxidation process, PbO2 first oxidizes OH− to produce the ˙OH radical as shown in eqn (30), which can further oxidize Cl(−I) and form the ˙Cl radical (eqn (8)). Ultimately, many of these ˙Cl radicals will self-combine, leading to the removal of Cl(−I) in the form of Cl2 gas. Pb(II), the reduction product of Pb(IV), will react with the coexisting SO42− and be precipitated in the form of lead sulfate (PbSO4) solid. | Pb(IV)–OH− + Cl(−I) + H+ → Pb(IV)–Cl(−I) + ˙Cl | (27) |
| Pb(IV)–Cl(−I) → Pb(III) + ˙Cl | (28) |
| Pb(III)–Cl(−I) → Pb(II) + ˙Cl | (29) |
| PbO2 + 2H+ → Pb(II) + 2˙OH | (30) |
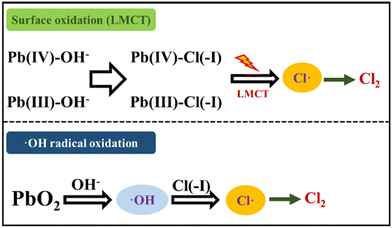 |
| Fig. 7 Mechanisms for Cl(−I) removal through the PbO2 oxidation process. | |
During the treatment of the simulated wastewater containing 1000 mg L−1 of Cl(−I) and 200 g L−1 of H2SO4, a high Cl(−I)-removal efficiency of 93.4% was obtained at the PbO2/Cl(−I) mole ratio of 2
:
1 under 50 °C at 30 min. Additionally, the PbO2 agent was regenerated from PbSO4 using the NaOH absorption solution of Cl2 gas according to eqn (31). After six-times regeneration and reuse, PbO2 still showed excellent Cl(−I)-removal ability with the efficiency above 90%.72 PbO2 shows the major advantages of high efficiency and regenerative ability. Additionally, the much lower price of PbO2 compared to that of NaBiO3 brings PbO2 greater advantages in the treatment cost. However, similar to NaBiO3, PbO2 easily decomposes under high temperature, which indicates that the strict dry and dark storage conditions of the agent are essential in this approach. Meanwhile, the precipitation of Pb(II) with SO42− will lead to the waste of H2SO4, a kind of valuable resource in the strongly acidic wastewater.
| PbSO4 + 2NaOH + NaClO → Na2SO4 + PbO2 + NaCl + H2O | (31) |
3. Conclusions and perspectives
In this article, the three major oxidation means for Cl(−I) removal from acidic industrial wastewater, including electricity, UV light and strong oxidant, are reviewed in detail. Table 1 summarizes the advantages, limitations and potential improvements of these oxidation-removal approaches for Cl(−I) in the acidic industrial wastewater as follows.
Table 1 Oxidation-removal approaches for Cl(−I) from acidic industrial wastewater
Methods |
Mechanisms |
Advantages |
Disadvantages |
Potential improvements |
Electrolysis |
Oxidation by electric current |
Strong stability |
High cost |
Reducing of energy consumption |
Wide suitable acidity range |
Avoidance of secondary impurities |
|
UV-induced oxidation methods |
Cu(0)/Cu(II) + UV |
Oxidation & precipitation by LMCT & AOPs |
Low dosage of copper slag |
High energy consumption |
Complex operation |
Bi2O3 + UV |
Oxidation & precipitation by AOPs |
Low dosage of Bi2O3 |
High energy consumption |
Regenerative ability of Bi2O3 |
Complex operation |
|
Strong-oxidant-dominated oxidation methods |
Na2S2O8 + heating |
Oxidation by AOPs |
Avoidance to foreign ions |
High cost for Na2S2O8 |
Stabilization and slow releasing of reagents |
Low energy consumption |
Strict storage conditions of Na2S2O8 |
NaBiO3 + heating |
Oxidation & precipitation by AOPs |
Low energy consumption |
Strict storage conditions of NaBiO3 |
Regenerative ability of NaBiO3 |
High price of NaBiO3 |
PbO2 + heating |
Oxidation by LMCT & AOPs |
Low energy consumption |
Strict storage conditions of PbO2 |
Regenerative ability of PbO2 |
Waste of valuable H2SO4 resource |
Low price of PbO2 |
1. All of the oxidation methods for Cl(−I) removal exhibit the advantage of high efficiency. Given this, these oxidation means are likely to be employed for the treatment of wastewater containing extremely high concentrations of Cl(−I). Despite this, some Cl2 gas will be produced and may induce secondary pollution without appropriate treatment countermeasures. Meanwhile, to avoid the waste of agents and the generation of byproducts, the oxidation technology is unsuitable for the wastewater containing multiple reducing substances;
2. The high energy consumption of current and UV light leads to the high cost of electrolysis and UV-induced oxidation methods. More research studies to lower the cost (for instance, determining a suitable UV lamp type and layout, optimized electrolysis cell configuration and modified electrode material) are required before the actual application;
3. The slow-release technology of the Na2S2O8, NaBiO3 and PbO2 agents must be further developed to avoid the agent waste and the safety risks. For instance, the porous supporting skeleton and hard shell can be considered to be applied on the modification of the Cl(−I)-removal agents for the sustained release effect.
In the field of industrial wastewater treatment, the removal of Cl(−I) has always been a technical challenge. Although many methods have been developed for the removal of Cl(−I) in recent decades, the traditional precipitation approach using copper slag is still the most widely applied one because of the low cost and easy operation. In this case, the newly developed oxidation methods show the major advantage of high efficiency. Before the application of these oxidation methods, a series of practical problems along with the removal of Cl(−I), including the high energy consumption, the strict storage conditions of reagents and the absorption of Cl2 gas, need to be taken into consideration.
Conflicts of interest
The authors declare no competing financial interests.
Acknowledgements
This work was supported by the Scientific Research Foundation for the PhD (Xuzhou University of Technology, No. 02900284). We are grateful to the editors and anonymous reviewers for their valuable comments and suggestions for our paper.
References
- M. K. Steele and J. A. Aitkenhead-Peterson, Long-term sodium and chloride surface water exports from the Dallas/Fort Worth region, Sci. Total Environ., 2011, 409, 3021–3032 CrossRef CAS PubMed.
- M. C. Boufadel, A mechanistic study of nonlinear solute transport in a groundwater-surface water system under steady state and transient hydraulic conditions, Water Resour. Res., 2000, 36, 2549–2565 CrossRef CAS.
- I. D. Jolly, K. L. McEwan and K. L. Holland, A review of groundwater-surface water interactions in arid/semi-arid wetlands and the consequences of salinity for wetland ecology, Ecohydrology, 2008, 1, 43–58 CrossRef CAS.
- E. Ennaassia, K. Elkacemi, A. Kossir and G. Cote, Study of the removal of Cd(II) from phosphoric acid solutions by precipitation of CdS with Na2S, Hydrometallurgy, 2002, 64, 101–109 CrossRef CAS.
- R. W. Hammack, H. M. Edenborn and D. H. Dvorak, Treatment of water from an open-pit copper mine using biogenic sulfide and limestone: A feasibility study, Water Res., 1994, 28, 2321–2329 CrossRef CAS.
- T. Luo, J. Cui, S. Hu, Y. Huang and C. Jing, Arsenic removal and recovery from copper smelting wastewater using TiO2, Environ. Sci. Technol., 2010, 44, 9094–9098 CrossRef CAS.
- S. Kumar, R. C. Gupta, S. Shrivastava and L. Csetenyi, Sustainable utilization of quartz sandstone mining wastes: Chloride and corrosion resistance, J. Mater. Civ. Eng., 2019, 31, 6018026–6018029 CrossRef CAS.
- A. H. Kaksonen, M. L. Riekkola-Vanhanen and J. A. Puhakka, Optimization of metal sulphide precipitation in fluidized-bed treatment of acidic wastewater, Water Res., 2003, 37, 255–266 CrossRef CAS.
- W. Zhang, D. Feng, X. Xie, X. Tong, Y. Du and Y. Cao, Solvent extraction and separation of light rare earths from chloride media using HDEHP-P350 system, J. Rare Earths, 2022, 40, 328–337 CrossRef CAS.
- E. Shoukat, M. Z. Ahmed, Z. Abideen, M. Azeem, M. Ibrahim, B. Gul and M. A. Khan, Short and long term salinity induced differences in growth and tissue specific ion regulation of Phragmites karka, Flora, 2020, 263, 151550–151559 CrossRef.
- C. G. Beebe, R. Schemmel and O. Mickelsen, Blood pressure of rats as affected by diet and concentration of NaCl in drinking water, Proc. Soc. Exp. Biol. Med., 1976, 151, 395–399 CrossRef CAS PubMed.
- A. D. Wilson, The tolerance of sheep to sodium chloride in food or drinking water, Aust. J. Agric. Res., 1966, 17, 503–514 CrossRef CAS.
- M. Thomas, Chloride thresholds in marine concrete, Cem. Concr. Res., 1996, 26, 513–519 CrossRef CAS.
- D. Chen, G. Sun, D. Hu and J. Shi, Study on the bearing capacity and chloride ion resistance of RC structures under multi-factor corrosive environment and continuous load, J. Build. Eng., 2021, 44, 102990–103000 CrossRef.
- Z. Liu, Y. Wang, J. Wang, C. Liu, J. Jiang and H. Li, Experiment and simulation of chloride ion transport and binding in concrete under the coupling of diffusion and convection, J. Build. Eng., 2022, 45, 103610–103625 CrossRef.
- L. Zeng and R. Song, Controlling chloride ions diffusion in concrete, Sci. Rep., 2013, 3, 3359–3365 CrossRef.
- T. Li, J. Lin and J. Liu, Analysis of time-dependent seismic fragility of the offshore bridge under the action of scour and chloride ion corrosion, Structures, 2020, 28, 1785–1801 CrossRef.
- A. El Warraky, H. A. El Shayeb and E. M. Sherif, Pitting corrosion of copper in chloride solutions, Anti-Corros. Methods Mater., 2004, 51, 52–61 CrossRef CAS.
- L. Niu and K. Nakada, Effect of chloride and sulfate ions in simulated boiler water on pitting corrosion behavior of 13Cr steel, Corros. Sci., 2015, 96, 171–177 CrossRef CAS.
- Z. Peng, J. Kong and Y. Liao, Experimental study on dichlorination of copper slag from zinc chloride leaching solution and its application, Youse Jinshu Kexue Yu Gongcheng, 2020, 11, 154–160 CAS.
- Z. Luo, H. Wang, W. Xie, Z. Yang and Y. Li, Study on the chlorine removal by copper slag in the zinc hydrometallurgy, Zhongguo Youse Yejin, 2020, 49, 17–20 Search PubMed.
- F. Ma, Experiment on wet dichlorination and comprehensive recovery of zinc oxide for melting and casting dust collection, Zhongguo Youse Yejin, 2022, 51, 113–118 Search PubMed.
- H. Liu, S. Lei, J. Zhang, Y. Zhao, W. Peng, W. Jiang and P. Li, Study on the industrialization application of bismuth removal in zinc hydrometallurgy system, Zhongguo Youse Yejin, 2018, 47, 29–32 Search PubMed.
- Z. Feng, S. Ning, W. Wang, Z. She, H. Wan and J. Wu, Dechlorination of zinc sulfate solution by bismuth oxide, Kuangye Gongcheng, 2015, 35, 63–66 CAS.
- W. Wu, J. Liu, S. Tian, Z. Wang and Z. Su, Removal of chloride from zinc electrolyte with Bi2O3, Shifa Yejin, 2014, 33, 480–482 CAS.
- T. Wajima, T. Shimizu, T. Yamato and Y. Ikegami, A study on seawater treatment using AgNO3 and Pb(NO3)2, Ocean Thermal Energy Conversion, 2008, 13, 75–79 Search PubMed.
- J. Peng, L. Li, J. Jiang, T. Yang and Y. Yang, Study on removal of high-concentration chloride in wastewater by co-precipitation, Jingshui Jishu, 2019, 38, 95–101 Search PubMed.
- P. Guo, L. Kong, X. Hu, X. Peng and X. Wang, Removal of Cl(−I) from strongly acidic wastewater containing Cu(II) by complexation-precipitation using thiourea: Efficiency enhancement by ascorbic acid, J. Hazard. Mater., 2021, 402, 123836–123844 CrossRef CAS PubMed.
- L. Hua, S. Lu, D. Zhao, L. Lu and Q. Zhang, Static experimental study of using ion exchange to remove chloride in refractory refinery wastewater, Wujiyan Gongye, 2015, 47, 66–69 Search PubMed.
- J. Dron and A. Dodi, Comparison of adsorption equilibrium models for the study of Cl−, NO3− and SO42− removal from aqueous solutions by an anion exchange resin, J. Hazard. Mater., 2011, 190, 300–307 CrossRef CAS PubMed.
- F. Sun, W. Liu, K. Yan and Y. E, Removal of chloride ion from desulfurization wastewater by ion-exchange resin, Wujiyan Gongye, 2019, 51, 45–48 Search PubMed.
- S. Miyata and T. Hirose, Physico-chemical properties of synthetic hydrotalcites in relation to composition, Clays Clay Miner., 1980, 28, 50–56 CrossRef CAS.
- L. Lv, P. Sun, Z. Gu, H. Du, X. Pang, X. Tao, R. Xu and L. Xu, Removal of chloride ion from aqueous solution by ZnAl-NO3 layered double hydroxides as anion-exchanger, J. Hazard. Mater., 2008, 161, 1444–1449 CrossRef PubMed.
- T. Kameda, Y. Miyano, T. Yoshioka, M. Uchida and A. Okuwaki, New treatment methods for waste water containing chloride ion using magnesium - aluminum oxide, Chem. Lett., 2000, 29, 1136–1137 CrossRef.
- A. E. Al-Rawajfeh, E. M. Al-Shamaileh, K. Al-Whoosh, A. Al-Ma'abrah, R. Al-Zorqan, R. Zanoon, K. Rawajfeh and S. Al-Jufout, Adsorption desalination of chloride ions on composite natural-synthetic materials: An approach for the reduction of chlorine corrosion in electrodeionization units, J. Ind. Eng. Chem., 2013, 19, 1895–1902 CrossRef CAS.
- Y. Burak, D. Andelman and H. Orland, Test-charge theory for the electric double layer, Phys. Rev. E: Stat., Nonlinear, Soft Matter Phys., 2004, 70, 16102–16118 CrossRef.
- B. B. Damaskin, Modeling of co-adsorption of cations and anions localized in different layers of the dense part of electrical double layer under the conditions of linear charge dependence of the adsorption energy, Russ. J. Electrochem., 2011, 47, 988–994 CrossRef CAS.
- Z. Shi and J. Lipkowski, Chloride adsorption at the Au(111) electrode surface, J. Electroanal. Chem., 1996, 403, 225–239 CrossRef.
- B. Chen, C. Jiang, Y. Wang, R. Fu, Z. Liu and T. Xu, Selectrodialysis with bipolar membrane for the reclamation of concentrated brine from RO plant, Desalination, 2018, 442, 8–15 CrossRef CAS.
- C. Jiang, Y. Wang, Z. Zhang and T. Xu, Electrodialysis of concentrated brine from RO plant to produce coarse salt and freshwater, J. Membr. Sci., 2014, 450, 323–330 CrossRef CAS.
- O. S. Burheim, F. Seland, J. G. Pharoah and S. Kjelstrup, Improved electrode systems for reverse electro-dialysis and electro-dialysis, Desalination, 2012, 285, 147–152 CrossRef CAS.
- G. Zago, F. M. Penha and M. M. Seckler, Product characteristics in simultaneous crystallization of NaCl and CaSO4 from aqueous solution under different evaporation rates, Desalination, 2019, 457, 85–95 CrossRef CAS.
- J. Liu, Z. Yin, W. Liu, X. Li and Q. Hu, Treatment of aluminum and fluoride during hydrochloric acid leaching of lepidolite, Hydrometallurgy, 2020, 191, 105222–105230 CrossRef CAS.
- D. Wang, W. Du, P. Cheng, N. Tang and X. Wang, Evaporative crystallization of salts from electrodialysis concentrated brine at atmospheric and subatmospheric pressures, IOP Conf. Ser.: Earth Environ. Sci., 2018, 121, 22027–22032 CrossRef.
- K. J. Hasegawa, Y. K. Kimura and T. K. K. Ono, Chloride cofactor in the photosynthetic oxygen-evolving complex studied by fourier transform infrared spectroscopy, Biochemistry, 2002, 41, 13839–13850 CrossRef CAS PubMed.
- L. Zhang, P. Lv, Y. He, S. Li, K. Chen and S. Yin, Purification of chlorine-containing wastewater using solvent extraction, J. Cleaner Prod., 2020, 273, 122863–122871 CrossRef CAS.
-
J. G. Speight, Lange's handbook of chemistry, McGraw-Hill Professional Publishing, New York, 2004 Search PubMed.
- X. Wang, C. Wang, H. Dai, Y. Lin, H. Hua and B. Shu, Treatment of spent acid containing chlorine and heavy metals by membrane electrolysis technology, Huagong Huanbao, 2014, 34, 454–457 Search PubMed.
- S. Liu, L. Gui, R. Peng and P. Yu, A novel porous Ni, Ce-doped PbO2 electrode for efficient treatment of chloride ion in wastewater, Processes, 2020, 8, 466–475 CrossRef CAS.
- X. Wu, Z. Liu and X. Liu, Chloride ion removal from zinc sulfate aqueous solution by electrochemical method, Hydrometallurgy, 2013, 134–135, 62–65 CrossRef CAS.
- S. Liu, Q. Wang, S. Xiao, K. Zhu, J. Qv and H. Wang, Removal of chloride ion in high salt wastewater by Ru-Ti-AC composite catalytic materials, Huaxue Gongcheng Yu Jishu, 2017, 7, 215–224 Search PubMed.
- L. A. O'Reilly, F. O. Lucas, P. Mcnally, A. Reader, G. Natarajan, S. Daniels, D. C. Cameron, A. Mitra, M. E. Martinez-Rosas and L. Bradley, Room-temperature ultraviolet luminescence from γ-CuCl grown on near lattice-matched silicon, J. Appl. Phys., 2005, 98, 113512–113516 CrossRef.
- D. Wang, Polyvinylalcohol film doped with CuCl nanoclusters, Thin Solid Films, 1996, 288, 254–255 CrossRef CAS.
- G. Natarajan, A. Mitra, S. Daniels, D. C. Cameron and P. J. McNally, Temperature dependent optical properties of UV emitting gamma-CuCl thin films, Thin Solid Films, 2008, 516, 1439–1442 CrossRef CAS.
- R. Yang, X. Lu, X. Huang, Z. Chen, X. Zhang, M. Xu, Q. Song and L. Zhu, Bi-component Cu2O-CuCl composites with tunable oxygen vacancies and enhanced photocatalytic properties, Appl. Catal., B, 2015, 170–171, 225–232 CrossRef CAS.
- L. Ye, Y. Su, X. Jin, H. Xie and C. Zhang, Recent advances in BiOX (X = Cl, Br and I) photocatalysts: Synthesis, modification, facet effects and mechanisms, Environ. Sci.: Nano, 2014, 1, 90–112 RSC.
- S. Zhao, Y. Zhang, Y. Zhou, C. Zhang, L. Sheng and J. Fang, Reactable polyelectrolyte-assisted synthesis of BiOCl with enhanced photocatalytic activity, Chem. Eng., 2017, 5, 1416–1424 CAS.
- H. Chung, W. Chen, X. Wen, J. N. Hart, H. Wu, Y. Lai, R. Amal and Y. H. Ng, Oxygen-deficient bismuth tungstate and bismuth oxide composite photoanode with improved photostability, Sci. Bull., 2018, 63, 990–996 CrossRef CAS.
- K. Barrera-Mota, M. Bizarro, M. Castellino, A. Tagliaferro, A. Hernandez and S. E. Rodil, Spray deposited β-Bi2O3 nanostructured films with visible photocatalytic activity for solar water treatment, Photochem. Photobiol. Sci., 2015, 14, 1110–1119 CrossRef CAS PubMed.
- X. Peng, W. Dou, L. Kong, X. Hu and X. Wang, Removal of chloride ions from strongly acidic wastewater using Cu(0)/Cu(II): Efficiency enhancement by UV irradiation and the mechanism for chloride ions removal, Environ. Sci. Technol., 2019, 53, 383–389 CrossRef CAS PubMed.
- W. Dou, X. Hu, L. Kong and X. Peng, UV-improved removal of chloride ions from strongly acidic wastewater using Bi2O3: Efficiency enhancement and mechanisms, Environ. Sci. Technol., 2019, 53, 10371–10378 CrossRef CAS PubMed.
- N. Huang, W. Wang, Z. Xu, M. Lee, Q. Wu and H. Hu, A study of synergistic oxidation between ozone and chlorine on benzalkonium chloride degradation: Reactive species and degradation pathway, Chem. Eng. J., 2020, 382, 122856–122862 CrossRef CAS.
-
A. G. Sharpe, Inorganic chemistry, Longman Scientific & Technical, England, 3rd edn, 1992 Search PubMed.
- I. A. Ike, K. G. Linden, J. D. Orbell and M. Duke, Critical review of the science and sustainability of persulphate advanced oxidation processes, Chem. Eng. J., 2018, 338, 651–669 CrossRef CAS.
- H. V. Lutze, N. Kerlin and T. C. Schmidt, Sulfate radical-based water treatment in presence of chloride: Formation of chlorate, inter-conversion of sulfate radicals into hydroxyl radicals and influence of bicarbonate, Water Res., 2015, 72, 349–360 CrossRef CAS.
- S. Wacawek, H. V. Lutze, K. Grübel, V. V. T. Padil, M. Černík and D. D. Dionysiou, Chemistry of persulfates in water and wastewater treatment: A review, Chem. Eng. J., 2017, 330, 44–62 CrossRef.
- J. Wang and S. Wang, Activation of persulfate (PS) and peroxymonosulfate (PMS) and application for the degradation of emerging contaminants, Chem. Eng. J., 2018, 334, 1502–1517 CrossRef CAS.
- X. Hu, F. Zhu, L. Kong and X. Peng, Sulfate radical-based removal of chloride ion from strongly acidic wastewater: Kinetics and mechanism, J. Hazard. Mater., 2020, 410, 124540–124548 CrossRef PubMed.
-
X. Yang, X. Yan, J. Cui, J. Wang, X. Wang and X. Qin, Inorganic chemistry (in Chinese)Higher Education Press, Beijing, 2010 Search PubMed.
- W. Dou, X. Hu, L. Kong, X. Peng and X. Wang, Removal of Cl(-I) from strongly acidic wastewater using NaBiO3: A process of simultaneous oxidation and precipitation, Desalination, 2020, 491, 114566–114573 CrossRef CAS.
- J. P. Carr and N. A. Hampson, Lead dioxide electrode, Chem. Rev., 1972, 72, 679–703 CrossRef CAS.
- J. Zhao, L. Kong, X. Hu and X. Peng, Clean and effective removal of Cl(−I) from strongly acidic wastewater by PbO2, J. Environ. Sci., 2022, 120, 1–8 CrossRef PubMed.
|
This journal is © The Royal Society of Chemistry 2023 |