A promising approach to an environmentally friendly pest management solution: nanocarrier-delivered dsRNA towards controlling the destructive invasive pest Tuta absoluta†
Received
29th November 2022
, Accepted 22nd February 2023
First published on 24th February 2023
Abstract
To develop an environmentally friendly pest controlling RNAi method for the destructive invasive pest Tuta absoluta, we analysed the binding ratio between the nanocarrier and dsRNA, performed morphological characterization, investigated stability, leaf absorption rate and insect wall permeability of dsRNA–nanoparticle complexes, in addition to exploring the RNAi controlling effect and the safety risk analysis. Chitosan (CS) and star polycation (SPc) may be loaded with dsRNA, and the optimum fusion at dsRNA to CS and SPc mass ratios of 12.5
:
1 and 1
:
1, respectively. The morphology characterization was changed from chain-like dsRNA to spherical dsRNA–nanoparticle complexes, and the size became smaller. Nanomaterials effectively protect dsRNA from nuclease degradation, and significantly improved the leaf penetration efficiency. Furthermore, they penetrated the epidermis to enter the insect gut. Moreover, dskr-h1/CS and dskr-h1/SPc complexes, applied as a foliar spray, were fed to T. absoluta larvae and both complexes resulted in a significant reduction in pupal weight and emergence rate. Additionally, safety risk analysis showed that the dskr-h1 target gene had no effect on the non-target organism N. tenuis, and was safe for the environment. The target gene had no match sequence with humans or known predacity and parasitical enemies in the same ecological niche. In conclusion, the results first indicated that CS and SPc nanomaterials could successfully mediate specific target dsRNA delivery in T. absoluta, and dsRNA–nanoparticle complexes could be used to control the tomato leaf miner. The study confirmed and enriched that nanocarrier-delivered RNAi system could successfully be used for controlling pests other than model insects, providing a basis for the development of green, safe, efficient, and sustainable control of other pests.
Environmental significance
In recent years, with the continuous use of chemical pesticides, the negative effects on the environment and human health are increasing, which has aroused wide concern. Therefore, the development of green, safe and efficient pest control technology is very important for environmental protection and human health. In this study, we investigated the potential of using a nanocarrier-delivery system in controlling the tomato leaf miner and proved the feasibility of local spraying RNAi. As a green, safe, and efficient preparation, dskr-h1/CS and dskr-h1/SPc were applied to the control tomato leaf miner. Consumption induced a significant decrease in gene expression, which led to an abnormal pupal weight and emergence rate. We analyzed the safety of dskr-h1 and found that dskr-h1 had no effect on the non-target biological control organism, N. tenuis. We believe that our study makes a significant contribution to the literature because the application of nanomaterials has challenges and prospects in the sustainable development of agriculture.
|
Introduction
The tomato leaf miner, Tuta absoluta (Meyrick) belongs to the Lepidopteran family Gelechiidae and is native to Peru in western South America. The tomato leaf miner is a global quarantine pest. It was initially discovered in China in August 2017 in Huocheng County, Ili Kazakh Autonomous Prefecture, Xinjiang Uygur Autonomous Region.1 Extensive surveys from 2018 to 2019 showed that the distribution of tomato leaf miner encompassed a total of 33 cities in seven provinces (including a county-level city of Chongqing), among which Yunnan and Guizhou provinces had the most serious density and damage.2,3 Larval T. absoluta burrow into plants causing extensive damage to the mesophyll, flowers, fruits, terminal buds, and young shoots, resulting in shrinking and drying leaves, deformed and rotten fruits, and withered plants.2,4,5 It is a key destructive pest of processed and fresh tomatoes.6,7 China is the largest tomato producing country, with a rapid increase from 2000 to 2020, reaching 34.7% of the global production in 2020.8 To maintain this high level of tomato production, effective prevention and control measures should be urgently introduced in infected areas, and a detection and monitoring program should be initiated in uninfected areas for effective prevention.
Currently, the control options available for tomato leaf miner are limited to agricultural, biological, and chemical control. These include installing mechanical barriers in greenhouses for ventilation, regular removal of damaged tomato plants and old leaves, and covering the soil with plastic film.9,10 Additionally, screening resistant tomato varieties11 and cultivating transgenic tomato plants with high expression of Cry1Ac can also reduce tomato leaf miner damage.12 Biological control strategies using parasitic and natural predatory enemies targeting the eggs and larvae have been tested with varying degrees of success.13–19 The susceptibility of tomato leaf miner to entomopathogenic fungi and Bacillus thuringiensis was confirmed in 2006 and can be used to control the growth of the larval stage.20,21 Chemical control involves using sex pheromones for early detection, followed by pesticides treatment.22–24 Insecticide application is the main control currently used by farmers to manage this pest. However, the widespread use of insecticides can lead to insect resistance, non-target biological side effects, and increased crop protection costs. Therefore, we need to reduce our over-reliance on chemical pesticides and increase efficiency by integrating chemical solutions with other healthy and environmentally friendly prevention and control methods.
RNA interference (RNAi) was named one of the top 10 scientific technologies by Science in 2001 and 2002. There are many advantages to this technology. It is highly specific and accurate, as well as easily degradable and environmentally friendly. Moreover, it is simple to use, has a short working cycle which greatly reduces labor and time costs, and can be applied in the field by spraying or irrigation.25 Studies have shown that inhibiting the expression of important genes for insect growth and development can cause insect disorders or death. Therefore, RNAi is a potentially effective method for controlling pests, avoiding overuse of chemical pesticides and promoting the development of sustainable agriculture.26–29 RNAi was successfully applied to various Lepidopteran insects, such as Plutella xylostella and Spodoptera frugiperda.30–32 Camargo et al. screened for highly expressed target genes (592, 2594, 4623, 1360, and 4303) in different developmental stages based on the transcriptome, and confirmed that the tomato leaf miner has an RNAi system in vivo, which affects RNAi.33 In tomato leaf miner, the functions of vacuolar ATPase-A (V-ATpase) and arginine kinase (AK) were verified,34 and the ryanodine receptors (RyRs), acetylcholinesterase subunit 1 (AChE), and nicotinic acetylcholine receptor α6 (nAChRs) were evaluated as targets for controlling tomato leaf miner by dsRNA injection and root delivery.35 Bento et al. provided dsRNA targeting juvenile hormone inducible protein (JHP), chitin synthase A (CHI), carboxylesterase (COE), and arginine kinase (AK) via bacteria which resulted in a significant reduction in transcript accumulation and increase in larval mortality, confirming oral dsRNA via bacteria is a potential RNAi-based approach for tomato leaf miner control.36 The mortality rate of the tomato leaf miner was 50% when fed dsTa-αCOP, and the survival rate was under eight days after dsV-ATPase feeding.37 After feeding with juvenile hormone binding protein (JHBP) and V-TRPase B dsRNA, the mortality increased significantly, and leaf damage degree and feeding efficiency decreased.38 The research results show that RNAi can be used for functional verification of genes, and has great potential as a new pest control technology. The successful application of RNAi in tomato leaf miners through spraying demonstrates the efficiency of using dsRNA to control this pest in the future.
RNAi efficiency in insects is low (especially in Lepidoptera, Hemiptera and Diptera) as dsRNA has low hydrophilicity, negative charge, poor delivery and uptake efficiency, and sensitivity to nuclease degradation.39 To achieve effective pest control, we need to improve the penetration and interference efficiency and stability of dsRNA. Nanomaterials are the most promising materials in the 21st century and are widely used across various disciplines, including biology and medicine due to their chemical reactivity, small size, and large specific surface area.40–42 Nanoparticles can protect dsRNA from degradation by RNase, and also promote dsRNA entering the insect epidermis, cell and peritrophic membranes, and effectively interacting with the nucleus functions.30,43–45 Currently, nanomaterials used in insect research are mainly chitosan (CS), chitosan–sodium tripolyphosphate, liposome, perylenediimide-cored cationic dendrimers, guanidine-containing polymers, perylenediimide-cored cationic dendrimers, star polycation (SPc), etc.46 Among them, CS is a natural polymer material (derived from marine organisms) with high biosafety, good biocompatibility, and easy biodegradation. When dsRNA encounters CS in solution, they undergo electrostatic attraction to form a stable complex, which originates from the interaction between the glucosamine group in CS and the phosphate group in dsRNA.47–53 SPc are cationic dendrimers composed of four peripheral amino acid functionalized arms that can condense random nucleic acids into complexes that are easily absorbed by endocytosis.54–59 These complexes improve pesticides biological activity and reduce pesticides residues.60 The first nanomaterial used in pesticides was CS, which silenced the chitin synthase gene in Anopheles gambiae. The AgCHS1/CS nanoparticle complexes reduced the AgCHS1 transcript level by 62.8% and the chitin content by 33.8%, indicating nanoparticle-based RNAi technology has the potential for high-throughput screening in gene function for applications in pest control.61 Zheng et al. identified a nanomaterials-mediated dsRNA body wall permeation system affecting Aphis glycines, that achieved a high RNAi efficiency (95.4%) and a population inhibition effect (80.5%) after spraying on the soybean aphid.44 SPc combined with dsRNA can effectively silence the expression of target genes, with a knockout efficiency of 58.87–86.86%. A. glycines mortality rate reached 81.67% with dsATPD + dsATPE/SPc local application and 78.5% with dsATPD + dsCHS1/SPc spraying.59 After A. ypsilon ingested dsV-ATPase/SPc, the relative gene expression was reduced by 60% and body length was significantly reduced.58 Applying a matrine/SPc/dshem complex induced gene silencing by 64.76% with a 90.09% population control on the seventh day after spraying.62 The laboratory development of nanomaterial-delivered dsRNA technology provides a powerful tool for gene function analysis and has great potential for field RNAi-based pest control.
Tomato leaf miner is rapidly invading China and has a potential to inhabit a wide geographical range, devastating the tomato industry. Further research is urgently required to effectively control the pest. We aim to improve effective control of tomato leaf miner by combining dsRNA with nanoparticles. Firstly, we explored the protective effect of nanoparticles on dsRNA and determined the optimum fusion ratio of CS, SPc, and dsRNA. We observed the morphological characterization of the dsRNA/nanoparticle complex and tested the stability of the complex in RNase A. We then explored the prevention and control potential of nanoparticle mediated RNA and tested leaf absorption capacity, insect body wall penetration efficiency, gene silencing effect, prevention and control effectiveness, and biosafety. We concluded that the creation of a dsRNA/nanoparticle complex may provide an easily operated and efficient tool for RNAi based pest management (Fig. 1).
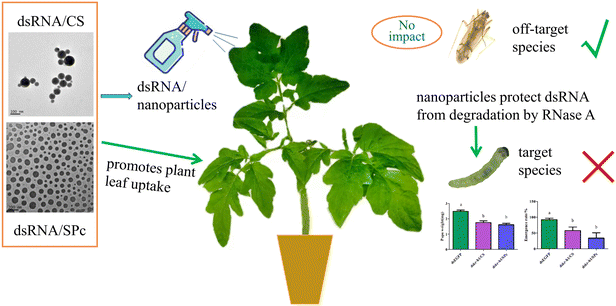 |
| Fig. 1 Nanocarrier-delivered dsRNA absorption on leaves. | |
Materials and methods
Insect rearing and host plants
The tomato leaf miner, Tuta absoluta, colony originated from Yuxi, Yunnan Province, in August 2018. The tomato variety was Maofen. The tomato leaf miner was reared in a laboratory insectary at 25 ± 2 °C with 50–60% relative humidity and a 14
:
10 h light
:
dark cycle. Host plants were individually grown in 9 cm-diameter pots in the same conditions as the tomato leaf miner.
RNA extraction and cDNA synthesis
Total RNA was isolated using the Micro total RNA extraction kit (Tianmo Biotech, Beijing, China). A NanoPhotometer™ P330 (Implen, Munich, Germany) with 1% agarose gel electrophoresis was used to determine RNA quality and concentration. First-strand cDNA was generated from 1 μg RNA using the SuperScript™ First-Strand Synthesis System (TransGen, Beijing, China).
Production of dsRNA transcription templates and synthesis of dsRNA
To generate dsRNA, two fragment templates of Met and kr-h1 were amplified by polymerase chain reaction (PCR) using cDNAs previously cloned as templates with forward and reverse primers containing the T7 primer sequence at the 5′ ends, respectively (F: taatacgactcactatagggTGGTTGCGGTAAAGGATT; R: taatacgactcactatagggTAACAGGGTCTGGCTGAG). Amplification reactions were conducted in 25 μL solutions with 19.0 μL of ddH2O, 2.5 μL of 10 × buffer, 0.5 μL of dNTPs (10 mM for each nucleotide), 1.0 mL of forward primer (10 mM μL−1), 1.0 mL of reverse primer (10 mM μL−1), 0.5 μL of cDNA template, and 0.5 μL of Taq DNA Polymerase (5 U μL−1; TransStart). The PCR cycling conditions were as follows: 94 °C for 5 min, followed by 35 cycles of 94 °C for 30 s, 60 °C for 30 s, and 72 °C for 30 s, and a final extension step of 72 °C for 10 min. The amplification of the PCR products was confirmed by separation on 1.5% agarose gels and visualized by staining with ethidium bromide under ultraviolet (UV) light. The sequencing was verified at Sangon Biotech. The dsRNA was synthesized using a MEGAscript RNAi kit (Ambion, Austin, TX, USA). One μg of PCR product was used as a transcription template. The dsRNA was resuspended in RNase free water. The dsRNA was analyzed by agarose gel electrophoresis and quantified spectrophotometrically. The dsRNA was stored at −80 °C until required.
Preparation of the dsRNA/CS nanoparticle complex
Preparation of the dsRNA/CS nanoparticle complexes followed Ramesh Kumar et al.63 method, with some modifications to obtain the optimal fusion ratio. 1) CS was dissolved in sodium acetate (NaAc) buffer (0.1 M sodium acetate–0.1 M acetic acid, pH of 4.5) to obtain a 0.02% wt/vol CS working solution, which was stored at room temperature; 2) 25 μg of dsRNA was dissolved in 100 μL of 50 mM sodium sulfate buffer and an equal volume of chitosan solution was added; 3) the mixture was heated in a metal bath at 55 °C for 1 min before transfer to a high-speed vortex oscillator for 30 s. The solution was left for 10 min to form the nanoparticle complex. The final concentration of dsRNA/CS was 125 ng μL−1; 4) Agarose gel electrophoresis detected the binding condition.
Preparation of the dsRNA/SPc nanoparticle complex
The SPc (Presented by China Agricultural University) was gently mixed with dsRNA at the recommended mass ratio of 1
:
1 (the final concentration for both SPc and dsRNA was 500 ng μL−1).59 1) SPc and dsRNA were mixed at a 1
:
1 mass ration and dissolved in ddH2O. To prepare the dsRNA/SPc complex, the mixture was incubated for 15 min at room temperature (25 °C). 2) In an aqueous solution, dsRNA spontaneously combined with SPc to form the dsRNA/SPc complex. Agarose gel electrophoresis detected the binding condition.
Determining the binding ratio between the chitosan nanocarrier and dsRNA
To identify the optimal fusion ratio of the dsRNA and nanomaterials, the CS working solution (prepared above) was diluted to 1/10, 1/20, 1/40, 1/80, and 1/100 following concentration gradient. Then 25 μg dsRNA was mixed with the diluted CS working solution to form a stable compound. A total of 5 μL of the mixed solution was added to 1 μL of 6 × DNA loading buffer. Agarose gel electrophoresis detected the dsRNA/nanoparticle complex binding. The optimal fusion ratio was determined using the electrophoresis results.
Particle size and morphological characterization of the complex – transmission electron microscopy (TEM) observation
The prepared dsRNA was diluted to a concentration of 1 μg L−1. The dsRNA/CS and dsRNA/SPc were prepared following a previously described method. A total of 1 μL dsRNA, CS solution, dsRNA/CS complex solution, SPc solution, and dsRNA/SPc complex solution were carefully added to a carbon film-coated copper wire, and placed at room temperature (25 °C) for 30 min to dry naturally. After drying, the samples were investigated using a JEM-1400 Flash transmission electron microscope. The optimum field of vision was required to move the samples with buttons or track balls. Magnification and brightness were adjusted before the morphology of each sample was photographed.
Scanning electron microscope (SEM) observation
A total of 1 μL of the prepared dsRNA solution, CS solution, dsRNA/CS complex solution, SPc solution, and dsRNA/SPc complex solution were placed on an aluminum sheet. The sheet was allowed to dry naturally at room temperature for 30 min. After drying, the sheet was pasted on the sample table and an ion sputtering apparatus sprayed gold for 30 s. The particles were placed in an S-4800 scanning electron microscope with 5.0 kV acceleration voltage. The overall morphology and associated characteristics of the particles were observed and photographed.
Stability test of dsRNA/nanoparticle complex
Hundred nanogram RNase A effectively degrades 1 μg dsRNA.64 We further explored the stability of the dsRNA/nanoparticle composite. The dsRNA/CS solution and dsRNA/SPc nanoparticle complex were diluted to 100 ng μL−1 with water, and evenly mixed before adding appropriate amounts of RNase A. The solutions were incubated for 20 min in the PCR apparatus at 37 °C. dsRNA quality was detected by agarose gel electrophoresis, and the brightness of the bands was measured by ImageJ. The dsRNA bands treated by RNase A were used as a control group. To calculate normalization, the grey value of the treatment group was divided by the grey value of the control group. Each treatment involved three replicates.
DsRNA absorption efficiency after different leaf treatments
To investigate the plant uptake of dsRNA, 20 μL cDNA was obtained by reverse transcription of dsRNA. A sample of 5 μL cDNA was successively diluted to 1/2, 1/4, 1/8, 1/16, and 1/32 following the concentration gradient. Quantitative primers QMet-F and QMet-R (forward: 5′-TGCTATTGATGATGTGCGAGT-3′ and reverse: 5′-AATGTTTTCTTTAGAGGGTGC-3′) amplified the samples. The cycle number was used as the ordinate and log (DNA copies) to create a standard curve and determine the relative quantitative dsRNA. The secondary structure of the dsRNA was destroyed in a metal bath at 65 °C for 5 min before undergoing reverse transcription.
The prepared dsRNA solution (at a final concentration of 25 ng μL−1) was sprayed on the leaf surface. The plant petiole was soaked in 200 μL of the same solution. After 4 hours, the leaves were collected and their RNA was extracted using the Trizol method. Real-time fluorescence quantitative PCR was used to detect the dsRNA content in the leaves after the different treatments. We recorded the maximum number of cycles, and calculated the log (DNA copies), according to the functional expression obtained from the standard curve. Four replicates were performed for each treatment and each replicate involved one tomato leaf. The control group was leaves without any dsRNA treatment.
Detecting uptake capacity of dsRNA/nanoparticle complexes in plant leaves
200 μL sample of the prepared dsRNA, dsRNA/CS, and dsRNA/SPc solutions, with a concentration of 25 ng μL−1 was evenly sprayed on the tomato leaves. After 4 hours of full absorption, quantitative PCR (qPCR) was used to determine the dsRNA contents. Each treatment involved four repetitions, each with one tomato leaf. The control leaves were sprayed with 200 μL ddH2O.
Detection of the penetration of dsRNA/nanoparticle complexes in insect body walls
Fluorescent dsRNA determined the penetration effect of the nanoparticle-delivered dsRNA in the insect body wall. Second instar tomato leaf miner larvae were placed in an ice box. Fluorescent dsRNA was placed on the back of the larvae using a microinjector. After absorption for 4 hours, the larvae were placed under a SZX16 fluorescence microscope to observe the fluorescence intensity. ImageJ was analyzed and the fluorescence intensity compared.
Control effect of the dsRNA/nanoparticle complex on the tomato leaf miner moth
The branches and leaves were separated from the tomato plants before inserting them into bottles for hydroponic treatment. The second instar larvae were placed on the leaves. The prepared dskr-h1, dskr-h1/CS, or dskr-h1/SPc solution was evenly sprayed on the tomato leaves. After 48 hours, five larvae were randomly selected for detection of expression levels. The remaining larvae were observed every 24 hours. We recorded the phenotypic traits including death, pupation, and emergence. An aqueous solution (25 ng μL−1) containing dsEGFP was used as a control. The experimental design included four biological replicates for each treatment.
Safety evaluation of dsRNA/nanoparticle complexes
Nucleic acid sequencing of RNAi target genes identified potential non-target sites in the transcriptome sequences of other species using Basic Local Alignment Search Tool (Blast) analysis, with the National Center for Biotechnology Information (NCBI) Blast – word size defined as 28 (https://www.ncbi.nlm.nih.gov/), match/mismatch scores defined as 1/−4 for the sequence alignment.65 Sequences with an E value ≤ 2 and cover scores ≥90% of the comparison results limited the mismatch to 2 base pairs.66 Safety was evaluated using the alignment results.
As a natural predator, Nesidiocoris tenuis is often the main biological control used for tomato leaf miner.67,68 Therefore, to ensure the benefits of biological controls are maintained, we explored the biological safety of dsRNA. Tomato leaves were sprayed with dskr-h1 and placed in an insect feeding device. A total of 20 adult N. tenuis insects were released into the device. The T. absoluta eggs and first instar larvae were randomly distributed on the leaves to feed the N. tenuis. The experiment involved six replicates. A control involved spraying with dsEGFP. N. tenuis and observing the insects daily for 10 days. The data were used to calculate the survival rate.
Statistical analysis
Analysis of variance (ANOVA) with Tukey's honestly significant difference (HSD) tests were performed using SPSS 20.0 (SPSS Inc., USA). Two-tailed Student's t-tests were performed using Prism 5.0 (GraphPad Software Inc., USA). Data were presented as the mean ± standard error (mean ± SEM). Differences were considered significant when P < 0.05.
Results
The nanomaterial binds to dsRNA to form a stable complex
A sample of 25 μg dsRNA was mixed with a CS working solution at different mass ratios and detected using agarose gel electrophoresis (ESI† Fig. S1). When the mass ratio of dsRNA to CS was 1.25
:
1 and 12.5
:
1, the dsRNA and CS were completely combined and clustered in the spot sample wells. When the mass ratio of dsRNA to CS was 25
:
1, part of the dsRNA was separated in the spot sample wells. This indicated that the dsRNA did not completely combine with the CS solution at this concentration, resulting in partial dsRNA shifting by electrostatic forces. When the mass ratio was 50
:
1, 100
:
1, or 125
:
1, the dsRNA dissipated from the band, indicating that CS concentration was too low to fully combine with dsRNA. Therefore, the brightness and distribution of the electrophoretic bands revealed the optimum fusion was at dsRNA to CS mass ratio of 12.5
:
1.
Nitrogen ions in the periphery of the SPc are positively charged and bound to the negatively charged dsRNA by electrostatic attraction. After mixing at a mass ratio of 1
:
1, gel electrophoresis revealed bright nucleic acid residues in the gel wells, indicating that dsRNA lost all electrical properties after binding to SPc. Therefore, in electrophoresis, dsRNA was blocked and no excess dsRNA escaped, indicating that at this mass ratio, dsRNA was fully bound to the SPc (ESI† Fig. S2).
Complex morphology characterization of the dsRNA/nanoparticle
Morphological characterization by TEM.
The morphological structure and surface morphology of the prepared dsRNA, CS, and SPc were clarified using transmission electron microscopy (TEM). In TEM, dsRNA formed a chain (Fig. 2A), while CS formed a block (Fig. 2B). After mixing dsRNA and CS, the dsRNA branches fully bound to CS (Fig. 2C), revealing a spherical distribution with an average particle size of 59 ± 23 nm. The SPc was a snowflake shape. When dsRNA was combined with SPc, they formed uniform spheres with an average grain diameter of 67 ± 24 nm (Fig. 2E).
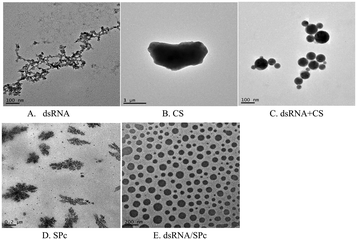 |
| Fig. 2 TEM images of dsRNA and nanoparticles. Morphological characterization of A. dsRNA; B. CS; C. dsRNA/CS; D. SPc; E. dsRNA/SPc. | |
Morphological characterization by SEM.
The SEM revealed branched dsRNA (Fig. 3A) and block-shaped CS (Fig. 3B). A significant change in morphology and structure was revealed when the two were combined (Fig. 3C). The SPc was a regular snowflake shape (Fig. 3D) and tightly bound together through electrostatic attraction after mixing with dsRNA (Fig. 3E).
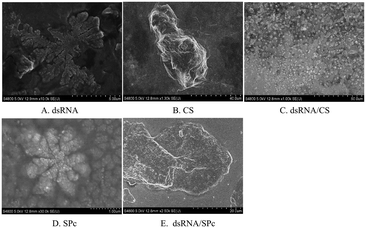 |
| Fig. 3 SEM images of the dsRNA and nanoparticles. Morphological characterization of A. dsRNA; B. CS; C. dsRNA/CS; D. SPc; E. dsRNA/SPc. | |
Nanomaterials protect dsRNA from degradation by RNase A
The dsRNA was degraded quickly by RNase A. Interestingly, when CS was bound to dsRNA, it protected the dsRNA from RNase A degradation (Fig. 4A), and the band density did not significantly vary (Fig. 4B). Similarly, SPc also bound to the dsRNA and protected the dsRNA from degradation by RNase A (Fig. 4C/D).
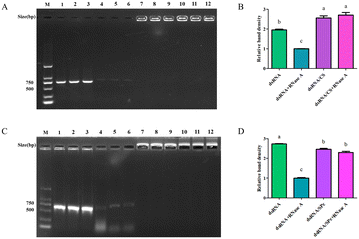 |
| Fig. 4 Stability of the dsRNA/CS complex in RNase A. A. Gel electrophoresis images of dsRNA after different treatments. M: DNA marker; 1–3: dsRNA; 4–6: dsRNA + RNase A; 7–9: dsRNA/CS; 10–12: dsRNA/CS + RNase A. B. Density analysis of the dsRNA bands. C. Gel electrophoresis images of dsRNA after different treatments. M: DNA marker; 1–3: dsRNA; 4–6: dsRNA + RNase A; 7–9: dsRNA/SPc; 10–12: dsRNA/SPc + RNase A. D. Density analysis of the dsRNA bands. The different letters (a–c) represent groups with significant differences according ANOVA test (Tukey's test, P < 0.05). | |
Efficient leaf uptake of dsRNA delivered by spraying or soaking
The dsRNA was diluted using a gradient and the cDNA was used as a template to construct a standard curve to calculate the dsRNA content in the leaves (Fig. 5A). Tomato leaves were treated by either soaking or spraying. The results showed that dsRNA effectively entered the leaves after soaking or spraying. Spraying significantly increased dsRNA leaf absorption efficiency (Fig. 5B).
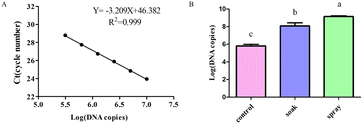 |
| Fig. 5 Detection of leaf absorption efficiency with different treatment methods. A. Standard curve used to calculate the dsRNA content. B. After tomato leaves were treated by either spraying or soaking for 4 h, the leaf RNA was extracted, and the cycle number of each sample was obtained by quantitative real-time PCR after reverse transcription. ANOVA assessed the data, and different letters indicated significant differences (P < 0.05). | |
The dsRNA/nanoparticle complex promotes plant leaf uptake
Leaves sprayed with dsRNA, dsRNA/CS, or dsRNA/SPc all contained dsRNA. The dsRNA content combined with SPc was significantly higher than the dsRNA in isolation or combined with CS. Therefore, SPc beneficially promoted the absorption of the double-stranded RNA in plant leaves (Fig. 6).
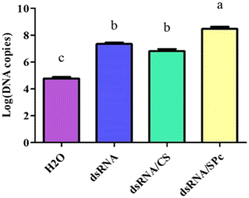 |
| Fig. 6 The absorption efficiency of dsRNA/nanoparticles complex in tomato leaves. H2O was used as a blank control group and dsRNA, dsRNA/CS, and dsRNA/SPc were each sprayed in a treatment group. A Tukey HSD test analyzed the data, different letters indicated significant differences (P < 0.05). The statistical results are expressed by means ± standard error. | |
The dsRNA/nanoparticle complex promoted body wall penetration
The tomato leaf miner was treated by placing a drop of either fluorescent dsRNA, fluorescent dsRNA/CS, or fluorescent dsRNA/SPc. Almost no fluorescent signal was expressed in the control group (Fig. 7). The fluorescent dsRNA, fluorescent dsRNA/CS, and fluorescent dsRNA/SPc had strong fluorescent signals, indicating that both the dsRNA and dsRNA/nanoparticle complex can effectively penetrate the tomato leaf miner. Fluorescence intensity analysis showed that the overall average fluorescence intensity of the tomato leaf miners treated with fluorescent dsRNA and dsRNA/SPc was significantly higher than fluorescent dsRNA/CS (Fig. 7E).
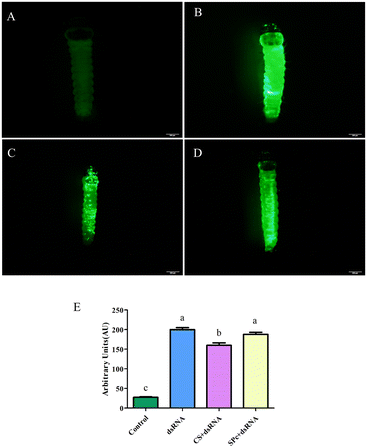 |
| Fig. 7 Infiltration effect of the transdermal dsRNA delivery system in Tuta absoluta. A. The control group, the second instar larvae were not treated with fluorescent dsRNA. B. Images of larvae treated with fluorescent dsRNA after 4 h. C. Images of larvae treated with fluorescent dsRNA/CS after 4 h. D. Images of larvae treated with fluorescent dsRNA/SPc after 4 h. E. Comparison of fluorescence intensity between the different treatments. A Tukey HSD test analyzed the data, different letters indicated significant differences (P < 0.05). The statistical results are expressed by means ± standard error. | |
T. absoluta control by the dsRNA/nanoparticle complex
dsRNA was fed to the tomato leaf miner through the leaf and the target gene was Takr-h1. Feeding dskr-h1/CS and dskr-h1/SPc nanoparticle complexes for 24 h significantly reduced the expression level of Takr-h1 at a rate 57.9% (dskr-h1/CS) and 60.7% (dskr-h1/SPc) lower than the control group (Fig. 8A). After consumption, the average pupal weight significantly decreased by 0.73 mg (29.29%) and 0.87 mg (35.03%), respectively (Fig. 8B) and the emergence rate by 34.74% and 58.36%, respectively (Fig. 8C).
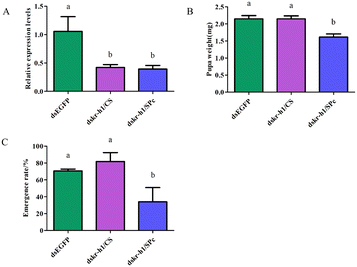 |
| Fig. 8 (A) Changes in the relative expression of the kr-h1 gene after consuming the dskr-h1/nanoparticle complex. (B) Changes in pupal weight after consuming the dskr-h1/nanoparticle complex. (C) Emergence rate after consuming the dskr-h1/nanoparticle complex. A Tukey HSD test analyzed the data, different letters indicated significant differences (P < 0.05). The statistical results are expressed as means ± standard error. | |
dskr-h1 has high biosafety
We analyzed the BLAST results of the nucleotide sequence of dskr-h1 in NCBI (ESI† Table S1). The sequence alignment results revealed that the gene is significantly similar to the sequences of six Lepidoptera insects, and the sequence coverage is only 5–13%. There was no match with the human genome, nor any predators or parasites in the same ecological niche. Therefore, non- and off-target effects were avoided. There was no significant difference in the survival of N. tenuis between the treatment with dsTakr-h1, dsGFP or the controls (ESI† Fig. S3). Therefore, the exogenous application of dsTakr-h1 may have no lethal effects on N. tenuis.
Discussion
Gel electrophoresis revealed that dsRNA effectively binds to chitosan (CS) and star polycation (SPc). CS nanoparticle complex is a spherical complex formed by the interaction between the negatively charged phosphate group (PO43−) of dsRNA and the positively charged amino group (–NH2) of the CS polymer. The particle sizes of the dsRNA/CS nanoparticle complexes varied as a function of the ratios.66,69 When the RNA to CS mass ratio was 12.5
:
1, the nanoparticle size was 59 ± 23 nm when measured by TEM. SPc had four arms in a core with tertiary amines. It stably binds dsRNA through electrostatic, hydrogen bonding, and van der Waals forces to improve the transfection efficiency.58,64 TEM revealed that the dsRNA became spherical after binding to SPc; and dsRNA/SPc nanoparticle complex particle size was 67 ± 24 nm. The SEM amplified dsRNA and SPc state and found them closely polymerized together. Owing to different TEM and SEM imaging principles, in which SEM observes the fine structure on the uneven surface of the sample, while TEM observes the fine structure inside the sample, different morphological characteristics were presented. The matrine/SPc complex produces similar morphological characteristics.70 Previous related research has revealed SPc reduces the particle size of osthole from 289 to 18 nm, as the osthole was encapsulated inside the cavity between the SPc arms, altering the osthole structure, resulting in spherical particles for most of the osthole/SPc complexes.71 The CS/SPc complex had similar morphological characteristics, with the CS particles in the aqueous solution decreasing from 145 to 17 nm.72 Research into binding dinotefuran to SPc, found that SPc could spontaneously bind to dinotefuran through hydrogen bonding using van der Waals force, and the particle size of dinotefuran was reduced from 269 to 29 nm.60 Previous research on SPc showed that the addition of SPc can effectively reduce the molecular particle size, making it more conducive to absorption by insects and leaves. The absorption by spraying and soaking of the tomato leaves was compared. The study found that both application methods transferred the dsRNA to the leaf interior. The absorption efficiency of dsRNA by the leaves was higher after spraying, which suggests spraying dsRNA preparations may be effective in the field/greenhouse. Related studies support the possibility of the foliar spraying dsRNA.73 Plant cells have totipotency, indicating that the loss of some plant organs can potentially recover a complete plant, including tomato cuttings in hydroponic culture.74 Therefore, there is also a potential for tomato leaves to fully absorb dsRNA by soaking, with totipotency allowing the treated leaves to develop into complete plants. This may reduce the damage caused by tomato leaf miner and improve control of this pest. Recent studies have reported that nanomaterials (such as SPc) can be used as carriers to deliver ROS-eliminating compounds by foliar application to enhance the stress tolerance of tomato.75 In an experiment to alleviate cucumber salt stress, foliar spraying produced better results than root application.76 Additionally, previous studies have shown that treating seeds with nanomaterials can improve seed quality, promote growth, and increase yield, which is potentially more environmentally friendly than foliar spraying or root applications.77–79 Therefore, future field application trials should investigate the pest control effects of foliar spraying, leaf soaking (with complete plants grown from the treated leaf), and/or seed dsRNA treatments.
Fluorescent labeling showed that CS effectively delivered dsRNA to the cells of S. frugiperda80 and Caenorhabditis elegans.81 By analyzing fluorescence intensity in the larval body wall after penetration, we concluded that SPc did not affect dsRNA delivery. Conversely, including CS in the dsRNA delivery attenuated the body wall penetration of the tomato leaf miner. We speculate that the acidic substances (such as sodium acetate, with a pH of 4.5) and salt substances (such as sodium sulfate) in the CS working solution causes the larvae to reject the solution. A study on C. elegans, found the pH of the intestine affected uptake.81 A thorough understanding of the uptake pathways of the nanoparticles is the basic requirement for applying nanobiotics in agriculture. The delivery pathways for nanoparticles into insect cells have previously been explored.29 The main pathway for nanoparticles to enter leaves is through the cuticle or stoma to reach the mesophyll and enter the plant vasculature.82–86 In terms of leaf absorption and insect body wall penetration efficiencies, SPc is more conducive to dsRNA delivery. A key factor for successful RNA-mediated pest control is the guaranteed stability of dsRNA during delivery.45,87 We detected that CS and SPc both played a role in successfully protecting dsRNA from RNase A degradation. Two truncated fragments of CYP6CY3 (P1/P2) dsRNA were delivered by the nanocarrier SPc transdermic delivery system, which successfully silenced the target genes in Aphis gossypii. After spraying A. gossypii with SPc + dsP1/P2 for 48 h, the expression levels were significantly reduced by 79.4% (CYP6CY3 P1 dsRNA) and 84.3% (CYP6CY3 P2 dsRNA), and the mortality rate at 84 h was 48.1% and 43.2%, respectively.88 Current data confirms both CS and SPc can protect dsRNA from RNase A and insect hemolymph degradation, and also promote the effective uptake of dsRNA by cells.64,66 After applying dskr-h1/CS and dskr-h1/SPc for 24 h, the RNAi efficiency was significant, and the effects on pupal weight and emergence rate were also significant. Previous research has demonstrated spray delivery systems based on nanomaterial SPc enhance the ability of dsRNA formulations to penetrate the insect epidermis, thereby triggering efficient RNAi in insects.29,73,88–90 From the perspective of effective control of tomato leaf miner, kr-h1 is not the best target gene yet. One of the possible reasons for its low mortality rate is that the growth, development and survival of insects are jointly regulated by multiple genes. Single gene interference may stimulate other complementary pathways. To achieve effective control of dsRNA against tomato leaf miner, more critical target genes should be screened or two or more target genes should be used together. DsRNA/nanoparticle complexes have broad research prospects when compared to the various potential ecological consequences of traditional pesticides. For future studies, screening of potential target genes should be conducted to increase mortality rate and efficient RNAi uses should be identified to effectively control tomato leaf miner in large-scale tomato production.
RNAi is highly sequence-specific, allowing the short dsRNA to directly degrade target transcripts. However, the conservation of this pathway in eukaryotes suggests that presence of sufficient homologous transcripts may have effect on nontarget organisms when using dsRNA.91 Therefore, a preventive approach should be incorporated when designing dsRNA, where regions of the target genes can be selected to ensure effects on non-target transcripts are minimized and any off-target matches are identified and avoided.92 We designed the dsRNA by selecting specific regions of the target gene to minimize non-target transcript homology in order to reduce the risk of non- and off-target effects. The results of Blast provide a preliminarily demonstration of the safety of the dsRNA. Ulrich et al. suggested that RNAi target genes investigation should not be limited to species or taxon-specific genes.65 The design of target sequences needs to focus on avoiding off-target effects in species deserving protection (such as honeybees) or common species in the local environment. Applying dskr-h1 can cause T. absoluta mortality, which is a safe biological control of N. tenuis. The low survival rate may be due to N. tenuis possessing low biological activity in response to changes in their living environment, host plants, and insects. In addition, the biotoxicity of nanoparticles has attracted much attention. The SPc oral LC50 for predatory ladybirds was 9925 times of the working SPc concentration, indicating the safety of SPc in practical production, and the application of SPc at extremely high concentration hardly affected the hatching of ladybirds eggs.93 However, long-term exposure of fruit fly larvae to SPc will have adverse effects on adult fruit flies.94 Therefore, application guidelines must be followed when used at a large-scale.
Nanomaterials have unique physical and chemical properties, such as small size and excellent catalytic activity, and can uniquely integrate with cell metabolism. The application of nanomaterials faces challenges and prospects in the sustainable agriculture space. However, before implementing dsRNA/nanoparticle complexes to control tomato leaf miner and other species, we must clarify the entry route of these complexes in the insect body, leaves, root tips, and seeds. Further research is also required on the influence of size, surface charge, and pH of the nanoparticle complexes on the interference effect. The triggered biochemical and molecular level reactions require in-depth understanding. Concurrently, it is essential to consider potential environmental and human health risks. Therefore, future research should involve in-depth research on nanoparticle complexes to provide a strong basis in supporting sustainable agriculture.
Conclusion
In this experiment, we proved the feasibility of local RNAi spraying. As a green, safe, and efficient preparation, dskr-h1/CS and dskr-h1/SPc were applied on tomato leaf miner. This treatment provides a promising and environmentally friendly pest management method. Nanomaterials have unique physical and chemical properties, such as small size, positive charge, excellent catalytic activity, and ability the to be uniquely integrated with the cell metabolism process, hence capable of effectively loading dsRNA, preventing dsRNA degradation, and being effectively absorbed in plant and insect cells. Consuming dskr-h1/CS and dskr-h1/SPc complexes by the tomato leaf miner induced a significant decrease in gene expression, leading to an abnormal pupal weight and emergence rate. We analyzed the safety of dskr-h1 and found that dskr-h1 had no effect on the non-target organism, N. tenuis. The application of nanomaterials has challenges and prospects in the sustainable agriculture space. We hope our research has further improved and enriched the nanocarrier-delivered system to provide a promising approach for sustainable pest control.
Author contributions
Xiaodi Wang: research summary, methodology, software, writing – original draft, writing – review & editing. Shunxia Ji: writing – review & editing. Siyan Bi: writing – review & editing. Yanhong Tang: writing – review & editing. Guifen Zhang: supervision. Shuo Yan: methodology. Fanghao Wan: writing – review & editing. Zhichuang Lü: conceptualization, funding acquisition, supervision, writing – review & editing. Wanxue Liu: funding acquisition.
Conflicts of interest
There are no conflicts of interest to declare.
Acknowledgements
This work was funded by the National Key Research and Development Program (2021YFD1400200, 2022YFC2601004 and 2021YFC2600400).
References
- G. F. Zhang, D. Y. Ma, W. X. Liu, Y. S. Wang, W. J. Fu, J. Wang, Y. H. Gao and F. H. Wan, The arrival of Tuta absoluta (Meyrick) (Lepidoptera: Gelechiidae), in China, Shengwu Anquan Xuebao, 2019, 28(03), 200–203 Search PubMed
, (in Chinese).
- G. F. Zhang, X. Q. Xian, Y. B. Zhang, R. Zhang, D. Y. Ma, W. X. Liu, Y. H. Gao, J. Wang, Z. L. Yang, Q. H. Li, Y. S. Wang, Y. T. Xue and F. H. Wan, Warning of the dispersal of a newly invaded alien species, tomato leaf miner Tuta absoluta (Meyrick), in China, Zhiwu Baohu, 2020, 46(02), 281–286 Search PubMed
, (in Chinese).
- G. F. Zhang, X. Q. Xian, Y. B. Zhang, W. X. Liu, H. Liu, X. D. Feng, D. Y. Ma, Y. S. Wang, Y. H. Gao, R. Zhang, Q. H. Li, F. H. Wan, W. J. Fu, J. Wang, M. Kuang, W. J. Yang, X. Rao, Y. Gao and A. M. Dai, Outbreak of the South American tomato leafminer, Tuta absoluta, in the Chinese mainland: geographic and potential host range expansion, Pest Manage. Sci., 2021, 77(12), 5475–5488 CrossRef CAS PubMed
.
- L. Batalla-Carrera, A. Morton and F. García-Del-Pino, Efficacy of entomopathogenic nematodes against the tomato leafminer Tuta absoluta in laboratory and greenhouse conditions, BioControl, 2010, 55, 523–530 CrossRef
.
- N. Desneux, E. Wajnberg, K. A. G. Wyckhuys, G. Burgio, S. Arpaia, C. A. Narváez-Vasquez, J. González-Cabrera, D. C. Ruescas, E. Tabone, J. Frandon, J. Pizzol, C. Poncet, T. Cabello and A. Urbaneja, Biological invasion of European tomato crops by Tuta absoluta: ecology, geographic expansion and prospects for biological control, J. Pest Sci., 2010, 83, 197–215 CrossRef
.
- J. B. Torres, C. A. Faria, W. S. Evangelista Jr. and D. Pratissoli, Within-plant distribution of the leaf miner Tuta absoluta (Meyrick) immatures in processing tomatoes, with notes on plant phenology, Int. J. Pest Manage., 2001, 47(3), 173–178 CrossRef
.
- R. Mansour, T. Brévault, A. Chailleux, A. Cherif, K. Grissa-Lebdi, K. Haddi, S. Mohamed, R. S. Nofemela, A. Oke, S. Sylla, H. E. Z. Tonnang, L. Zappalà, M. Kenis, N. Desneux and A. Biondi, Occurrence, biology, natural enemies and management of Tuta absoluta in Africa, Entomol. Gen., 2018, 38(2), 83–112 CrossRef
.
-
FAOSTAT, FAO Statistical Database (FAOSTAT), ICT Update, FAO, Rome, 2020, https://www.fao.org/faostat/en/#data/QCL, (accessed 11 March 2022).
-
E. P. Patricia and B. V. Alicia, Insects, mites and diseases associated with tomatoes in Chile, INIA Books Collection - Agricultural Research Institute, Santiago, Chile, GCS Prints, 2002 Search PubMed
.
- K. Abbes, A. Harbi and B. Chermiti, The tomato leafminer Tuta absoluta (Meyrick) in Tunisia: Current status and management strategies, Bull. OEPP, 2012, 42(2), 226–233 CrossRef
.
- A. Cherif, R. Mansour and K. Grissa-Lebdi, Biological aspects of tomato leafminer Tuta absoluta (Lepidoptera: Gelechiidae) in conditions of Northeastern Tunisia: possible implications for pest management, Environmental and Experimental Biology, 2013, 11, 179–184 Search PubMed
.
- H. Selale, F. Dağli, N. Mutlu, S. Doğanlar and A. Frary, Cry1Ac-mediated resistance to tomato leaf miner (Tuta absoluta) in tomato, Plant Cell, Tissue Organ Cult., 2017, 131, 65–73 CrossRef CAS
.
- C. H. Marchiori, C. G. Silva and A. P. Lobo, Parasitoids of Tuta absoluta (Meyrick, 1917) (Lepidoptera: Gelechiidae) collected on tomato plants in lavras,
State of Minas Gerais, Brazil, Braz. J. Biol., 2004, 64(3A), 551–552 CrossRef CAS PubMed
.
- M. A. Medeiros, N. J. Vilela and F. H. Franca, Eficiência técnica e econômica do controle biológico da traça-do-tomateiro em ambiente protegido, Hortic. Bras., 2006, 24(2), 180–184 CrossRef
.
- A. Biondi, R. N. C. Guedes, F. H. Wan and N. Desneux, Ecology, worldwide spread, and management of the invasive South American tomato pinworm, Tuta absoluta: past, present, and future, Annu. Rev. Entomol., 2018, 63, 239–258 CrossRef CAS PubMed
.
- A. Zouba, B. Chermiti, R. Chraiet and K. Mahjoubi, Effect of two indigenous Trichogramma species on the infestation level by tomato miner Tuta absoluta in tomato greenhouses in the south-west of Tunisia, Tunis. J. Plant Prot., 2013, 8(2), 87–106 Search PubMed
.
- S. A. El-Arnaouty, J. Pizzol, H. H. Galal, M. N. Kortam, A. I. Afifi, V. Beyssat, N. Desneux, A. Biondi and I. H. Heikal, Assessment of two Trichogramma species for the control of Tuta absoluta in North African tomato greenhouses, Afr. Entomol., 2014, 22(4), 801–809 CrossRef
.
- A. Cherif, R. Mansour, S. Barhoumi-Attia, L. Zappalà and K. Grissa-Lebdi, Effectiveness of different release rates of Trichogramma cacoeciae (Hymenoptera: Trichogrammatidae) against Tuta absoluta (Lepidoptera: Gelechiidae) in protected and open field tomato crops in Tunisia, Biocontrol Sci. Technol., 2018, 29(2), 149–161 CrossRef
.
- Y. B. Zhang, X. C. Tian, H. Wang, C. Castañé, J. Arnó, J. Collatz, J. Romeis, S. R. Wu, X. Q. Xian, W. X. Liu, F. H. Wan and G. F. Zhang, Host selection behavior of the host-feeding parasitoid Necremnus tutae on Tuta absoluta, Entomol. Gen., 2021, 42(3), 445–456 CrossRef
.
- S. M. Rodriguez, P. M. Gerding and I. A. France, Selección de aislamientos de hongos entomopatógenos para el control de huevos de la polilla del tomate, Tuta absoluta (Meyrick) (Lepidoptera: gelechiidae), Agric. Tec., 2006, 66(2), 151–158 Search PubMed
.
- L. Niedmann Lolas and L. Meza-Basso, Evaluación de cepas nativas de Bacillus thuringiensis como una alternativa de manejo integrado de la polilla del tomate (Tuta absoluta (Meyrick; Lepidoptera: Gelechiidae) en Chile), Agric. Tec., 2006, 66(3), 235–246 Search PubMed
.
- M. D. Collavino and R. A. Gimenez, Efecto del imidacloprid en el control de la polilla del tomate (Tuta absoluta Meyrick), Idesia, 2008, 26(1), 65–72 Search PubMed
.
- S. Vacas, C. Alfaro, J. Primo and V. Navarro-Llopis, Studies on the development of a mating disruption system to control the tomato leafminer, Tuta absoluta Povolny (Lepidoptera: Gelechiidae), Pest Manage. Sci., 2011, 67(11), 1473–1480 CrossRef CAS PubMed
.
- O. Campolo, A. Cherif, M. Ricupero, G. Siscaro, K. Grissa-Lebdi, A. Russo, L. M. Cucci, P. Di Pietro, C. Satriano, N. Desneux, A. Biondi, L. Zappalà and V. Palmeri, Citrus peel essential oil nanoformulations to control the tomato borer, Tuta absoluta: chemical properties and biological activity, Sci. Rep., 2017, 7(1), 13036 CrossRef PubMed
.
- S. E. Mohr, J. A. Smith, C. E. Shamu, R. A. Neumüller and N. Perrimon, RNAi screening comes of age: Improved techniques and complementary approaches, Nat. Rev. Mol. Cell Biol., 2014, 15(9), 591–600 CrossRef CAS PubMed
.
- J. Zhang, S. A. Khan, D. G. Heckel and R. Bock, Next-generation insect-resistant plants: RNAi-mediated crop protection, Trends Biotechnol., 2017, 35(9), 871–882 CrossRef CAS PubMed
.
- J. Z. Niu, G. M. Shen, O. Christiaens, G. Smagghe, L. He and J. J. Wang, Beyond insects: current status and achievements of RNA interference in mite pests and future perspectives, Pest Manage. Sci., 2018, 74(12), 2680–2687 CrossRef CAS PubMed
.
- M. Zotti, E. A. Dos Santos, D. Cagliari, O. Christiaens, C. N. T. Taning and G. Smagghe, RNA interference technology in crop protection against arthropod pests, pathogens and nematodes, Pest Manage. Sci., 2018, 74(6), 1239–1250 CrossRef CAS PubMed
.
- S. Yan, B. Y. Ren and J. Shen, Nanoparticle-mediated double-stranded RNA delivery system: A promising approach for sustainable pest management, Insect Sci., 2021, 28(1), 21–34 CrossRef CAS PubMed
.
- N. Kunte, E. Mcgraw, S. Bell, D. Held and L.-A. Avila, Prospects, challenges and current status of RNAi through insect feeding, Pest Manage. Sci., 2020, 76(1), 26–41 CrossRef CAS PubMed
.
- R. Ellango, R. Asokan, G. Sharath Chandra, N. K. Krishna Kumar, R. Mahmood and V. V. Ramamurthy, Tyrosine hydroxylase, a potential target for the RNAi-mediated management of diamondback moth (Lepidoptera: Plutellidae), Fla. Entomol., 2018, 101(1), 1–5 CrossRef CAS
.
- B. Z. Zhang, X. Su, C. A. Zhen, L. Y. Lu, Y. S. Li, X. Ge, D. M. Chen, Z. Pei, M. W. Shi and X. L. Chen, Silencing of Cytochrome P450 in Spodoptera frugiperda (Lepidoptera: Noctuidae) by RNA interference enhances susceptibility to chlorantraniliprole, J. Insect Sci., 2020, 20(3), 1–7 CrossRef PubMed
.
- R. de A. Camargo, R. H. Herai, L. N. Santos, F. M. Bento, J. E. Lima, H. Marques-Souza and A. Figueira, De novo transcriptome assembly and analysis to identify potential gene targets for RNAi-mediated control of the tomato leafminer (Tuta absoluta), BMC Genomics, 2015, 16(1), 635 CrossRef PubMed
.
- R. A. Camargo, G. O. Barbosa, I. P. Possignolo, L. E. Peres, E. Lam, J. E. Lima, A. Figueira and H. Marques-Souza, RNA interference as a gene silencing tool to control Tuta absoluta in tomato (Solanum lycopersicum), PeerJ, 2016, 4, e2673 CrossRef PubMed
.
- S. Majidiani, R. F. Pourabad, F. Laudani, O. Campolo, L. Zappalà, S. Rahmani, S. A. Mohammadi and V. Palmeri, RNAi in Tuta absoluta management: Effects of injection and root delivery of dsRNAs, J. Pest Sci., 2019, 92, 1409–1419 CrossRef
.
- F. M. Bento, R. N. Marques, F. B. Campana, C. G. Demétrio, R. A. Leandro, J. R. P. Parra and A. Figueira, Gene silencing by RNAi via oral delivery of dsRNA by bacteria in the South American tomato pinworm, Tuta absoluta, Pest Manage. Sci., 2020, 76(1), 287–295 CrossRef CAS PubMed
.
- S. Rahmani and A. R. Bandani, A gene silencing of V-ATPase subunit A interferes with survival and development of the tomato leafminer, Tuta absoluta, Arch. Insect Biochem. Physiol., 2021, 106(1), e21753 CrossRef CAS PubMed
.
- G. Ramkumar, R. Asokan, N. R. Prasannakumar, B. Kariyanna, S. Karthi, M. S. Alwahibi, M. S. Elshikh, A. Abdel-Megeed, A. Ghaith, S. Senthil-Nathan, K. Kalaivani, W. B. Hunter and P. Krutmuang, RNA interference suppression of v-ATPase B and juvenile hormone binding protein genes through topically applied dsRNA on tomato leaves: Developing biopesticides to control the South American pinworm, Tuta absoluta (Lepidoptera: Gelechiidae), Front. Physiol., 2021, 12, 742871 CrossRef PubMed
.
- E. Van Ekert, C. A. Powell, R. G. Shatters Jr and D. Borovsky, Control of larval and egg development in Aedes aegypti with RNA interference against juvenile hormone acid methyl transferase, J. Insect Physiol., 2014, 70, 143–150 CrossRef CAS PubMed
.
- G. M. Whitesides, J. P. Mathias and C. T. Seto, Molecular self-assembly and nanochemistry: a chemical strategy for the synthesis of nanostructures, Science, 1991, 254(5036), 1312–1319 CrossRef CAS PubMed
.
- H. Gleiter, Nanostructured materials: basic concepts and microstructure, Acta Mater., 2000, 48(1), 1–29 CrossRef CAS
.
- D. Nykypanchuk, M. M. Maye, D. van der Lelie and O. Gang, DNA-guided crystallization of colloidal nanoparticles, Nature, 2008, 451, 549–552 CrossRef CAS PubMed
.
- D. X. Shen, F. Zhou, Z. J. Xu, B. C. He, M. Li, J. Shen, M. Z. Yin and C. J. An, Systemically interfering with immune response by a fluorescent cationic dendrimer delivered gene suppression, J. Mater. Chem. B, 2014, 2(29), 4653–4659 RSC
.
- Y. Zheng, Y. S. Hu, S. Yan, H. Zhou, D. L. Song, M. Z. Yin and J. Shen, A polymer/detergent formulation improves dsRNA penetration through the body wall and RNAi-induced mortality in the soybean aphid Aphis glycines, Pest Manage. Sci., 2019, 75(7), 1993–1999 CrossRef CAS PubMed
.
- S. Yan, B. Y. Ren, B. Zeng and J. Shen, Improving RNAi efficiency for pest control in crop species, BioTechniques, 2020, 68(5), 283–290 CrossRef CAS PubMed
.
- X. D. Wang, S. X. Ji, X. N. Shen, W. X. Liu, F. H. Wan, G. F. Zhang and Z. C. Lü, Research and application of nanoparticle-mediated RNAi technology in pest control, Zhongguo Shengwu Fangzhi Xuebao, 2021, 37(06), 1298–1312 Search PubMed
, (in Chinese).
- S. C. De Smedt, J. Demeester and W. E. Hennink, Cationic polymer based gene delivery systems, Pharm. Res., 2000, 17(2), 113–126 CrossRef CAS PubMed
.
- T. G. Park, J. H. Jeong and S. W. Kim, Current status of polymeric gene delivery systems, Adv. Drug Delivery Rev., 2006, 58(4), 467–486 CrossRef CAS PubMed
.
- X. Zhang, K. Mysore, E. Flannery, K. Michel, D. W. Severson, K. Y. Zhu and M. Duman-Scheel, Chitosan/interfering RNA nanoparticle mediated gene silencing in disease vector mosquito larvae, J. Visualized Exp., 2015, 97, 52523 Search PubMed
.
- R. K. Dhandapani, D. Gurusamy, J. L. Howell and S. R. Palli, Development of CS-TPP-dsRNA nanoparticles to enhance RNAi efficiency in the yellow fever mosquito, Aedes aegypti, Sci. Rep., 2019, 9, 8775 CrossRef PubMed
.
- W. Xiao, J. Peng and Y. D. Zhang, Preparation and transfection efficiency of mPEG-CS/livin shRNA complex, Zhongguo Zuzhi Gongcheng Yanjiu Yu Linchuang Kangfu, 2011, 15(16), 2920–2924 CAS
, (in Chinese).
- Y. Zhao, S. Q. Jian and X. R. Zhang, The advance of chitosan as targeted preparation carrier material, Jilin Yiyao Xueyuan Xuebao, 2012, 33(1), 35–38 Search PubMed
, (in Chinese).
- Q. D. Shao, Z. Wang, Y. F. Li, T. M. Xu, J. Y. Sun, F. Jiang and F. X. Lv, Stromal vascular fraction combined with acellular bone matrix-chitosan scaffold for radical defect repair, Zhongguo Zuzhi Gongcheng Yanjiu, 2017, 21(6), 843–847 Search PubMed
, (in Chinese).
- G. Kong, R. D. Braun and M. W. Dewhirst, Hyperthermia enables tumor-specific nanoparticle delivery: effect of particle size, Cancer Res., 2000, 60(16), 4440–4445 CAS
.
- K. Y. Win and S. S. Feng, Effects of particle size and surface coating on cellular uptake of polymeric nanoparticles for oral delivery of anticancer drugs, Biomaterials, 2005, 26(15), 2713–2722 CrossRef CAS PubMed
.
- M. Z. Yin, J. Shen, R. Gropeanu, G. O. Pflugfelder, T. Weil and K. Müllen, Fluorescent core/shell nanoparticles for specific cell-nucleus staining, Small, 2008, 4(7), 894–898 CrossRef CAS PubMed
.
- Z. J. Xu, B. C. He, W. Wei, K. L. Liu, M. Z. Yin, W. T. Yang and J. Shen, Highly water-soluble perylenediimide-cored poly (amido amine) vector for efficient gene transfection, J. Mater. Chem. B, 2014, 2(20), 3079–3086 RSC
.
- J. H. Li, J. Qian, Y. Y. Xu, S. Yan, J. Shen and M. Z. Yin, A facile-synthesized star
polycation constructed as a highly efficient gene vector in pest management, ACS Sustainable Chem. Eng., 2019, 7, 6316–6322 CrossRef CAS
.
- S. Yan, J. Qian, C. Cai, Z. Z. Ma, J. H. Li, M. Z. Yin, B. Y. Ren and J. Shen, Spray method application of transdermal dsRNA delivery system for efficient gene silencing and pest control on soybean aphid Aphis glycines, J. Pest Sci., 2020, 93, 449–459 CrossRef
.
- Q. H. Jiang, Y. H. Xie, M. Peng, Z. J. Wang, T. J. Li, M. Z. Yin, J. Shen and S. Yan, Nanocarrier-pesticide delivery system with promising benefits in a case of dinotefuran: Strikingly enhanced bioactivity and reduced pesticide residue, Environ. Sci.: Nano, 2022, 9(3), 988–999 RSC
.
- X. Zhang, J. Zhang and K. Y. Zhu, Chitosan/double-stranded RNA nanoparticle-mediated RNA interference to silence chitin synthase genes through larval feeding in the African malaria mosquito (Anopheles gambiae), Insect Mol. Biol., 2010, 19(5), 683–693 CrossRef CAS PubMed
.
- M. S. Li, Z. Z. Ma, M. Peng, L. Li, M. Z. Yin, S. Yan and J. Shen, A gene and drug co-delivery application helps to solve the short life disadvantage of RNA drug, Nano Today, 2022, 43, 101452 CrossRef CAS
.
- D. Ramesh Kumar, P. Saravana Kumar, M. R. Gandhi, N. A. Al-Dhabi, M. G. Paulraj and S. Ignacimuthu, Delivery of chitosan/dsRNA nanoparticles for silencing of wing development vestigial (vg) gene in Aedes aegypti mosquitoes, Int. J. Biol. Macromol., 2016, 86, 89–95 CrossRef CAS PubMed
.
- Z. Z. Ma, Y. Zheng, Z. J. Chao, H. T. Chen, Y. H. Zhang, M. Z. Yin, J. Shen and S. Yan, Visualization of the process of a nanocarrier-mediated gene delivery: stabilization, endocytosis and endosomal escape of genes for intracellular spreading, J. Nanobiotechnol., 2022, 20(1), 124 CrossRef CAS PubMed
.
- J. Ulrich, V. A. Dao, U. Majumdar, C. Schmitt-Engel, J. Schwirz, D. Schultheis, N. Ströhlein, N. Troelenberg, D. Grossmann, T. Richter, J. Dönitz, L. Gerischer, G. Leboulle, A. Vilcinskas, M. Stanke and G. Bucher, Large scale RNAi screen in Tribolium reveals novel target genes for pest control and the proteasome as prime target, BMC Genomics, 2015, 16(1), 674 CrossRef PubMed
.
- H. Kolge, K. Kadam, S. Galande, V. Lanjekar and V. Ghormade, New frontiers in pest control: Chitosan nanoparticles-shielded dsRNA as an effective topical RNAi spray for gram podborer biocontrol, ACS Appl. Bio Mater., 2021, 4(6), 5145–5157 CrossRef CAS PubMed
.
- M. Pérez-Hedo, R. Suay, M. Alonso, M. Ruocco, M. Giorgini, C. Poncet and A. Urbaneja, Resilience and robustness of IPM in protected horticulture in the face of potential invasive pests, Crop Prot., 2017, 97, 119–127 CrossRef
.
- A. Urbaneja, J. González-Cabrera, J. Arnó and R. Gabarra, Prospects for the biological control of Tuta absoluta in tomatoes of the Mediterranean basin, Pest Manage. Sci., 2012, 68, 1215–1222 CrossRef CAS PubMed
.
- S. Das, N. Debnath, Y. Cui, J. Unrine and S. R. Palli, Chitosan, carbon quantum dot, and silica nanoparticle mediated dsRNA delivery for gene silencing in Aedes aegypti: A comparative analysis, ACS Appl. Mater. Interfaces, 2015, 7(35), 19530–19535 CrossRef CAS PubMed
.
- S. Yan, Q. Hu, J. H. Li, Z. J. Chao, C. Cai, M. Z. Yin, X. G. Du and J. Shen, A star polycation acts as a drug nanocarrier to improve the toxicity and persistence of botanical pesticides, ACS Sustainable Chem. Eng., 2019, 7(20), 17406–17413 CrossRef CAS
.
- S. Yan, Q. Hu, Q. H. Jiang, H. T. Chen, J. Wei, M. Z. Yin, X. G. Du and J. Shen, Simple osthole/nanocarrier pesticide efficiently controls both pests and diseases fulfilling the need of green production of strawberry, ACS Appl. Mater. Interfaces, 2021, 13(30), 36350–36360 CrossRef CAS PubMed
.
- X. D. Wang, K. K. Zheng, W. Y. Cheng, J. Li, X. X. Liang, J. Shen, D. L. Dou, M. Z. Yin and S. Yan, Field application of star polymer-delivered chitosan to amplify plant defense against potato late blight, Chem. Eng. J., 2021, 417, 129327 CrossRef CAS
.
- K. San Miguel and J. G. Scott, The next generation of insecticides: dsRNA is stable as a foliar-applied insecticide, Pest Manage. Sci., 2016, 72(4), 801–809 CrossRef CAS PubMed
.
- W. Q. Wang, J. Zhang, Q. P. Zhang, X. H. Pan and R. Hui, The primary study of soilless culture techniques of some plants, Xibei Shifan Daxue Xuebao, Ziran Kexueban, 2006, 42(5), 81–84 CAS
, (in Chinese).
- Y. Zhang, L. Fu, S. J. Jeon, J. Yan, J. P. Giraldo, K. Matyjaszewski, R. D. Tilton and G. V. Lowry, Star polymers with designed reactive oxygen species scavenging and agent delivery functionality promote plant stress tolerance, ACS Nano, 2022, 16(3), 4467–4478 CrossRef CAS PubMed
.
- L. Chen, Y. Peng, L. Zhu, Y. Huang, Z. Bie and H. Wu, CeO2 nanoparticles improved cucumber salt tolerance is associated with its induced early stimulation on antioxidant system, Chemosphere, 2022, 299, 134474 CrossRef CAS PubMed
.
- M. S. Amritha, K. Sridharan, J. T. Puthur and O. P. Dhankher, Priming with nanoscale materials for boosting abiotic stress tolerance in crop plants, J. Agric. Food Chem., 2021, 69, 10017–10035 CrossRef PubMed
.
- Z. Elhaj Baddar and J. M. Unrine, Effects of soil pH and coatings on the eficacy of polymer coated ZnO nanoparticulate fertilizers in wheat (Triticum aestivum), Environ. Sci. Technol., 2021, 55(20), 13532–13540 CrossRef CAS PubMed
.
- L. J. Zhao, T. H. Bai, H. Wei, J. L. Gardea-Torresdey, A. Keller and J. C. White, Nanobiotechnology-based strategies for enhanced crop stress resilience, Nat. Food, 2022, 3, 829–836 CrossRef
.
- G. Theerawanitchpan, N. Saengkrit, W. Sajomsang, P. Gonil, U. Ruktanonchai, S. Saesoo, T. W. Flegel and V. Saksmerprome, Chitosan and its quaternized derivative as effective long dsRNA carriers targeting shrimp virus in Spodoptera frugiperda 9 cells, J. Biotechnol., 2012, 160(3–4), 97–104 CrossRef CAS PubMed
.
- S. S. Lichtenberg, O. V. Tsyusko, S. R. Palli and J. M. Unrine, Uptake and bioactivity of chitosan/double-stranded RNA polyplex nanoparticles in Caenorhabditis elegans, Environ. Sci. Technol., 2019, 53(7), 3832–3840 CrossRef CAS PubMed
.
- A. Avellan, J. Yun, Y. L. Zhang, E. Spielman-Sun, J. M. Unrine, J. Thieme, J. Li, E. Lombi, G. Bland and G. V. Lowry, Nanoparticle size and coating chemistry control foliar uptake pathways, translocation, and leaf-to-rhizosphere transport in wheat, ACS Nano, 2019, 13(5), 5291–5305 CrossRef CAS PubMed
.
- Y. Shen, J. Borgatta, C. Ma, G. Singh, C. Tamez, N. P. Schultes, Z. Zhang, O. P. Dhankher, W. H. Elmer, L. He, R. J. Hamers and J. C. White, Role of foliar biointerface properties and nanomaterial chemistry in controlling Cu transfer into wild-type and mutant Arabidopsis thaliana leaf tissue, J. Agric. Food Chem., 2022, 70(14), 4267–4278 CrossRef CAS PubMed
.
- J. Hong, C. Wang, D. C. Wagner, J. L. Gardea-Torresdey, F. He and C. M. Rico, Foliar application of nanoparticles: mechanisms of absorption, transfer, and multiple impacts, Environ. Sci.: Nano, 2021, 8, 1196–1210 RSC
.
- Y. M. Su, V. Ashworth, C. Kim, A. S. Adeleye, P. Rolshausen, C. Roper, J. White and D. Jassby, Delivery, uptake, fate, and transport of engineered nanoparticles in plants: a critical review and data analysis, Environ. Sci.: Nano, 2019, 6, 2311–2331 RSC
.
- H. Wu and Z. Li, Nano-enabled agriculture: How do nanoparticles cross barriers in plants?, Plant Commun., 2022, 3, 100346 CrossRef CAS PubMed
.
- K. B. Rebijith, R. Asokan, H. R. Hande, N. K. Kumar, V. Krishna, J. Vinutha and N. Bakthavatsalam, RNA interference of odorant-binding protein 2 (OBP2) of the cotton aphid, Aphis gossypii (Glover), resulted in altered electrophysiological responses, Appl. Biochem. Biotechnol., 2016, 178(2), 251–266 CrossRef CAS PubMed
.
- L. Y. Wei, L. J. Zhang, N. Liu, X. W. Gao and X. N. Liu, Effect of RNAi targeting CYP6CY3 on the growth, development and insecticide susceptibility of Aphis gossypii by using nanocarrier-based transdermal dsRNA delivery system, Pestic. Biochem. Physiol., 2021, 177, 104878 CrossRef CAS PubMed
.
- I. El-Shesheny, S. Hajeri, I. El-Hawary, S. Gowda and N. Killiny, Silencing abnormal wing disc gene of the Asian citrus psyllid, Diaphorina citri disrupts adult wing development and increases nymph mortality, PLoS One, 2013, 8(5), e65392 CrossRef CAS PubMed
.
- N. Dias, D. Cagliari, F. S. Kremer, L. N. Rickes, D. E. Nava, G. Smagghe and M. Zotti, The South American fruit fly: An important pest insect with RNAi-sensitive larval stages, Front. Physiol., 2019, 10, 794 CrossRef PubMed
.
- S. J. Fletcher, P. T. Reeves, B. T. Hoang and N. Mitter, A perspective on RNAi-based biopesticides, Front. Plant Sci., 2020, 11, 51 CrossRef PubMed
.
- Y. Naito, T. Yamada, T. Matsumiya, K. Ui-Tei, K. Saigo and S. Morishita, dsCheck: Highly sensitive off-target search software for double-stranded RNA-mediated RNA interference, Nucleic Acids Res., 2005, 33, W589–W591 CrossRef CAS PubMed
.
- M. Dong, D. Chen, L. Che, N. Gu, M. Yin, X. Du, J. Shen and S. Yan, Biotoxicity evaluation of a cationic Star Polymer on a predatory ladybird and cooperative pest control by polymer-delivered pesticides and ladybird, ACS Appl. Mater. Interfaces, 2022, 14(4), 6083–6092 CrossRef CAS PubMed
.
- S. Yan, N. Li, Y. Guo, Y. Chen, C. Ji, M. Yin, J. Shen and J. Zhang, Chronic exposure to the star polycation (SPc) nanocarrier in the larval stage adversely impairs life history traits in Drosophila melanogaster, J. Nanobiotechnol., 2022, 20(1), 515 CrossRef CAS PubMed
.
|
This journal is © The Royal Society of Chemistry 2023 |