Cu2O@Co/N-doped carbon as antibacterial catalysts for oxygen reduction in microbial fuel cells†
Received
27th October 2022
, Accepted 3rd December 2022
First published on 7th December 2022
Abstract
Biofouling and sluggish kinetics on the cathode surface reduce the power generation of microbial fuel cells (MFCs). In this work, ZIF-derived Cu2O@Co/N-doped carbon (Cu2O@Co/NC) was used as an antibacterial oxygen reduction reaction (ORR) catalyst for MFCs. As an antibacterial agent on the cathode surface, Cu2O can inhibit the excessive growth of biofilms, facilitate the diffusion of OH− ions, and reduce the electron transfer resistance in ORR. The interaction between Cu2O and Co/NC was studied by charge density analysis. Charge redistribution can promote the adsorption of O2 molecules, resulting in enhanced ORR activity. The Cu2O@Co/NC cathode demonstrated superior ORR activities due to a half-wave potential of 0.80 V and an onset potential of 0.89 V versus RHE. The corresponding MFCs gained a maximum power density of 1100 mW m−2 after 450 h of operation, which was higher than that of Co/NC (739 mW m−2) and similar to that of commercial Pt/C (1067 mW m−2). Our work provides a strategy to achieve high power density of MFCs by combining the advantages of Cu2O and Co/NC catalysts.
Environmental significance
Biological sludge easily blocks the pores of the catalytic layer on the surface of cathode electrodes after long-term operation of microbial fuel cells. Bacterial contamination increases the charge transport impedance and hinders ion diffusion, reducing the power generation and wastewater degradation of the devices. We report ZIF-derived Cu2O@Co/NC as an antibacterial oxygen reduction catalyst for antibacterial effect and efficient power generation in microbial fuel cells. Cu2O as an antibacterial component can inhibit the excessive growth of biofilms on the surface of the cathode catalyst, thereby maintaining the diffusion channel of OH− ions and reducing the electron transfer resistance. The Cu2O@Co/NC cathode can ensure low overpotentials due to efficient ion diffusion and fast electron transfer, including a higher half-wave potential, onset potential and lower Tafel slope. The antibacterial mechanisms might include the induction of oxidative stress, prevention of cell replication and disruption of cell membrane integrity.
|
Introduction
Microbial fuel cells (MFCs) convert chemical energy into electricity via the degradation of organics using electroactive microorganisms to realize resource regeneration.1,2 MFCs, as bioelectrochemical hybrid systems, have been used in electricity generation, wastewater treatment, biohydrogen generation and biosensing.3 Oxygen reduction reaction (ORR) processes slow down due to the effect of cathode biofouling, further limiting the power generation of MFCs. In general, the colonization of biofilms blocks the channels of the cathode layer, thereby increasing the charge transfer resistance and hampering the diffusion of ions. The aerobic-respiring bacteria consume dissolved oxygen, resulting in the lowering of dissolved oxygen concentrations and the reduction of reaction kinetics as the biofilm thickness increased.4–7 Therefore, the hazard of biofouling prevents the MFCs from sustaining high power output for long periods of operation. Some methods such as physical cleaning, chemical cleaning, electric field and surface modification methods are used to mitigate biofouling.8 However, physical scraping requires regular treatment and chemical washing easily causes the catalyst layer to fall off. The mitigating biofouling methods based on electric fields are usually limited by the complexity of energy consumption and operating environments. Therefore, we hope to prevent biofouling by the antibacterial treatment of the cathode surface.
Cu2O is widely used as an antifouling coating to prevent biofouling on marine facilities owing to its high efficiency for antibacterial effects.9,10 The sterilization principle is based on the release of copper ions and the production of reactive oxygen species to inhibit bacterial growth.11 Although Cu2O can be used as a biocide to reduce the population of fouling organisms on the cathode surface, the low ORR activity of Cu2O makes it unsuitable for use as an ORR catalyst alone. Cobalt-based zeolitic imidazole framework (ZIF) catalysts have been considered as star electrocatalysts due to their large specific surface area, uniform nitrogen doping and adjustable structure.12,13 In particular, ZIF-derived Co-based catalysts possess Co–N active sites for ORR.14 If Cu2O and Co/NC are combined, it would be possible to design and prepare highly active ORR catalysts that inhibit the growth of biofilms.
In this work, ZIF-derived Cu2O@Co/N-doped carbon (Cu2O@Co/NC) was synthesized to provide Co–N active sites for ORR. Fast mass transport channels of Co/NC resulted from porous carbonized nanocubes. Electron distribution was studied using density functional theory (DFT) calculations to understand the effects of interface. The ORR activity can be estimated by half-wave potential, onset potential and Tafel slope. The antibacterial effect reduced the accumulation of biofilms to facilitate the electron transfer and OH− ion diffusion transport of MFCs. The polarization and power density curves of MFCs were further used to verify the performance of Cu2O@Co/NC as an antibacterial ORR catalyst.
Experimental section
Computational methods
Spin-polarized DFT calculations were performed using the Vienna Ab initio Simulation Package (VASP). The generalized gradient approximation (GGA) with the Perdew–Burke–Ernzerhof (PBE) functional was implemented to evaluate electron exchange correlation and projector augmented wave potentials were used to describe ion-electron interactions.15 The cut-off energy was set to 500 eV and the convergence threshold was conducted as 10−6 eV and 0.02 eV Å−1 for energy and force, respectively. The interaction between neighboring slabs was avoided with a vacuum space of 16 Å. The k-point mesh was selected as 3 × 2 × 1 in the structure optimization using the Monkhorst–Pack method. The adsorption energy (ΔE) is defined as follows:where ET is the initial energy of substrates and EO2 is the energy of O2.
Materials
2-Methylimidazole, cetyltrimethylammonium bromide (CTAB), cobalt nitrate hexahydrate (Co(NO3)2·6H2O), cupric sulphate pentahydrate (CuSO4·5H2O), L-ascorbic acid (AA) and sodium hydroxide (NaOH) were used in the experiments. Carbon cloth (WOS1009) was gained from Carbon Energy Technology Ltd of Taiwan. Electrolytic cells were obtained from Lambert Scientific Instrument Ltd (Beijing, China).
Synthesis
Synthesis of Co/NC: first, 10 mg of CTAB was dissolved in 20 mL ultrapure water with stirring. Then, 0.58 g Co(NO3)2·6H2O was added into this solution with stirring to form a pink solution. The pink solution was poured into 2-methylimidazole (9.08 g)/ultrapure water (140 mL) with constant stirring for 30 min at ambient temperature to obtain a purple precipitate. The purple precipitate was centrifuged and washed six times with ethanol and then dried in a vacuum chamber at 60 °C overnight to obtain ZIF-67. Finally, ZIF-67 was annealed at 550 °C under a N2 atmosphere for 2 h to obtain Co/NC.
Synthesis of Cu2O@Co/NC: Cu2O@Co/NC was prepared by a liquid-phase reduction method (Fig. S1†). First, 0.20 g Co/NC was mixed with 50 mL ultrapure water and dispersed under ultrasonication for 10 min. Then, 0.32 g NaOH was dissolved into 50 mL ultrapure water and mixed with the above-mentioned solution with stirring to form a homogeneous solution. Next, 1.00 g CuSO4·5H2O was introduced to this solution to form Cu(OH)2 precipitates. Finally, 0.70 g AA was added and stirred for 1 h for the reduction of Cu(OH)2 to Cu2O. The precipitates were washed with ethanol followed by vacuum-drying at 60 °C overnight.
Electrochemical measurements
Electrochemical tests were conducted using a CHI760E workstation in a three-electrode system, in which a glassy carbon electrode was used as the working electrode, a Pt foil as the counter electrode and Hg/HgO as the reference electrode, respectively. All of potentials in electrochemical tests were converted to the reversible hydrogen electrode (RHE) according to the following equation: | ERHE = EHg/HgO + 0.059 pH + 0.098 | (2) |
For the rotating disk electrode (RDE), the electron transfer number was calculated from the Koutecky–Levich (K–L) equation as follows:16 | 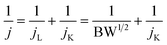 | (3) |
| B = 0.62nFC0D02/3V−1/6 | (4) |
where j, jL, and jK are the measured current density, diffusion limited current density and kinetic current density, respectively, w is the angular velocity of rotation, n represents the electron transfer number, F is the Faradic constant (96
485 C mol−1), C0 is the bulk concentration of O2 (1.2 × 10−6 mol cm−3), D0 is the diffusion coefficient of O2 in solutions (1.9 × 10−5 cm2 s−1), and V is the kinematic viscosity (0.01 cm2 s−1).
Antibacterial activity
Escherichia coli (ATCC 25922) was selected as the antibacterial model to conduct in vitro antibacterial tests. A LB liquid medium and a solid medium were prepared in advance for sterilization. The bacterial solution was diluted to 106 CFU mL−1 with a sterile PBS solution, and then 0.1 mL dilute bacterial solution was inoculated on the LB solid medium. Following this, 20 μL catalyst solution with a concentration of 0.1 g mL−1 was dropped on a 8 mm filter paper affixed on the medium. The blank control group was dropped the same volume of sterile water. The culture dish was placed in a 37 °C incubator for 24 h. The antibacterial activity of the catalyst was characterized by measuring the diameter of the inhibition circle. The tests were repeated three times to ensure the accuracy of the results.
Assembly and operation of MFCs
The air cathode was composed of a catalyst layer, a collector layer (100 mesh titanium mesh) and a gas diffusion layer. First, 90 mg acetylene black, 150 μL 60% polytetrafluoroethylene (PTFE) and 3 mL ethanol were sonicated for 30 min followed by coating on one side of a titanium mesh (3 cm × 3 cm) uniformly. The gas diffusion layer was obtained by heating it to 370 °C for 20 min, which contributed to the diffusion of O2. Then, 36 mg catalyst, 30 mg acetylene black, 50 μL 10% PTFE and 2 mL ethanol were sonicated for 30 min to prepare a catalyst ink. Another side of the titanium mesh was coated with 4 mg cm−2 catalyst as the catalyst layer for subsequent assembly. The anode was made of carbon cloth (2 cm × 3 cm) after pretreatment. The bacterial solution for inoculation was derived from the long-term stable operating dual-chamber MFC. MFCs were operated with an external resistance of 1000 Ω at a constant temperature of 30 °C. The anode electrolyte was renewed when the output voltage dropped below 50 mV. The electrolyte contained CH3COONa (0.5 g L−1), NaH2PO4·2H2O (2.76 g L−1), Na2HPO4·12H2O (11.4 g L−1), NH4Cl (0.3 g L−1) and KCl (1.3 g L−1). The output voltage was recorded every 5 min using a data acquisition instrument (USB-5936, Aertai, China). Power density curves and polarization curves were measured by varying the external resistors.
Results and discussion
DFT calculations
DFT calculations were carried out to investigate the effects of Cu2O on the ORR activity of Co/NC. The Co/NC model consisted of 6 Co,7 N, and 53 C atoms. The Cu2O@Co/NC model was established based on the Co/NC model by adding Cu2O (48 Cu, 24 O atoms) (Fig. S2a,†1a). The effects of Cu2O on the interface charge were described by the charge density difference. Interfacial interaction induced by Cu2O can be observed from electron accumulation and depletion (Fig. 1b). Charge redistribution was induced by strong interfacial interaction to activate catalyst activity. Interfacial charge distribution between Cu2O and Co/NC was further clarified by the Bader charge analysis quantitatively (Fig. S2b,†1c). Co atoms lost more electrons (0.02–0.04e) and N atoms obtained less electrons (0.09–0.13e) owing to the interaction of Cu2O and Co/NC. The charge distribution around Co atoms presented a “claw” shape in Fig. S2c.† Losing more electrons of Co atoms indicated that the valence state of Co atoms on Cu2O@Co/NC was more positive than Co/NC. Oxygen adsorption energy was used to evaluate the O2 adsorption capacity of catalysts. The lower O2 adsorption energy of Cu2O@Co/NC (−1.48 eV) than that of Co/NC (−1.29 eV) implied the stronger oxygen adsorption capacity of Cu2O@Co/NC (Fig. 1d). Compared with Co/NC, Cu2O@Co/NC had an affinity towards O2 recognized as essential to the ORR process. Projected density of states (PDOS) and density of states (DOS) were used to analyze the electronic structure changes of the models. The calculated d-band center of Co for Cu2O@Co/NC (−1.70 eV) was shifted positively than Co/NC (−1.85 eV) (Fig. 1e). The closer the d-band center was to Fermi level, the stronger the binding force between the adsorbate and the substrate.17 The optimized d-band center by introducing Cu2O enhanced the intrinsic ORR catalytic activity. The increase in electronic states near the Fermi level of Cu2O@Co/NC was beneficial to promote electron transfer (Fig. 1f). Hence, the enhanced oxygen absorption and electron transfer accelerated the ORR process.
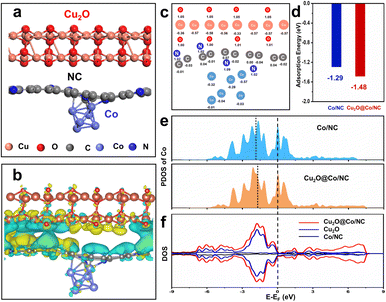 |
| Fig. 1 (a) Cu2O@Co/NC model. (b) Charge density difference of Cu2O@Co/NC, the yellow and cyan regions indicate electron accumulation and depletion. (c) Calculated charge of atoms of Cu2O@Co/NC. (d) O2 adsorption energy of Co/NC and Cu2O@Co/NC. (e) PDOS of the Co 3d orbital in Co/NC and Cu2O@Co/NC. (f) Comparative DOS plots of Cu2O, Co/NC and Cu2O@Co/NC. | |
Characterization
ZIF-67 had a uniform cube shape with a smooth surface (Fig. S3a†). Co/NC maintained the same nanocube-like morphology with a porous and rough surface (Fig. 2a, S3b†). The porous N-doped carbon framework provided accessible active sites and pathways for mass transport. Co nanoparticles were evenly embedded in a N-doped carbon framework. High-resolution transmission electron microscopy (HRTEM) further revealed that the thickness of the carbon layer was about 5 nm (Fig. 2b). The lattice spacing of 0.204 nm was indexed to the (111) plane of metallic Co from the inset image. The carbon layer can avoid the aggregation of Co nanoparticles and further control the size of Co nanoparticles. The interconnected carbon can form a conductive carbon network to promote fast electron transfer. The Co/NC nanocubes were coated by dispersing Cu2O clusters to protect Co/NC from biofouling (Fig. 2c and d). Meanwhile, the electronic states of Co/NC were modulated by dispersing Cu2O, optimizing O2 adsorption energy. Cu2O and Co/NC were tightly connected by a N-doped carbon backbone (Fig. 2e and f). A distinct interface boundary can be observed between Co/NC and Cu2O. The identified lattice spacing of 0.243 nm could be attributed to Cu2O (111) in the HRTEM image. The corresponding elemental analysis showed the distribution of Co, Cu, N and C elements (Fig. 2g). N-doped carbon was distributed uniformly, while Co and Cu2O were distributed inside and outside, respectively. C and N elements distributed uniformly in the N-doped carbon cubes. Co nanoparticles embedded in the N-doped carbon backbone and concentrated at the center of N-doped carbon cubes. Cu elements coated on the surface of Co/NC.
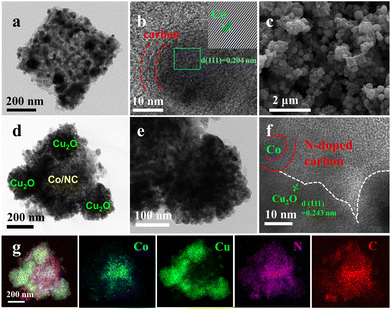 |
| Fig. 2 (a) TEM and (b) HRTEM images of Co/NC. (c) SEM image of Cu2O@Co/NC. (d–f) TEM and HRTEM images of Cu2O@Co/NC. (g) Elemental mapping images of Cu2O@Co/NC. | |
The characteristic diffraction peaks of as-synthesized ZIF-67 are completely consistent with those of simulated ZIF-67 (Fig. S4†). All the samples presented broad diffraction peaks at 21.7° and 22.8° due to agraphitic carbon (Fig. 3a). The diffraction peaks at 44.4° and 51.6° were indexed to the (111) and (200) crystal faces of metallic Co (JCPDS No. 01-1255), respectively. The peaks at 36.4°, 42.3°, 61.5°, and 73.5° were assigned to the (111), (200), (220) and (311) lattice planes of Cu2O (JCPDS No. 05-0667). The peak of Cu2O (111) showed stronger intensity than that of other planes and the Cu2O (111) crystal planes provided dominant active sites for ORR, in accordance with the previous reports in the literature.18 Two obvious peaks at the D band (1370 cm−1) and G band (1590 cm−1) presented the presence of defects and graphitization (Fig. 3b). Cu2O@Co/NC obtained a balance between carbon defects and graphitization, because the relative intensity of D band to G band (ID/IG) value was 1.12.
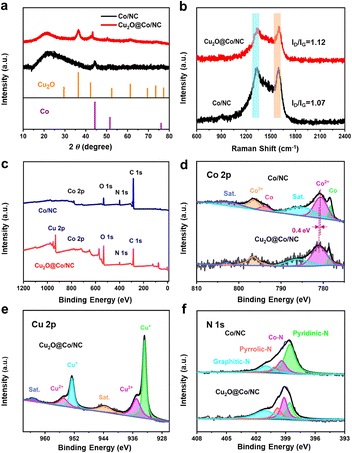 |
| Fig. 3 (a) XRD patterns of Co/NC and Cu2O@Co/NC. (b) Raman spectra of Co/NC and Cu2O@Co/NC. (c) XPS full scan of Cu2O@Co/NC, (d) Co 2p, (e) Cu 2p, (f) N 1s. | |
The XPS survey spectrum of Cu2O@Co/NC demonstrated the presence of C, N, O, Co and Cu elements (Fig. 3c). C 1s can be classified into four different peaks corresponding to C–C/C
C, C–N/C
N, C–O and C
O. The emergence of peaks corresponding to C–N/C
N verified that nitrogen was doped into the carbon matrix. A binding energy of 530.8 eV of Cu2O@Co/NC could be ascribed to Cu–O, suggesting the presence of Cu2O (Fig. S5†). The Co 2p spectrum can be deconvoluted to Co 2p3/2, Co 2p1/2 and two satellite peaks (Fig. 3d). The characteristic peaks of Co2+ (781.0, 796.7 eV), Co0 (778.6, 793.9 eV) and satellite peaks (785.8802.8 eV) were identified in the Co 2p spectrum of Cu2O@Co/NC, while Co2+ (780.6, 796.5 eV), Co0 (778.3, 793.8 eV) and satellite peaks (784.9, 802.1 eV) can be identified for Co/NC. The presence of Co2+ was attributed to the formation of Co–N coordination inherited from ZIF-67, which was ORR active sites.19 Some of Co2+ ions were reduced by the reaction with carbon to form metallic Co. A positive shift in the peak of Co2+ revealed that Cu2O may interact with Co/NC by electron redistribution. The positive shift suggested more electron depletion and higher valence state of Co atoms, which was consistent with DFT calculations. For the Cu 2p region, two peaks located at 932.8 and 952.7 eV could be ascribed to Cu+ in agreement with XRD (Fig. 3e). Binding energies of 935.0 and 954.9 eV were ascribed to Cu2+, which was accredited to the partial oxidation of Cu2O.20 The Cu+-to-Cu2+ ratio was 1.8, suggesting that Cu+ was dominant. Two satellite peaks were observed at 943.6 and 963.3 eV. The high resolution of N 1 s can be deconvoluted into four characteristic peaks with four nitrogen species: pyridinic-N (398.6 eV), Co-N (399.1 eV), pyrrolic-N (399.8 eV) and graphitic-N (401.1 eV) (Fig. 3f). The synergistic effect of nitrogen species can enhance the ORR activity.
Electrochemical activity
All samples exhibited obvious oxygen reduction peaks in O2-saturated 0.1 M KOH, indicating their oxygen reduction activity (Fig. 4a). The comparison of Cu2O@Co/NC cyclic voltammetry (CV) curves in N2- and O2-saturated 0.1 M KOH can clearly distinguish the position of the oxygen reduction peak (Fig. S6a†). The mass ratio of Co/NC and CuSO4 was 1
:
5, and the sample exhibited the best ORR activity (Fig. S6b†). Co/NC, Cu2O@Co/NC and Pt/C displayed oxygen reduction peaks at 0.79, 0.80 and 0.87 V and the corresponding peak current densities were 2.35, 2.75 and 3.59 mA cm−2, respectively. The oxygen reduction peak of Cu2O@Co/NC was more positive than that of Co/NC, and the electrochemical active area of the CV curve was larger than that of Co/NC. The electrochemical active area was increased due to the presence of Cu2O. The peak current density of Cu2O@Co/NC was higher than that of Co/NC, manifesting that the reaction rate of ORR was improved. Linear sweep voltammetry (LSV) was conducted at a rotation speed of 1600 rpm in O2-saturated 0.1 M KOH (Fig. 4b). Cu2O@Co/NC showed a more positive onset potential (Eonset, at 0.2 mA cm−2) of 0.89 V, and a half-wave potential (E1/2) of 0.80 V compared with Co/NC (Eonset = 0.82 V, E1/2 = 0.69 V), which was smaller than 20% Pt/C (Eonset = 1.00 V, E1/2 = 0.86 V).21 Tafel plots were used to reflect ORR kinetics within the mixed kinetic-diffusion controlled region (Fig. 4c).22 The small Tafel slope value indicated fast kinetics for ORR. Cu2O@Co/NC displayed a lower Tafel slope of 62.99 mV dec−1, whereas Co/NC showed Tafel slopes of 69.94 mV dec−1. The comparison of various ORR catalysts activities is presented in Table S2† and the half-wave potentials of ORR catalysts are presented in Fig. S10.† Cu2O@Co/NC demonstrated superior ORR activities.23–28 The oxygen reduction pathway was obtained by different rotation rates (Fig. S7a†). According to the K–L equation, the calculated electron transfer number was 3.4, suggesting that two- and four-electron pathways coexisted and ORR proceeded predominantly via a four-electron reaction pathway (Fig. S7b†). The electrochemical impedance spectrum (EIS) curve of Cu2O@Co/NC showed a smaller semicircle than that of Co/NC, implying a smaller charge transfer resistance (Fig. 4d, Table S1†). Cu2O can contribute electrons to oxygen and obtain electrons from the N-doped carbon backbone, accelerating the electron transfer and reducing the charge transfer resistance. The fast ORR process was linked with the optimized binding energy of oxygen resulting from charge redistribution. The tolerance to methanol crossover effects of Cu2O@Co/NC was obtained by adding 3 M CH3OH into electrolytes (Fig. 4e). The CV curve of Cu2O@Co/NC retained the same shape and showed an obvious oxygen reduction peak, while the CV curve of Pt/C completely deformed. The results revealed that Cu2O@Co/NC had better poison tolerance than that of Pt/C, which played a pivotal role for MFCs. The CV scans of catalysts in the non-Faraday region with different sweep speeds can reflect double-layer capacitance (Cdl) (Fig. S8†). Electrochemical surface areas (ECSAs) were evaluated with Cdl in the potential range without ORR occurring (Fig. 4f). The calculated Cdl value of Cu2O@Co/NC (15.17 mF cm−2) was higher than that of Co/NC (7.62 mF cm−2), signaling that Cu2O@Co/NC possessed more active sites.
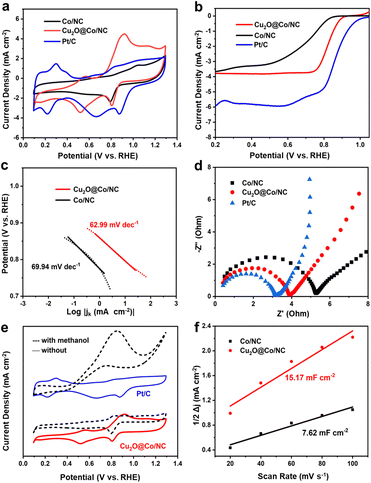 |
| Fig. 4 (a) CV curves in O2-saturated 0.1 M KOH. (b) LSV curves of catalysts in 0.1 M KOH at a rotation speed of 1600 rpm. (c) Tafel plots of catalysts (d) Nyquist curves of Cu2O@Co/NC, Co/NC and Pt/C. (e) Resistance to poisoning of Cu2O@Co/NC and 20 wt% Pt/C. (f) Double-layer capacity of the catalysts. | |
Antibacterial activity
The performance of MFCs is greatly affected by biofouling on the cathode surface due to the increasing internal resistance and impeding mass transport. Cathodes with antibacterial properties can effectively inhibit the formation of biofilms. The inhibition zone method was used to assess the antibacterial activity of cathode catalysts. The bacteriostatic ring diameter of Cu2O@Co/NC was about 2 mm, while Co/NC did not show any obvious bacteriostatic ring under the same condition (Fig. 5). Cu2O@Co/NC exhibited good potential to inhibit the formation of cathode biofilms due to the antibacterial effect of Cu2O.
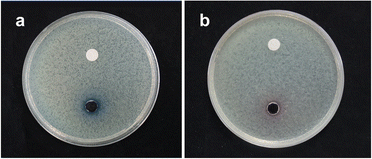 |
| Fig. 5 Photographs of Escherichia coli (ATCC25922) cultured on a solid medium with 20 μL of 0.1 g mL−1 Cu2O@Co/NC (a) and Co/NC (b). | |
Performances of MFCs
The output voltage of Cu2O@Co/NC was 0.43 V, which was higher than that of Co/NC and comparable to commercial 20 wt% Pt/C (Fig. 6a). The output voltage of Pt/C was highest in the first cycle corresponding to the oxygen reduction activity among the catalysts. The maximum output voltage of Pt/C decreased from 0.46 to 0.42 V after four cycles, while Cu2O@Co/NC remained stable at about 0.43 V. The voltage of Pt/C dropped rapidly in view of poor poison resistance and inactivation of the Pt/C catalyst. The thicker biofilm formed on the cathode of Co/NC after 450 h operation proved that Cu2O@Co/NC can inhibit the excessive growth of biofilms (Fig. 6b). The power density and polarization data were obtained when the output voltage was stable after 450 h of operation. The maximum power density observed with Cu2O@Co/NC was 1100 mW m−2 at a high current density of 4.46 A m−2, compared to that of Co/NC (739 mW m−2) and Pt/C (1067 mW m−2) (Fig. 6c). The maximum power density of Cu2O@Co/NC was noteworthy compared to those of reported catalysts based on metal carbon material (Table S3†). When the power density reached the maximum, the external resistance was equal to the internal resistance of MFCs. The internal resistance of Cu2O@Co/NC (280 Ω) was lower than that of Co/NC (440 Ω) and Pt/C (300 Ω). The voltages of Cu2O@Co/NC, Pt/C and Co/NC decreased with the increase in current density (Fig. 6d). The polarization curves of Cu2O@Co/NC and Pt/C exhibited similar trends, implying similar electrode catalysis processes. The voltage decreased rapidly at a current density of 2.8 A m−2 for Co/NC. The voltage decrease was attributed to the internal resistance and hampered OH− diffusion, which was caused by the growth of biofilms on the cathode surface. The open circuit voltage of Cu2O@Co/NC was the largest. Cu2O@Co/NC with a high potential and stable power generation benefitted from the high oxygen reduction activity and efficient antifouling property. Cu2O was oxidized to CuO by O2, and CuO was reduced to Cu2O by obtaining electrons in the solution to form a redox cycle, which facilitated electron transfer.
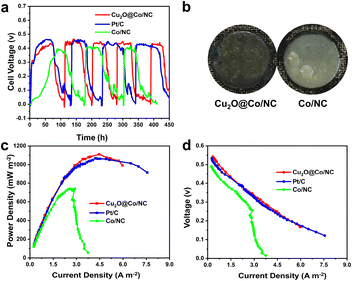 |
| Fig. 6 (a) Output voltages. (b) Biofilm on the cathode surface after 450 h of operation. (c) Power density of the MFCs. (d) Polarization curves of the MFCs. | |
The mechanisms of bacterial inhibition are proposed as follows: (1) the catalyst can produce reactive oxygen species (O2−˙, OH−˙, and H2O2) to cause oxidative stress via a Fenton-like reaction, (2) the catalyst may prevent cell replication by binding with DNA or specific proteins and (3) positively charged Cu species can contact with negatively charged groups to destroy membrane integrity and disrupt cell functions.9,29,30 These mechanisms could help understand the pathways for the contact killing of bacteria by antibacterial catalysts.
Conclusions
In summary, the efficient and stable operation of MFCs attributed to the high efficiency of ORR process. The high ORR activity stemmed from strengthening O2 adsorption of Cu2O@Co/NC induced by charge redistribution. Cu2O@Co/NC showed enhanced half-wave potential, onset potential and low Tafel slope. Cu2O can effectively inhibit the excessive growth of biofilms, promoting electron transfer and OH− ion diffusion transport in MFCs. Effective electron transfer and OH− ion diffusion transport decreased internal resistance and concentration difference. The possible antibacterial mechanisms of Cu2O@Co/NC included oxidative stress, prevention of replication and destruction of the membrane integrity of bacteria. Cu2O@Co/NC as an antibacterial ORR catalyst exhibited a low overpotential and a high power density in MFCs. This work provides a synthetic guidance to design and prepare cathode ORR catalysts with high efficiency and practicality.
Author contributions
All authors have given approval to the final version of the manuscript.
Conflicts of interest
There are no conflicts to declare.
Acknowledgements
This work was financially supported by the Key R&D Program of Ministry of Science and Technology of China (2018YFC1803100), Youth Project of Science & Technology Research Program of Chongqing Education Commission of China (KJQN201901208). We also thank the Big Data Center of Southeast University for providing the facility support on the numerical calculations.
Notes and references
- B. E. Logan, B. Hamelers, R. Rozendal, U. Schroder, J. Keller, S. Freguia, P. Aelterman, W. Verstraete and K. Rabaey, Microbial fuel cells: methodology and technology, Environ. Sci. Technol., 2006, 40, 5181–5192 CrossRef CAS PubMed
.
- A. J. Slate, K. A. Whitehead, D. A. C. Brownson and C. E. Banks, Microbial fuel cells: An overview of current technology, Renewable Sustainable Energy Rev., 2019, 101, 60–81 CrossRef CAS
.
- G. Palanisamy, H.-Y. Jung, T. Sadhasivam, M. D. Kurkuri, S. C. Kim and S.-H. Roh, A comprehensive review on microbial fuel cell technologies: Processes, utilization, and advanced developments in electrodes and membranes, J. Cleaner Prod., 2019, 221, 598–621 CrossRef CAS
.
- Y. Yuan, S. Zhou and J. Tang, In situ investigation of cathode and local biofilm microenvironments reveals important roles of OH− and oxygen transport in microbial fuel cells, Environ. Sci. Technol., 2013, 47, 4911–4917 CrossRef CAS PubMed
.
- M. T. Noori, M. M. Ghangrekar, C. K. Mukherjee and B. Min, Biofouling effects on the performance of microbial fuel cells and recent advances in biotechnological and chemical strategies for mitigation, Biotechnol. Adv., 2019, 37, 107420 CrossRef CAS PubMed
.
- X. Jin, N. Yang, Y. Liu, F. Guo and H. Liu, Bifunctional cathode using a biofilm and Pt/C catalyst for simultaneous electricity generation and nitrification in microbial fuel cells, Bioresour. Technol., 2020, 306, 123120 CrossRef CAS PubMed
.
- S. C. Popat, D. Ki, B. E. Rittmann and C. I. Torres, Importance of OH(−) transport from cathodes in microbial fuel cells, ChemSusChem, 2012, 5, 1071–1079 CrossRef CAS PubMed
.
- M. J. Al Lawati, T. Jafary, M. S. Baawain and A. Al-Mamun, A mini review on biofouling on air cathode of single chamber microbial fuel cell; prevention and mitigation strategies, Biocatal. Agric. Biotechnol., 2019, 22, 101370 CrossRef
.
- W. Wu, W. Zhao, Y. Wu, C. Zhou, L. Li, Z. Liu, J. Dong and K. Zhou, Antibacterial behaviors of Cu2O particles with controllable morphologies in acrylic coatings, Appl. Surf. Sci., 2019, 465, 279–287 CrossRef CAS
.
- H. Li, S. Luo, L. Zhang, Z. Zhao, M. Wu, W. Li and F. Q. Liu, Water- and acid-sensitive Cu2O@Cu-MOF nano sustained-release capsules with superior antifouling behaviors, ACS Appl. Mater. Interfaces, 2022, 14, 1910–1920 CrossRef CAS PubMed
.
- Z. Yang, X. Hao, S. Chen, Z. Ma, W. Wang, C. Wang, L. Yue, H. Sun, Q. Shao, V. Murugadoss and Z. Guo, Long-term antibacterial stable reduced graphene oxide nanocomposites loaded with cuprous oxide nanoparticles, J. Colloid Interface Sci., 2019, 533, 13–23 CrossRef CAS PubMed
.
- Z. Wang, W. Xu, X. Chen, Y. Peng, Y. Song, C. Lv, H. Liu, J. Sun, D. Yuan, X. Li, X. Guo, D. Yang and L. Zhang, Defect-rich nitrogen doped Co3O4/C porous nanocubes enable high-efficiency bifunctional oxygen electrocatalysis, Adv. Funct. Mater., 2019, 29, 1902875 CrossRef
.
- Z. Liang, N. Kong, C. Yang, W. Zhang, H. Zheng, H. Lin and R. Cao, Highly curved nanostructure-coated Co, N-doped carbon materials for oxygen electrocatalysis, Angew. Chem., Int. Ed., 2021, 60, 12759–12764 CrossRef CAS
.
- Y. Ha, B. Fei, X. Yan, H. Xu, Z. Chen, L. Shi, M. Fu, W. Xu and R. Wu, Atomically dispersed Co-Pyridinic N-C for superior oxygen reduction reaction, Adv. Energy Mater., 2020, 10, 2002592 CrossRef CAS
.
- Á. Valdés, Z. W. Qu, G. J. Kroes, J. Rossmeisl and J. K. Nørskov, Oxidation and photo-oxidation of water on TiO2 surface, J. Phys. Chem. C, 2008, 112, 9872–9879 CrossRef
.
- P. Yin, T. Yao, Y. Wu, L. Zheng, Y. Lin, W. Liu, H. Ju, J. Zhu, X. Hong, Z. Deng, G. Zhou, S. Wei and Y. Li, Single cobalt atoms with precise N-coordination as superior oxygen reduction reaction catalysts, Angew. Chem., Int. Ed., 2016, 55, 10800–10805 CrossRef CAS PubMed
.
- J. K. Norskov, F. Abild-Pedersen, F. Studt and T. Bligaard, Density functional theory in surface chemistry and catalysis, Proc. Natl. Acad. Sci. U. S. A., 2011, 108, 937–943 CrossRef CAS PubMed
.
- X. Zhang, K. Li, P. Yan, Z. Liu and L. Pu, N-type Cu2O doped activated carbon as catalyst for improving power generation of air cathode microbial fuel cells, Bioresour. Technol., 2015, 187, 299–304 CrossRef CAS PubMed
.
- F. Hu, H. Yang, C. Wang, Y. Zhang, H. Lu and Q. Wang, Co-N-doped mesoporous carbon hollow spheres as highly efficient electrocatalysts for oxygen reduction reaction, Small, 2017, 13, 1602507 CrossRef PubMed
.
- Y. Guo, M. Dai, Z. Zhu, Y. Chen, H. He and T. Qin, Chitosan modified Cu2O nanoparticles with high catalytic activity for p-nitrophenol reduction, Appl. Surf. Sci., 2019, 480, 601–610 CrossRef CAS
.
- X. Feng, Q. Jiao, W. Chen, Y. Dang, Z. Dai, S. L. Suib, J. Zhang, Y. Zhao, H. Li and C. Feng, Cactus-like NiCo2S4@NiFe LDH hollow spheres as an effective oxygen bifunctional electrocatalyst in alkaline solution, Appl. Catal., B, 2021, 286, 119869 CrossRef CAS
.
- X. Huang, Y. Wang, W. Li and Y. Hou, Noble metal-free catalysts for oxygen reduction reaction, Sci. China: Chem., 2017, 60, 1494–1507 CrossRef CAS
.
- C. Chen, Z. J. Tang, J. Y. Li, C. Y. Du, T. Ouyang, K. Xiao and Z. Q. Liu, MnO enabling highly efficient and stable Co-Nx/C for oxygen reduction reaction in both acidic and alkaline media, Adv. Funct. Mater., 2022, 202210143 Search PubMed
.
- Z. Wang, J. Huang, L. Wang, Y. Liu, W. Liu, S. Zhao and Z. Q. Liu, Cation-Tuning Induced d-band center modulation on Co-based spinel oxide for oxygen reduction/evolution reaction, Angew. Chem., Int. Ed., 2022, 61, e202114696 CAS
.
- S.-J. Li, Y. Xie, B.-L. Lai, Y. Liang, K. Xiao, T. Ouyang, N. Li and Z.-Q. Liu, Atomic modulation of Fe-Co pentlandite coupled with nitrogen-doped carbon sphere for boosting oxygen catalysis, Chin. J. Catal., 2022, 43, 1502–1510 CrossRef CAS
.
- X. T. Wu, L. J. Peng, K. Xiao, N. Li and Z. Q. Liu, Rational design and synthesis of hollow Fe-N/C electrocatalysts for enhanced oxygen reduction reaction, Chem. Commun., 2021, 57, 5258–5261 RSC
.
- L. Wang, X. Jin, J. Fu, Q. Jiang, Y. Xie, J. Huang and L. Xu, Mesoporous non-noble metal electrocatalyst derived from ZIF-67 and cobalt porphyrin for the oxygen reduction in alkaline solution, J. Electroanal. Chem., 2018, 825, 65–72 CrossRef CAS
.
- Z. Lu, B. Wang, Y. Hu, W. Liu, Y. Zhao, R. Yang, Z. Li, J. Luo, B. Chi, Z. Jiang, M. Li, S. Mu, S. Liao, J. Zhang and X. Sun, An Isolated Zinc-Cobalt Atomic Pair
for Highly Active and Durable Oxygen Reduction, Angew. Chem., Int. Ed., 2019, 58, 2622–2626 CrossRef CAS PubMed
.
- A. M. EI Saeed, M. A. El-Fattah, A. M. Azzam, M. M. Dardir and M. M. Bader, Synthesis of cuprous oxide epoxy nanocomposite as an environmentally antimicrobial coating, Int. J. Biol. Macromol., 2016, 89, 190–197 CrossRef PubMed
.
- S. Meghana, P. Kabra, S. Chakraborty and N. Padmavathy, Understanding the pathway of antibacterial activity of copper oxide nanoparticles, RSC Adv., 2015, 5, 12293–12299 RSC
.
|
This journal is © The Royal Society of Chemistry 2023 |