Distinguishing the nanoplastic–cell membrane interface by polymer type and aging properties: translocation, transformation and perturbation†
Received
30th August 2022
, Accepted 18th November 2022
First published on 21st November 2022
Abstract
Nanoscale plastics, being ubiquitous in the environment, can cross biological barriers primarily through interactions with the cell membrane; yet there remains a lack of knowledge about the mechanisms regulating the nanoplastic–cell membrane interface. Herein, we systematically describe nanoplastics of five common materials and three aging properties and reveal that interfacial processes including nanoplastic translocation, transformation and membrane perturbation are governed by the competition of polymer–polymer and polymer–lipid interactions. Molecular simulations suggest spontaneous insertion of polystyrene and polyethylene nanoplastics into the membrane, where some kinds of lipids are adsorbed and form monolayered coronas, increasing the nanoplastic's effective size, reducing its hydrophobicity, and endowing it with a negative surface charge. Polyethylene terephthalate nanoplastics of lower hydrophobicity readily penetrate through the membrane with fewer lipids adsorbed. Polypropylene and polyvinyl chloride nanoplastics dissolve in the membrane to retard their translocation and aggravate membrane perturbation. Among aging properties, decreasing size facilitates nanoplastic dissolution, and fiber-shaped nanoplastics easily translocate from the tip first. Different molecules are rearranged and exchanged at the charged nanoplastic–cell membrane interface to alter nanoplastic translocation and membrane remodeling. Such distinctive interactions reduce membrane fluidity and rigidity, making it less stable and apt to collapse under stress. These results uncover key roles of the polymer type and aging properties in nanoplastic fate and toxicity to mammalian cells.
Environmental significance
Cellular processes of nanoplastics start from the membrane surface, where the nature of their interactions determines whether and how nanoplastics enter cells, induce membrane damage, and are transformed to alter their fate inside/outside the cell. However, the mechanisms governing the nanoplastic–cell membrane interface remain unclear and are difficult to study at the molecular level in vivo. In this computational effort, nanoplastics of five common materials and three aging properties are employed to systematically examine their interactions with a model mammalian cell membrane. Molecular dynamics simulations identify the distinctive behaviors of nanoplastics at the cell membrane surface and reveal processes of membrane translocation, nanoplastic transformation and membrane perturbation determined by the competition of polymer–polymer and polymer–lipid interactions. These results are integrated to form molecular insights into nanoplastic bioavailability, distribution, clearance and toxicity to mammalian cells, in which the polymer type and aging properties significantly matter.
|
1 Introduction
In the past few years, plastic pollution has become a global threat to the ecoenvironment and human health. The global production of plastics has increased from 1.5 million tons in 1950 to 367 million tons in 2020,1 and the number keeps increasing. It was estimated that around 10% of the plastics produced every year end up as debris in the environment,2,3 and plastic debris further disintegrates over time into microplastics and eventually nanoplastics as a result of UV radiation, mechanical abrasion, and biological degradation.4 Recently, as high as 16
200
000 micro(nano)plastics per liter were detected from polypropylene infant feeding bottles,5 and billions of micro(nano)plastics were detected in one cup of tea, which were released from a plastic teabag.6 Furthermore, the emergence of COVID-19 has caused worldwide use of face masks to result in an increased possibility of human exposure to micro(nano)plastics.7 Therefore, it is urgent to assess what potential risks micro(nano)plastics bring to human health.8
Although considerable efforts have been made in the detection and risk assessment of microplastics,9,10 very limited information about the fate and distribution of nanoplastics in biological tissues is available due to analytical challenges associated with in situ detection and characterization.11–14 Nanoplastics differ distinctly from microplastics in physicochemical properties and biological reactivity.15,16 The much smaller size makes the abundance of nanoplastics in the environment likely several orders of magnitude higher than that of microplastics. Moreover, smaller nanoplastics easily cross biological barriers,17 thus exhibiting a wider and deeper distribution inside the body, posing a higher risk to the human health.18 However, the lack of methodologies in collection and separation of nanoplastics from environmental and biological samples compelled researchers to use engineered polystyrene (PS) nanospheres,19–24 which are distinct from incidental nanoplastics of diverse materials and aging properties. Therefore, our understanding about the nanoplastic behaviors in biological systems is quite inadequate.
Among possible portals, ingestion and inhalation are two major routes of human exposure to micro(nano)plastics.25–29 Our previous study revealed suppression of lipid digestion by micro(nano)plastics through reducing lipase activity and decreasing the bioavailability of lipid droplets.30 Larger microplastics are usually trapped in the gut after ingestion by a wide variety of species,31 while nanoplastics easily cross the gut barrier and translocate to secondary tissues after ingestion.17,32 Recently, PS nanospheres were found to reach and deposit in the brain of mice via aerosol inhalation and induce severe neurotoxicity through interactions with neural cells.33 Upon entering the blood circulation, which was recently evidenced,34 nanoplastics are expected to reach other organs and cells to generate toxic effects via inducing oxidative stress, membrane damage and mitochondrial dysfunction.20,35 In particular, the plasma membrane is an important barrier against nanoplastic invasion into cells,36 and the nature of their interactions determines whether and how nanoplastics enter cells, induce membrane damage, and are transformed to alter their fate inside/outside the cell. Although the interfacial behaviors of engineered PS nanospheres resemble that of traditional nanoparticles as have been intensively studied,37–43 environmental nanoplastics are diverse in polymer type and experience complex aging processes to further increase heterogeneities in size, shape and surface property. We ask how nanoplastics of different materials and aging properties interact with the cell membrane, and what outcomes are these interactions related to nanoplastic biodistribution, biotransformation, clearance and toxicity.
Tailored computer simulation has become an indispensable tool for revealing molecular mechanisms underlying the bio–nano interface.44–46 In this study, we adopted molecular dynamics (MD) simulation to probe nanoplastic interactions with an idealized mammalian cell membrane. Based on most previous studies using synthesized PS nanospheres as the representative model, we prepared a family of nanoplastics of PS, polyethylene (PE), polyethylene terephthalate (PET), polypropylene (PP) and polyvinyl chloride (PVC), which are most commonly detected in the environment.47,48 The nanoplastic size, shape and surface charge were considered as three aging properties. Processes of nanoplastic translocation and dissolution, lipid adsorption and membrane perturbation were comparatively analyzed, with all results integrated to form implications relevant to the nanoplastic's fate and toxicity to mammalian cells.
2 Materials and methods
2.1 Molecular models and system setup
Simulations based on the molecular dynamics (MD) method were performed using the GROMACS 2019 software package.49 To capture sufficient details at the molecular level while extending time and length scales to be appropriate for simulating nanoplastic behaviors at the cell membrane surface, the widely used Martini coarse-grained (CG) force field was adopted.50 Fig. S1A† shows the atomistic and corresponding CG models of different polymers used in simulations. CG force field parameters for beads in PS, PE and PP have been established and validated by previous simulations,51,52 and those for beads in PET and PVC were tuned based on their molecular structures and similarities with other polymers.53 Bisphenol A (BPA) molecules were considered as model additives and transformed to CG models using a similar procedure.
Nanoplastics of different materials were constructed by adopting the melting–annealing procedure.53,54 For each material, a number of polymers and BPA molecules of 0.05% mass ratio were randomly positioned in vacuum to start a simulation with the NVT ensemble. The temperature was set initially above the melting point to achieve a high-mobility melting state with sufficient crosslinking of polymer chains (Fig. S2†). Then the system was annealed with a constant velocity of 0.01 K ps−1 until the glass-transition temperature was reached (melting and glass-transition temperatures for five considered polymers are listed in Table S1†). After acquiring equilibrium structures, the system was solvated and further equilibrated at room temperature for 100 ns. Nanoplastic size was tuned by changing the number of polymers, and two sizes, including 10 nm and 5 nm, were considered in this study. To examine the effect of nanoplastic shape, a number of PE chains were arranged into a fiber shape, which was then equilibrated in air and water. The termini of polymers at the nanoplastic surface were grafted with cationic and anionic groups to represent positive and negative surface charges (Fig. S1B†).
Different from previous studies using bilayers consisting of, at most, four or five lipid components, here we used an idealized mammalian cell membrane model developed by Ingólfsson et al.55 This membrane of 44 nm × 44 nm in lateral size contains phosphatidylcholines (PCs), sphingomyelin (SM) and gangliosides (GMs) predominantly in the outer leaflet and phosphatidylethanolamine (PE), phosphatidylserine (PS), and other charged lipids in the inner leaflet (Fig. S1C†). Combination of different headgroups and lipid acyl chains resulted in 63 different lipid species asymmetrically distributed across two leaflets (Fig. S1D†). A number of Na+ and Cl− ions were added to neutralize the system and represent the ionic strength of 0.15 M.
2.2 Details of MD simulation
Unbiased MD simulations of nanoplastic–membrane interactions were performed with the NPT ensemble using the semi-isotropic Berendsen barostat (P = 1 bar) with a coupling constant of 4 ps and compressibility of 5 × 10−5 bar−1 in both lateral and membrane normal directions. The temperature was kept constant at T = 310 K using a V-rescale thermostat with a coupling constant of 4 ps. Van der Waals interactions were calculated using a cutoff of 1.2 nm, with the Lennard-Jones potential smoothly shifted to zero between 0.9 nm and 1.2 nm to reduce the cutoff noise. The coulombic potential, with a cutoff of 1.2 nm, was smoothly shifted to zero from 0 nm to 1.2 nm. Long-range electrostatic interactions were treated using the particle mesh Ewald summation method. The time step of simulations was 10 fs, with the neighbor list updated every 10 steps. Periodic boundary conditions were adopted in all three directions. Simulated snapshots were rendered using VMD.56 To elucidate the effects of polymer type and aging properties on the nanoplastic translocation through the cell membrane, steered MD simulations were also conducted. An external force of spring constant 2000 kJ mol−1 nm−2 was exerted on each nanoplastic center to pull it across the membrane at a constant velocity of 0.001 nm ps−1.57
3 Results
3.1 Cell membrane interactions with nanoplastics of different materials
Fig. 1 lists the time sequences of snapshots depicting cell membrane processes of nanoplastics of five considered materials. Apparently, the manner of nanoplastic–membrane interactions is strongly dependent on the polymer type. PS and PE nanoplastics rapidly translocate into membrane (Fig. 1A and B and Videos S1 and S2†) and acquire favorable contacts with hydrophobic lipid tails (Fig. S3A†). Due to mismatch between the nanoplastic size and the membrane thickness, numerous lipids are extracted and cover the nanoplastics from the top side. Distinct from PS and PE nanoplastics, PET nanoplastic directly pierces through the membrane (Fig. 1C and Video S3†). Due to PET's lower hydrophobicity as previously measured,58 surrounding lipids are not extracted but repelled for translocation (Fig. S3A†), reminiscent of small hydrophilic nanoparticles proved to directly penetrate through the membrane.38,59,60 PS, PE and PET nanoplastics remain intact during interactions with the membrane, whereas PP nanoplastics dissolve in the membrane to get more favorable contacts with lipid tails (Fig. 1D and S3A and Video S4†), causing apparent increases of the nanoplastic gyration radius and the polymer–polymer interaction energy (Fig. S3B and C†). PVC nanoplastic shows a similar but lower degree of dissolution (Fig. 1E and Video S5†). With prolonged simulation time, PP and PVC nanoplastics are surmised to dissolve completely inside the membrane, and toxic additives (e.g., BPAs) are released to increase their bioavailability (Fig. S4†).
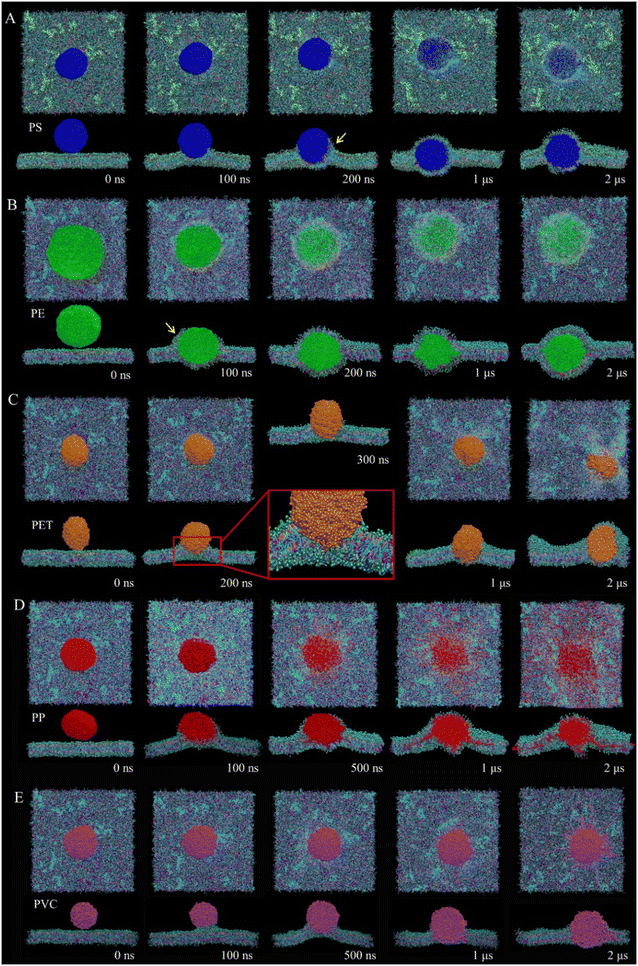 |
| Fig. 1 Distinguishing cell membrane interactions with PS (A), PE (B), PET (C), PP (D) and PVC (E) nanoplastics. Both top and cross-sectional views are provided, and solvent molecules are not displayed for clarity. Yellow arrows denote lipid extraction by PS and PE nanoplastics. | |
The membrane plays a physical barrier role against nanoplastic cell entry, and the equilibrium states of nanoplastic–cell membrane interactions can be regarded as the preparative step for subsequent internalization.61 Finite energy barriers usually exist due to membrane remodeling and integrity41 and can be overcome by forces generated from actin polymerization or membrane fluctuation.62 To comparatively evaluate the cell entry capacity of different nanoplastics, the equilibrium states of nanoplastic–membrane interactions acquired above were set as the initial structures for running steered simulations. Comparing the time evolutions of the resistant force and typical snapshots (Fig. 2 and S5†), the nanoplastic cell entry is strongly influenced by the early state of nanoplastic–cell membrane interactions, which essentially depend on the polymer type. PET nanoplastic, which was identified to directly pierce the membrane with fewer lipids adsorbed, accomplishes cell entry with the lowest energy cost. PS and PE nanoplastics, in contrast, adsorb more lipids to hinder their translocation. As expected, membrane translocation of the PP nanoplastic has the highest force barrier due to its dissolution. Dissolved polymers acquire more favorable contacts with surrounding lipids to trap nanoplastics inside the membrane. Of note, PET nanoplastics were more frequently detected in human blood than other types of nanoplastics,34 indicating that inhaled or ingested PET nanoplastics could readily cross the alveolar or gut barriers to enter blood circulation. Our simulations support this speculation and explain from the molecular level that fewer hydrophobic PET nanoplastics easily translocate directly through the membrane and accomplish cell uptake. In contrast, PP nanoplastics are easily trapped inside the membrane with a lower capacity of cell uptake, and long-term membrane perturbation could be induced as will be discussed later.
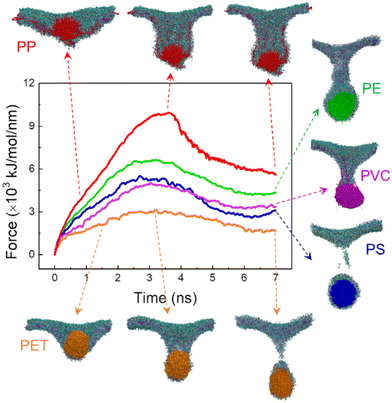 |
| Fig. 2 Time evolutions of the resistant force during mechanical translocation of different nanoplastics across the membrane. Typical snapshots are displayed from the cross-sectional view to illustrate structural change during translocation. | |
3.2 Molecular structure and composition of lipid coronas formed on nanoplastics
Nanoplastics experience transformations during interactions with the cell membrane, which can be represented as nanoplastic dissolution and lipid adsorption. In particular, lipids are adsorbed to form coronas expected to change the nanoplastic's physicochemical properties. Fig. 3A shows cross-sectional views of lipid coronas formed on PS and PE nanoplastics. To quantitatively describe the molecular structures of lipid adsorption on different nanoplastics, the number densities of lipid head and tail groups as a function of distance to the nanoplastic center were calculated (Fig. 3B–F). For PS and PE nanoplastics, which were estimated to adsorb more lipids during interactions with the membrane (Fig. 1A and B), the density of lipid headgroups shows single peaks peripheral to the lipid tails attached to the nanoplastic surface (Fig. 3B and C). It clearly manifests a monolayer conformation of approximately 3 nm in thickness, and lipid headgroups are preferentially exposed to represent the altered surface property. The sharper peak of the lipid density distribution suggests a tighter lipid arrangement on the PE nanoplastic (Fig. 3C), as also displayed in the snapshot (Fig. 3A). The monolayer structural feature is insignificant for PET, PP and PVC nanoplastics (Fig. 3D–F), which show distinct modes of cell membrane interactions. In addition, dissolution of PP and PVC nanoplastics is further evidenced by apparent decreases of the polymer density especially near the nanoplastic surface (Fig. 3E and F).
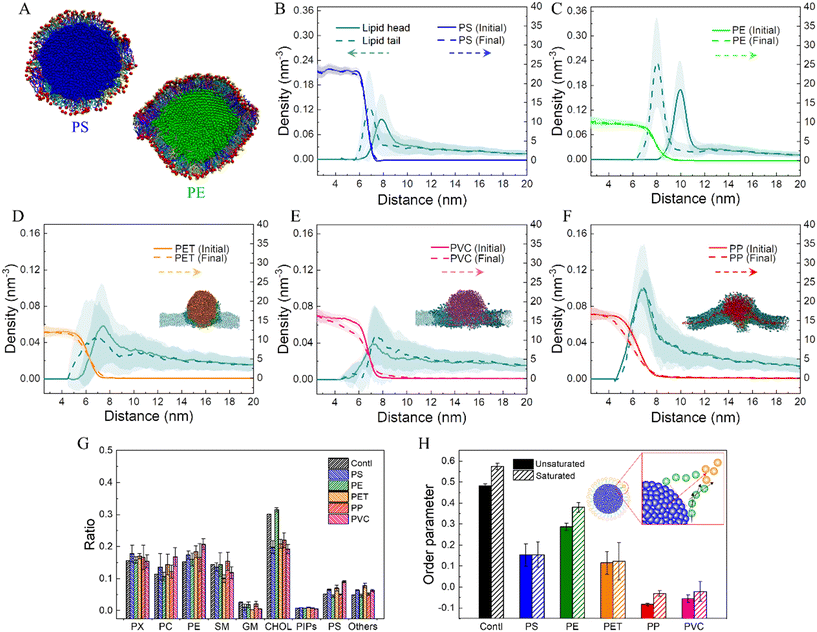 |
| Fig. 3 Chemical compositions and molecular structures of lipid coronas formed on nanoplastics. (A) Cross-sectional views of lipid coronas formed on PS and PE nanoplastics. Lipids are displayed in different colors according to the headgroup charge, tail length and saturation degree. (B–F) Number densities of lipid head (cyan solid lines) and tail (cyan dashed lines) groups as functions of their distances from the center of PS (B), PE (C), PET (D), PVC (E) and PP (F) nanoplastics. The number density distributions of polymers at initial (solid lines) and final (dashed lines) states are compared to illustrate the structural change of nanoplastics before and after membrane interactions. (G) Quantitative comparison of the ratios of lipid components adsorbed on different nanoplastics and those in the bulk membrane. Two beads were defined as in contact if their distance is smaller than 0.6 nm. (H) Order parameters of unsaturated and saturated lipids adsorbed on different nanoplastics. The order parameter was calculated using the equation , where θ is the angle between the normal direction at the adsorption site of the nanoplastic surface and the vector connecting the two adjacent beads, as illustrated in the inset of H. | |
Much effort has been made in analyzing protein corona composition on various nanoparticles in culture medium.63–65 In contrast, scarce knowledge is available about lipid coronas formed on nanoplastics during their interactions with mammalian cell membranes of equal complexity.66 The current mammalian cell membrane model consists of 63 lipid species with different headgroups, tail lengths and saturation degrees (Fig. S1C and D†), thus allowing us to comparatively analyze the composition of lipid coronas on nanoplastics. Clearly, lipids adsorbed on PS and PE nanoplastics are multi-component, as displayed in the snapshots (Fig. 3A). To quantitatively identify corona compositions on nanoplastics, the numbers of different molecules in contact with nanoplastics were calculated (Fig. 3G). Data show that most lipid compositions in coronas are close to the bulk membrane, except that the ratio of cholesterol molecules adsorbed on the PE nanoplastic was much larger than that in contact with other nanoplastics. The molecular structures of coronas were further analyzed by calculating the lipid order parameter on nanoplastics (Fig. 3H). Compared to the bulk membrane, lipids adsorbed on nanoplastics are less ordered, while lipids on PE nanoplastic show relatively high-order parameters, consistent with the tighter lipid arrangement as measured by radial distribution function (Fig. 3B). Due to the higher crystallinity as previously measured,67 PE nanoplastics usually have smoother surfaces favoring tight and ordered lipid arrangement. It can be learned from these results that lipid coronas can increase the nanoplastic's effective size, reduce its hydrophobicity, and endow nanoplastics with a slightly negative surface charge, all of which could influence the nanoplastic's fate inside and outside the cell.
3.3 Membrane perturbation by nanoplastics
The ultrastructure of lipids around nanoplastics was characterized by calculating the lipid density distributions from the top and cross-sectional views (Fig. S6A and C†). Combining snapshots (Fig. S6B and D†), the extent of ultrastructural perturbation induced by nanoplastics is closely related to the manner of interactions, which is essentially determined by the polymer type. PS and PE nanoplastics are encapsulated inside the membrane, and the local patch is split into two monolayers covering the upper and lower sides of nanoplastics. The highest lipid density locates at the junctional area between nanoplastics and the membrane, suggesting strong hydrophobic interactions between nanoplastics and lipid tails. PET nanoplastic of lower hydrophobicity repels surrounding lipids to generate a hydrophilic pore underneath, and this transient defect can be recovered after nanoplastic translocation. PP and PVC nanoplastics induce severe perturbation through protruding numerous polymers to the membrane interior. Both intact and dissolved nanoplastics diffuse much slower in the membrane than the lipid components, thus reducing the membrane fluidity as characterized by decreases of the lipid diffusion coefficients (Table S2†). In accordance with the ultrastructure perturbation, the membrane compressibility modulus and bending modulus are decreased, indicating that membranes incorporated with nanoplastics become less stable and apt to deform and even collapse under stress. Of all considered materials, PP nanoplastics show the highest degree of ultrastructure and mechanical perturbation, and such damage could be long-standing because PP nanoplastics are more difficult to remove and are trapped in the membrane due to dissolution.
3.4 Aging nanoplastic translocation in the cell membrane
Micro(nano)plastics continuously disintegrate into smaller particles until fully degraded in the environment, and size was expected as a primary aging property determining the cellular process of nanoplastics.68,69 In our simulations, smaller nanoplastics of 5 nm equal to the membrane thickness rapidly translocate into the membrane. In the finite simulation period, only PE and PET nanoplastics remain intact, whereas PS, PP and PVC nanoplastics are all dissolved to a higher extent than larger nanoplastics (Fig. 4A). Enhanced dissolution may aggravate membrane perturbation as has been proved (Fig. S6 and Table S2†). To our knowledge, there is currently no experimental result evidencing dissolution of nanoplastics in biological tissues, which can be explained by two possible reasons. One is that most experimental studies used PS nanoplastics, which showed higher stability than PP and PVC nanoplastics as illustrated by our simulations (Fig. 1A). On the other hand, nanoplastics studied by experiments are mostly larger than 50 nm in diameter, which is much larger than those considered in this study. Our simulations suggest that decreasing the nanoplastic size can effectively reduce the stability through decreasing the crosslinking degree of hemi-flexible polymers. We thus expect future experimental research using much smaller nanoplastics to probe nanoplastic behaviors in biological systems. To understand how size affects nanoplastic translocation through the membrane, an external force was exerted on the center of each nanoplastic initially suspended above the membrane. As expected, all nanoplastics accomplish translocation in a short period. The energy of interactions between nanoplastics and lipids was calculated (Fig. 4B). Combining typical snapshots (Fig. 4C), it can be found that nanoplastics of higher hydrophobicity, such as PS, PP and PE,58 adsorb more lipids to retard their translocation. In contrast, PET and PVC nanoplastics are less hydrophobic, thus adsorbing fewer lipids to facilitate translocation.
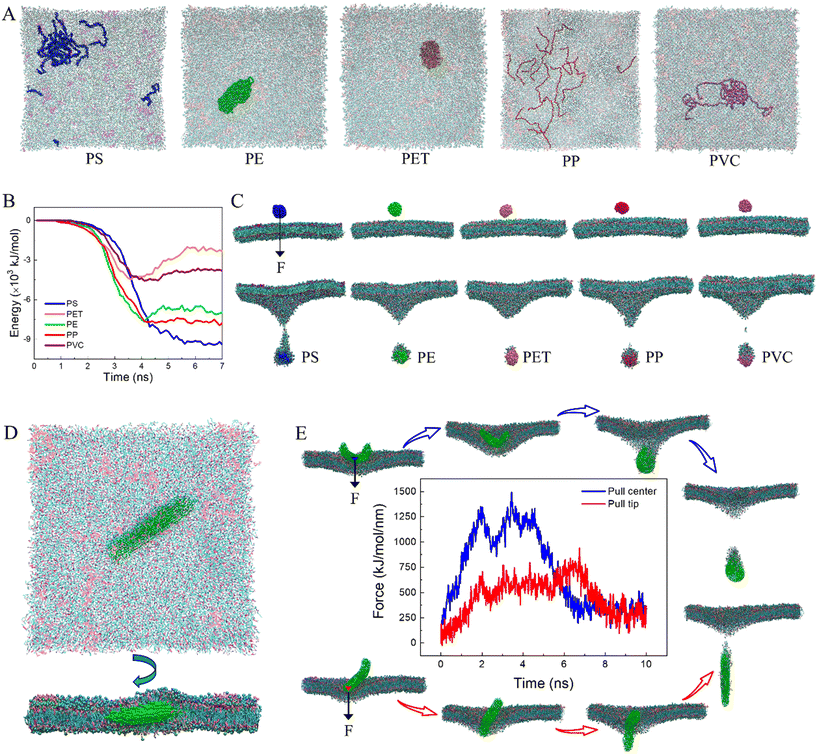 |
| Fig. 4 Effects of size and shape on nanoplastic translocation through the membrane. (A) Top views of final snapshots of cell membrane interactions with smaller nanoplastics (5 nm) of different polymer types. (B) Time evolutions of the interaction energy between the membrane and different nanoplastics during their translocation across the membrane. (C) Initial and final snapshots of nanoplastic translocation through the membrane. (D) Top and cross-sectional views of cell membrane interaction with a fiber-shaped PE nanoplastic. (E) Comparison of fiber-shaped nanoplastic translocation through the membrane in center and tip first modes. | |
Aging micro(nano)plastics usually have irregular shapes, and shape is regarded as a crucial property influencing membrane response to conventional nanomaterials.70–72 Considering that micro(nano)plastics in the form of fibers were often detected in the environment, especially indoor air,73 a fiber-shaped nanoplastic was prepared to elucidate the effect of geometry on nanoplastic interactions with the cell membrane. Similar to spherical nanoplastics, the fiber-shaped nanoplastic rapidly pierces the membrane (Fig. S7†). Slight membrane perturbation is induced at an early stage (t = 20 ns, Fig. S7†), but it rapidly recovers as the fiber-shaped nanoplastic enters the membrane and keeps lying to acquire more contacts with hydrophobic lipid tails (Fig. 4D). To explore how a fiber-shaped nanoplastic accomplishes cell uptake, an external force was exerted respectively on the fiber tip and the center. Comparing time evolutions of the resistant force and typical snapshots (Fig. 4E and S8A and B†), the fiber-shaped nanoplastic tends to enter the cell from the tip first, similar to other anisotropic nanoparticles, such as carbon nanotubes and graphene nanosheets.37,74 The higher force barrier for center mode translocation is ascribed to the larger initial contact area with the membrane (Fig. S8C†), and the fiber-shaped nanoplastic is forced to bend during translocation (Fig. S8B and D†).
Natural weathering can add functional groups onto nanoplastics to introduce charges, as has been experimentally measured using Fourier-transform infrared spectroscopy and scanning electron microscopy with energy-dispersive X-ray analysis.75 To understand how the surface charge influences cell membrane response to nanoplastics, the termini of polymers at the PE nanoplastic surface were decorated with cationic and anionic groups. Results show distinct modes of cell membrane interactions with charged nanoplastics compared to neutral ones. Introduction of charge reduces nanoplastic hydrophobicity, thus decreasing the strength of nanoplastic–cell membrane interactions. In contrast with neutral nanoplastics extracting numerous lipids to form monolayered coronas (Fig. 1B), fewer lipids are extracted by charged nanoplastics, and severe membrane deformation is induced (Fig. 5A and B and Video S6†), as characterized by the lipid density distributions from the cross-sectional view (Fig. 5C and S9A–C†). We also calculated the surface charge density and observed apparent charge redistribution on the nanoplastic surface after membrane interactions (Fig. 5D and S9B–D†). Upon contact with the membrane, charged groups at the bottom of the nanoplastic surface are repelled to expose its hydrophobic interior, acquiring favorable contacts with lipid tails (Fig. 5G and H). Shortly, nanoplastics are transformed to a flower shape with most charged groups rearranged and pointing outward the membrane, as quantified by increases of the nanoplastic gyration radius (Fig. 5I) and decreases of the average angle between vectors connecting the nanoplastic center with surface charges and the membrane normal direction (Fig. 5J). To further decrease the interaction energy (Fig. 5K), lipids are gradually extracted, and the membrane is deformed to wrap onto nanoplastics. As such, charged groups are somewhat pulled back, causing a slight increase of the average angle (Fig. 5J). Comparing the time evolutions of the gyration radius, average angle and interaction energy, the strength of cell membrane interactions is higher for cationic nanoplastics because over 40% of lipids in the membrane are negatively charged and more attractive to the cationic nanoplastic surface (Fig. 5E and S10†). For PP nanoplastics, which dissolve inside the membrane (Fig. 1D), introduction of charge reduces the nanoplastic hydrophobicity, decreases the strength of cell membrane interactions, and thus retards the nanoplastic dissolution (Fig. S11†). Again, cationic nanoplastics show higher extents of cell membrane interactions, supporting previous experiments explaining the higher capacity of cell entry and cytotoxicity for cationic nanoplastics.68,76
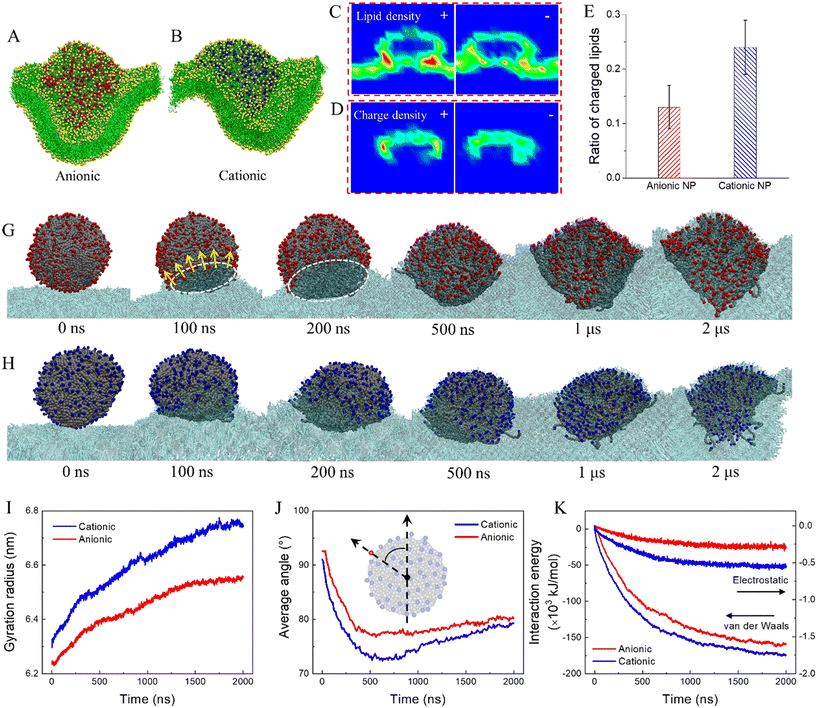 |
| Fig. 5 Roles of the surface charge on nanoplastic interactions with cell membrane. (A and B) Side views of final snapshots comparing cell membrane interactions with anionic and cationic nanoplastics. (C and D) Final distributions of lipid density (C) and nanoplastic surface charge density (D) from the cross-sectional view. (E) Ratios of charged lipids in contact with cationic and anionic nanoplastics. (G and H) Time sequences of typical snapshots depicting cell membrane interactions with anionic (G) and cationic (H) nanoplastics. Lipids are set semi-transparent for viewing the shape transformation of nanoplastics. (I–K) Time evolutions of the gyration radius (I), average angle (J), and nanoplastic–membrane interaction energy (K) during charged nanoplastic interactions with the membrane. The inset of (J) defines the angle between a vector connecting the nanoplastic center with a surface charge group and the membrane normal direction. | |
4 Discussion
The cellular processes of nanoplastics start from the membrane surface, and the nature of nanoplastic–cell membrane interactions determines whether and how nanoplastics enter cells, induce membrane damage, and are transformed to alter their fate inside/outside the cell. The cell uptake and toxicity of nanoplastics have been evidenced by previous experiments albeit using PS nanospheres as an idealized model.77,78 Of note, environmental nanoplastics are diverse in polymer type and aging properties,15 the effect of which on the nanoplastic–cell membrane interface, however, has never been explored. When experiments encounter difficulties due to technical challenges involved in collection or synthesis of nanoplastics of desired materials and properties, tailored computer simulation is advantageous in revealing mechanisms underlying cell membrane responses to various nanoplastics. Our simulations reveal distinctive interfacial behaviors of nanoplastics and demonstrate from the molecular level that processes of membrane translocation, nanoplastic transformation and membrane perturbation can be dictated by the polymer type and aging properties (Fig. 6). All these data can be integrated to guide assessment of nanoplastic bioavailability, biodistribution, biotransformation and clearance.
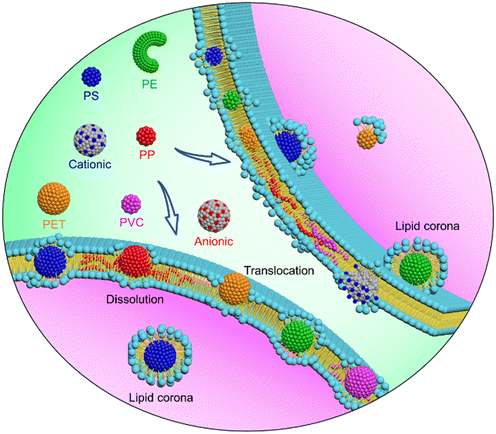 |
| Fig. 6 Schematics of distinctive cell membrane interactions with nanoplastics of different polymer types and aging properties. | |
First, both capacity and pathway of nanoplastic cell entry are strongly influenced by the polymer type and aging property. Among considered materials, PET nanoplastics are less hydrophobic58 and penetrate through the membrane with less energy required (Fig. 2). It likely explains why PET nanoplastics were more often detected in human blood and animal organs,34 indicating that inhaled or ingested PET nanoplastics can readily cross alveolar or gut barriers to distribute wider and deeper in biological tissues. In contrast, PS and PE nanoplastics of higher hydrophobicity adsorb more lipids in the membrane to retard their translocation, and endocytosis could be an alternative pathway, as proved by earlier experiments.79 Distinct from other materials, PP and PVC nanoplastics dissolve in the membrane to increase the bioavailability of both polymers and toxic additives, and their translocation is strongly restrained. As a potential consequence, dissolved nanoplastics are hard to clear and are trapped in the membrane for a long time. Moreover, it would become difficult to separate and detect dissolved nanoplastics using traditional particle-based measuring methods,11 thus underestimating their abundance and potential toxicity. Decreasing nanoplastic size could aggravate this effect because smaller nanoplastics are less stable and easier to dissolve in the membrane (Fig. 4A). Nonspherical nanoplastics, such as nanofibers and nanosheets, usually undergo self-rotation to reduce the barrier of membrane translocation from the sharp edge or corner first (Fig. 4E), similar to some anisotropic nanomaterials like carbon nanotubes and graphene.37,41,74 Surface charge, which can be introduced by photoaging,80 alters nanoplastic cell entry through modulating the competition of polymer–polymer and polymer–lipid interactions at the interface. Distinct from traditional nanomaterials, on which charged groups are relatively immobilized,77,81 charged groups on polymer-based nanoplastics can be rearranged to promote cell membrane wrapping on nanoplastics (Fig. 5). Previous experiments demonstrated distinct accumulation of differentially charged nanoplastics in plant tissues,19 and the cellular processes of nanoplastics were also influenced by the surface charge.68,77 Our simulations reveal that cationic nanoplastics attract more lipids to enhance uptake.
Second, nanoplastics undergo complex and dynamic transformations during transport in biological tissues.45,82,83 In particular, on the mammalian cell membrane surface, distinctive transformations for nanoplastics of different polymer types and aging properties are predicted by our simulations. Different from PP and PVC nanoplastics dissolved in the membrane to increase the bioavailability of both polymers and toxic additives, PS and PE nanoplastics of relatively high hydrophobicity adsorb numerous lipids to form lipid coronas, causing an approximately 3 nm increase of the nanoplastic's effective size (Fig. 3). Further analysis uncovers a monolayered structural feature of the lipid corona, in which lipid headgroups preferentially locate at the outmost layer to decrease nanoplastic surface hydrophobicity and endow nanoplastics with a negative surface charge. As the lipid composition of the corona formed on nanoplastics is close to that of the bulk membrane (Fig. 3G), the density of phosphate groups on nanoplastics is obviously increased to enhance their biorecognition.84 Changes in these properties are believed to alter the subsequent fate of nanoplastics inside/outside the cell.85
Third, membrane damage has been experimentally observed as one cause of cytotoxicity induced by micro(nano)plastics.86 Different from microplastics previously found to adhere on the cell membrane to destabilize it via mechanical stretching,87 much smaller nanoplastics can translocate deeper in or be wrapped by the membrane (Fig. 2), where the ultrastructure, fluidity and mechanical properties are perturbed to a higher extent. Our simulations suggest distinct modes and degrees of membrane perturbation by nanoplastics depending on the polymer type and aging properties (Fig. S6 and S7†). PS and PE nanoplastics can immerge in the membrane to extract numerous lipids on their surfaces, and lipids could be depleted to increase membrane permeability as experimentally measured.77 In contrast, membrane perturbation by PET nanoplastics was mild because of PET's less hydrophobicity. PP and PVC nanoplastics dissolve in the membrane to release toxic additives, which could pose more significant threats to organisms than nanoplastics themselves. Moreover, dissolved nanoplastics diffuse much slower than lipids (Table S2†) and strikingly reduce the membrane fluidity to suppress membrane function. To some extent, decreasing the nanoplastic size could alleviate ultrastructural perturbation but promote nanoplastic dissolution to trap nanoplastics in the membrane with prolonged perturbation. In contrast with anionic nanoplastics, cationic nanoplastics cause more severe membrane perturbation due to favorable electrostatic interactions with the membrane containing negatively charged lipids, in accordance with experimental results.68,76
Although our simulations provide molecular-level insights into the nanoplastic's fate and toxicity to mammalian cells, it is worth putting a caveat on these results. Firstly, membrane complexity has been improved by incorporating 63 different lipid species asymmetrically distributed across two leaflets (Fig. S1†), but it is still far from the complexity of a real cell membrane, which contains diverse proteins apart from lipids.88 Earlier experiments and simulations have identified some functional proteins, such as G protein-coupled receptors,89 ion channels,90 and cytochrome c,91 acting as potential targets for nanoparticle binding to cell membranes. Currently, it still remains challenging to simulate nanoplastic interactions with cell membranes of real structural heterogeneity. Secondly, nanoplastics of high surface energy are believed to acquire coatings of other biomolecules before reaching the target cell membrane surface.42,92 This adsorption layer is thought to conceal the original nanoplastic surface properties, thus altering the nature of subsequent nanoplastic–cell membrane interactions. Importantly, the competition of interactions between different components should exist at the nano–bio interface. Under strong interactions of certain nanoplastics with lipids, some pre-adsorbed molecules could be repelled or exchanged to expose the original nanoplastic surface for cell membrane recognition, as has been observed for engineered nanoparticles.43,93,94 Otherwise, this adsorption layer could sterically hinder direct contact between nanoplastics and the cell membrane.95 Third, earlier experiments evidenced lipid peroxidation and membrane damage induced by nanoplastics through generation of reactive oxygen species,96 different from structural and mechanical perturbations revealed by our simulations. To reveal these chemical mechanisms requires combination of conventional molecular mechanics with quantum mechanics.
5 Conclusions
Increasing plastic debris in the environment eventually breaks down into countless nanoplastics of diverse materials and aging properties, which have become a pervasive threat to the ecoenvironment and human health. However, research on the biological effects of nanoplastics is in its infancy, and the molecular mechanisms are largely unknown. By means of MD simulation, we have prepared a family of nanoplastics of five common materials and three aging properties to systematically investigate their interactions with a mammalian cell membrane. Different from most previous studies using PS nanospheres as the representative model, our results demonstrate distinctive cell membrane interactions with nanoplastics depending on the polymer type and aging properties. PS and PE nanoplastics of relatively high hydrophobicity are spontaneously immersed in the membrane, where lipids are extracted and form monolayered coronas, increasing the nanoplastic's effective size, reducing its hydrophobicity, and endowing nanoplastics with negative surface charges. In contrast, PET nanoplastics are less hydrophobic and penetrate the membrane with less energy required. PP and PVC nanoplastics dissolve to release polymers and toxic additives in the membrane, and their translocation is retarded. Among aging properties, decreasing size promotes nanoplastic dissolution, and fiber-shaped nanoplastics easily translocate from the tip first. Charged groups on nanoplastics can be rearranged to alter nanoplastic translocation and lipid rearrangement. Membrane damage induced by nanoplastics is reflected in reduced fluidity, bending rigidity and compressibility, meaning that the membrane becomes less stable and apt to collapse under stress. To our knowledge, this is the first molecular-level study of mammalian cell membrane interactions with various nanoplastics, and our results evidence the importance of polymer type and aging properties in future assessment of nanoplastics' biological effects.
Author contributions
Lingzhi Li: investigation, methodology, writing – original draft. Shixin Li: methodology, writing – review & editing. Yan Xu: methodology. Luyao Ren: writing – review & editing. Lin Yang: writing – review & editing. Xia Liu: writing – review & editing. Yanhui Dai: writing – review & editing. Jian Zhao: supervision, writing – review & editing. Tongtao Yue: conceptualization, writing – review & editing, supervision.
Conflicts of interest
There are no conflicts of interest to declare.
Acknowledgements
This work was supported by grants from the National Natural Science Foundation of China (grant numbers: 31871012, 32000922) and the Natural Science Foundation of Shandong Province (ZR2021YQ23).
References
-
P. Europe, Plastics–the facts 2020, PlasticEurope, 2020, vol. 1, pp. 1–64 Search PubMed.
- S. B. Borrelle, J. Ringma, K. L. Law, C. C. Monnahan, L. Lebreton, A. McGivern, E. Murphy, J. Jambeck, G. H. Leonard and M. A. Hilleary, Predicted growth in plastic waste exceeds efforts to mitigate plastic pollution, Science, 2020, 369, 1515–1518 CrossRef CAS PubMed.
- K. L. Law, N. Starr, T. R. Siegler, J. R. Jambeck, N. J. Mallos and G. H. Leonard, The United States' contribution of plastic waste to land and ocean, Sci. Adv., 2020, 6, eabd0288 CrossRef PubMed.
- X.-F. Wei, M. Bohlén, C. Lindblad, M. Hedenqvist and A. Hakonen, Microplastics generated from a biodegradable plastic in freshwater and seawater, Water Res., 2021, 198, 117123 CrossRef CAS PubMed.
- D. Li, Y. Shi, L. Yang, L. Xiao, D. K. Kehoe, Y. K. Gun'ko, J. J. Boland and J. J. Wang, Microplastic release from the degradation of polypropylene feeding bottles during infant formula preparation, Nat. Food, 2020, 1, 746–754 CrossRef CAS.
- L. M. Hernandez, E. G. Xu, H. C. Larsson, R. Tahara, V. B. Maisuria and N. Tufenkji, Plastic teabags release billions of microparticles and nanoparticles into tea, Environ. Sci. Technol., 2019, 53, 12300–12310 CrossRef CAS PubMed.
- L. Li, X. Zhao, Z. Li and K. Song, COVID-19: Performance study of microplastic inhalation risk posed by wearing masks, J. Hazard. Mater., 2021, 411, 124955 CrossRef CAS PubMed.
- F. Dang, Q. Wang, Y. Huang, Y. Wang and B. Xing, Key knowledge gaps for One Health approach to mitigate nanoplastic risks, Eco-Environment & Health, 2022, 1, 11–22 Search PubMed.
- C. Wang, J. Zhao and B. Xing, Environmental source, fate, and toxicity of microplastics, J. Hazard. Mater., 2021, 407, 124357 CrossRef CAS.
- A. D. Vethaak and J. Legler, Microplastics and human health, Science, 2021, 371, 672–674 CrossRef CAS.
- N. P. Ivleva, Chemical analysis of microplastics and nanoplastics: Challenges, advanced methods, and perspectives, Chem. Rev., 2021, 121, 11886–11936 CrossRef CAS PubMed.
- H. Cai, E. G. Xu, F. Du, R. Li, J. Liu and H. Shi, Analysis of environmental nanoplastics: Progress and challenges, Chem. Eng. J., 2021, 410, 128208 CrossRef CAS.
- C. Li, Y. Gao, S. He, H.-Y. Chi, Z.-C. Li, X.-X. Zhou and B. Yan, Quantification of nanoplastic uptake in cucumber plants by pyrolysis gas chromatography/mass spectrometry, Environ. Sci. Technol. Lett., 2021, 8, 633–638 CrossRef CAS.
- F. A. Monikh, M. G. Vijver, D. M. Mitrano, H. A. Leslie, Z. Guo, P. Zhang, I. Lynch, E. Valsami-Jones and W. J. Peijnenburg, The analytical quest for sub-micron plastics in biological matrices, Nano Today, 2021, 41, 101296 CrossRef.
- J. Gigault, H. El Hadri, B. Nguyen, B. Grassl, L. Rowenczyk, N. Tufenkji, S. Feng and M. Wiesner, Nanoplastics are neither microplastics nor engineered nanoparticles, Nat. Nanotechnol., 2021, 16, 501–507 CrossRef CAS PubMed.
- R. Lehner, C. Weder, A. Petri-Fink and B. Rothen-Rutishauser, Emergence of nanoplastic in the environment and possible impact on human health, Environ. Sci. Technol., 2019, 53, 1748–1765 CrossRef CAS PubMed.
- D. Magrì, P. Sanchez-Moreno, G. Caputo, F. Gatto, M. Veronesi, G. Bardi, T. Catelani, D. Guarnieri, A. Athanassiou and P. P. Pompa, Laser ablation as a versatile tool to mimic polyethylene terephthalate nanoplastic pollutants: characterization and toxicology assessment, ACS Nano, 2018, 12, 7690–7700 CrossRef PubMed.
- C. Q. Y. Yong, S. Valiyaveettil and B. L. Tang, Toxicity of microplastics and nanoplastics in mammalian systems, Int. J. Environ. Res. Public Health, 2020, 17, 1509 CrossRef CAS.
- X.-D. Sun, X.-Z. Yuan, Y. Jia, L.-J. Feng, F.-P. Zhu, S.-S. Dong, J. Liu, X. Kong, H. Tian and J.-L. Duan, Differentially charged nanoplastics demonstrate distinct accumulation in Arabidopsis thaliana, Nat. Nanotechnol., 2020, 15, 755–760 CrossRef CAS.
- Q. Shi, J. Tang, X. Liu and R. Liu, Ultraviolet-induced photodegradation elevated the toxicity of polystyrene nanoplastics on human lung epithelial A549 cells, Environ. Sci.: Nano, 2021, 8, 2660–2675 RSC.
- Z. Li, C. Feng, W. Pang, C. Tian and Y. Zhao, Nanoplastic-induced genotoxicity and intestinal damage in freshwater benthic clams (Corbicula fluminea): comparison with microplastics, ACS Nano, 2021, 15, 9469–9481 CrossRef.
- Y. Ji, Y. Wang, D. Shen, Q. Kang and L. Chen, Mucin corona delays intracellular trafficking and alleviates cytotoxicity of nanoplastic-benzopyrene combined contaminant, J. Hazard. Mater., 2021, 406, 124306 CrossRef CAS.
- S. Xu, C. Wu, W.-B. Guo, L. Yang, R. Ji, K. Pan and A.-J. Miao, Polystyrene Nanoplastics Inhibit the Transformation of Tetrabromobisphenol A by the Bacterium Rhodococcus jostii, ACS Nano, 2022, 16, 405–414 CrossRef CAS PubMed.
- Y. Liu, Y. Wang, X. Ling, Z. Yan, D. Wu, J. Liu and G. Lu, Effects of nanoplastics and butyl methoxydibenzoylmethane on early zebrafish embryos identified by single-cell RNA sequencing, Environ. Sci. Technol., 2021, 55, 1885–1896 CrossRef CAS.
- G. M. Zarus, C. Muianga, C. M. Hunter and R. S. Pappas, A review of data for quantifying human exposures to micro and nanoplastics and potential health risks, Sci. Total Environ., 2021, 756, 144010 CrossRef CAS PubMed.
- M. S.-L. Yee, L.-W. Hii, C. K. Looi, W.-M. Lim, S.-F. Wong, Y.-Y. Kok, B.-K. Tan, C.-Y. Wong and C.-O. Leong, Impact of microplastics and nanoplastics on human health, Nanomaterials, 2021, 11, 496 CrossRef CAS PubMed.
- J. Zhang, L. Wang and K. Kannan, Microplastics in house dust from 12 countries and associated human exposure, Environ. Int., 2020, 134, 105314 CrossRef CAS PubMed.
- K. D. Cox, G. A. Covernton, H. L. Davies, J. F. Dower, F. Juanes and S. E. Dudas, Human consumption of microplastics, Environ. Sci. Technol., 2019, 53, 7068–7074 CrossRef CAS.
- J. C. Prata, Airborne microplastics: consequences to human health?, Environ. Pollut., 2018, 234, 115–126 CrossRef CAS.
- H. Tan, T. Yue, Y. Xu, J. Zhao and B. Xing, Microplastics reduce lipid digestion in simulated human gastrointestinal system, Environ. Sci. Technol., 2020, 54, 12285–12294 CrossRef CAS.
- M. Cole and T. S. Galloway, Ingestion of nanoplastics and microplastics by Pacific oyster larvae, Environ. Sci. Technol., 2015, 49, 14625–14632 CrossRef CAS PubMed.
- A. Wegner, E. Besseling, E. M. Foekema, P. Kamermans and A. A. Koelmans, Effects of nanopolystyrene on the feeding behavior of the blue mussel (Mytilus edulis L.), Environ. Toxicol. Chem., 2012, 31, 2490–2497 CrossRef CAS.
- X. Liu, Y. Zhao, J. Dou, Q. Hou, J. Cheng and X. Jiang, Bioeffects of Inhaled Nanoplastics on Neurons and Alteration of Animal Behaviors through Deposition in the Brain, Nano Lett., 2022, 22, 1091–1099 CrossRef CAS.
- H. A. Leslie, M. J. van Velzen, S. H. Brandsma, D. Vethaak, J. J. Garcia-Vallejo and M. H. Lamoree, Discovery and quantification of plastic particle pollution in human blood, Environ. Int., 2022, 163, 107199 CrossRef CAS PubMed.
- M. C. Guerrera, M. Aragona, C. Porcino, F. Fazio, R. Laurà, M. Levanti, G. Montalbano, G. Germanà, F. Abbate and A. Germanà, Micro and nano plastics distribution in fish as model organisms: histopathology, blood response and bioaccumulation in different organs, Appl. Sci., 2021, 11, 5768 CrossRef CAS.
- Y. Wei, H. Chen, Y.-X. Li, K. He, K. Yang and H.-B. Pang, Synergistic Entry of Individual Nanoparticles into Mammalian Cells Driven by Free Energy Decline and Regulated by Their Sizes, ACS Nano, 2022, 16, 5885–5897 CrossRef PubMed.
- X. Shi, A. von Dem Bussche, R. H. Hurt, A. B. Kane and H. Gao, Cell entry of one-dimensional nanomaterials occurs by tip recognition and rotation, Nat. Nanotechnol., 2011, 6, 714–719 CrossRef CAS PubMed.
- K. Yang and Y.-Q. Ma, Computer simulation of the translocation of nanoparticles with different shapes across a lipid bilayer, Nat. Nanotechnol., 2010, 5, 579–583 CrossRef CAS.
- Y. Tu, M. Lv, P. Xiu, T. Huynh, M. Zhang, M. Castelli, Z. Liu, Q. Huang, C. Fan and H. Fang, Destructive extraction of phospholipids from Escherichia coli membranes by graphene nanosheets, Nat. Nanotechnol., 2013, 8, 594 CrossRef CAS PubMed.
- C. A. Lochbaum, A. K. Chew, X. Zhang, V. Rotello, R. C. Van Lehn and J. A. Pedersen, Lipophilicity of Cationic Ligands Promotes Irreversible Adsorption of Nanoparticles to Lipid Bilayers, ACS Nano, 2021, 15, 6562–6572 CrossRef CAS.
- J. Mao, P. Chen, J. Liang, R. Guo and L.-T. Yan, Receptor-mediated endocytosis of two-dimensional nanomaterials undergoes flat vesiculation and occurs by revolution and self-rotation, ACS Nano, 2016, 10, 1493–1502 CrossRef CAS.
- E. S. Melby, S. E. Lohse, J. E. Park, A. M. Vartanian, R. A. Putans, H. B. Abbott, R. J. Hamers, C. J. Murphy and J. A. Pedersen, Cascading effects of nanoparticle coatings: Surface functionalization dictates the assemblage of complexed proteins and subsequent interaction with model cell membranes, ACS Nano, 2017, 11, 5489–5499 CrossRef CAS.
- X. Wang, X. Wang, X. Bai, L. Yan, T. Liu, M. Wang, Y. Song, G. Hu, Z. Gu and Q. Miao, Nanoparticle Ligand Exchange and Its Effects at the Nanoparticle–Cell Membrane Interface, Nano Lett., 2018, 19, 8–18 CrossRef.
- V. Cagno, P. Andreozzi, M. D'Alicarnasso, P. Jacob Silva, M. Mueller, M. Galloux, R. Le Goffic, S. T. Jones, M. Vallino and J. Hodek, Broad-spectrum non-toxic antiviral nanoparticles with a virucidal inhibition mechanism, Nat. Mater., 2018, 17, 195–203 CrossRef CAS.
- M. Cao, R. Cai, L. Zhao, M. Guo, L. Wang, Y. Wang, L. Zhang, X. Wang, H. Yao and C. Xie, Molybdenum derived from nanomaterials incorporates into molybdenum enzymes and affects their activities in vivo, Nat. Nanotechnol., 2021, 16, 708–716 CrossRef CAS.
- M. Lee, N. Ni, H. Tang, Y. Li, W. Wei, A. Kakinen, X. Wan, T. P. Davis, Y. Song and D. T. Leong, A Framework of Paracellular Transport via Nanoparticles-Induced Endothelial Leakiness, Adv. Sci., 2021, 8, 2102519 CrossRef CAS.
- Y. Xie, Y. Li, Y. Feng, W. Cheng and Y. Wang, Inhalable microplastics prevails in air: Exploring the size detection limit, Environ. Int., 2022, 162, 107151 CrossRef CAS PubMed.
- M. Trainic, J. M. Flores, I. Pinkas, M. L. Pedrotti, F. Lombard, G. Bourdin, G. Gorsky, E. Boss, Y. Rudich and A. Vardi, Airborne microplastic particles detected in the remote marine atmosphere, Commun. Earth Environ., 2020, 1, 64 CrossRef.
- H. Rakhshani, E. Dehghanian and A. Rahati, Enhanced GROMACS: toward a better numerical simulation framework, J. Mol. Model., 2019, 25, 1–8 CrossRef PubMed.
- S. J. Marrink, H. J. Risselada, S. Yefimov, D. P. Tieleman and A. H. De Vries, The MARTINI force field: coarse grained model for biomolecular simulations, J. Phys. Chem. B, 2007, 111, 7812–7824 CrossRef CAS PubMed.
- G. Rossi, L. Monticelli, S. R. Puisto, I. Vattulainen and T. Ala-Nissila, Coarse-graining polymers with the MARTINI force-field: polystyrene as a benchmark case, Soft Matter, 2011, 7, 698–708 RSC.
- E. Panizon, D. Bochicchio, L. Monticelli and G. Rossi, MARTINI coarse-grained models of polyethylene and polypropylene, J. Phys. Chem. B, 2015, 119, 8209–8216 CrossRef CAS PubMed.
- L. Li, Y. Xu, S. Li, X. Zhang, H. Feng, Y. Dai, J. Zhao and T. Yue, Molecular modeling of nanoplastic transformations in alveolar fluid and impacts on the lung surfactant film, J. Hazard. Mater., 2022, 427, 127872 CrossRef CAS.
- H. Feng, Y. Liu, Y. Xu, S. Li, X. Liu, Y. Dai, J. Zhao and T. Yue, Benzo [a] pyrene and heavy metal ion adsorption on nanoplastics regulated by humic acid: cooperation/competition mechanisms revealed by molecular dynamics simulations, J. Hazard. Mater., 2022, 424, 127431 CrossRef CAS PubMed.
- H. I. Ingólfsson, M. N. Melo, F. J. Van Eerden, C. Arnarez, C. A. Lopez, T. A. Wassenaar, X. Periole, A. H. De Vries, D. P. Tieleman and S. J. Marrink, Lipid organization of the plasma membrane, J. Am. Chem. Soc., 2014, 136, 14554–14559 CrossRef.
- W. Humphrey, A. Dalke and K. Schulten, VMD: visual molecular dynamics, J. Mol. Graphics, 1996, 14, 33–38 CrossRef PubMed.
- T. Yue, R. Lv, D. Xu, Y. Xu, L. Liu, Y. Dai, J. Zhao and B. Xing, Competitive and/or cooperative interactions of graphene-family materials and benzo [a] pyrene with pulmonary surfactant: a computational and experimental study, Part. Fibre Toxicol., 2021, 18, 46 CrossRef CAS.
- K. Min, J. D. Cuiffi and R. T. Mathers, Ranking environmental degradation trends of plastic marine debris based on physical properties and molecular structure, Nat. Commun., 2020, 11, 727 CrossRef CAS.
- J. Lin, L. Miao, G. Zhong, C.-H. Lin, R. Dargazangy and A. Alexander-Katz, Understanding the synergistic effect of physicochemical properties of nanoparticles and their cellular entry pathways, Commun. Biol., 2020, 3, 1–10 CrossRef.
- W. Wang, R. Yang, F. Zhang, B. Yuan, K. Yang and Y.-Q. Ma, Partner-facilitating transmembrane penetration of nanoparticles: a biological test in silico, Nanoscale, 2018, 10, 11670–11678 RSC.
- Y. Liu, S. Li, X. Liu, H. Sun, T. Yue, X. Zhang, B. Yan and D. Cao, Design of small nanoparticles decorated with amphiphilic ligands: self-preservation effect and translocation into a plasma membrane, ACS Appl. Mater. Interfaces, 2019, 11, 23822–23831 CrossRef CAS PubMed.
- D. A. Schafer, Coupling actin dynamics and membrane dynamics during endocytosis, Curr. Opin. Cell Biol., 2002, 14, 76–81 CrossRef CAS PubMed.
- R. L. Pinals, D. Yang, D. J. Rosenberg, T. Chaudhary, A. R. Crothers, A. T. Iavarone, M. Hammel and M. P. Landry, Quantitative protein corona composition and dynamics on carbon nanotubes in biological environments, Angew. Chem., Int. Ed., 2020, 59, 23668–23677 CrossRef CAS.
- X. Lu, P. Xu, H.-M. Ding, Y.-S. Yu, D. Huo and Y.-Q. Ma, Tailoring the component of protein corona via simple chemistry, Nat. Commun., 2019, 10, 4520 CrossRef.
- M. Ovais, S. K. Nethi, S. Ullah, I. Ahmad, S. Mukherjee and C. Chen, Recent advances in the analysis of nanoparticle-protein coronas, Nanomedicine, 2020, 15, 1037–1061 CrossRef CAS PubMed.
- L. L. Olenick, J. M. Troiano, A. Vartanian, E. S. Melby, A. C. Mensch, L. Zhang, J. Hong, O. Mesele, T. Qiu and J. Bozich, Lipid corona formation from nanoparticle interactions with bilayers, Chem, 2018, 4, 2709–2723 CAS.
- A. Z. Samuel, B.-H. Lai, S.-T. Lan, M. Ando, C.-L. Wang and H.-o. Hamaguchi, Estimating percent crystallinity of polyethylene as a function of temperature by Raman spectroscopy multivariate curve resolution by alternating least squares, Anal. Chem., 2017, 89, 3043–3050 CrossRef CAS PubMed.
- J. Zhu, J. Wang, R. Chen, Q. Feng and X. Zhan, Cellular Process of Polystyrene Nanoparticles Entry into Wheat Roots, Environ. Sci. Technol., 2022, 56, 6436–6444 CrossRef CAS PubMed.
- Y.-X. Zhang, M. Wang, L. Yang, K. Pan and A.-J. Miao, Bioaccumulation of differently-sized polystyrene nanoplastics by human lung and intestine cells, J. Hazard. Mater., 2022, 439, 129585 CrossRef CAS PubMed.
- Z. Luo, S. Li, Y. Xu, Z. Yan, F. Huang and T. Yue, The role of nanoparticle shape in translocation across the pulmonary surfactant layer revealed by molecular dynamics simulations, Environ. Sci.: Nano, 2018, 5, 1921–1932 RSC.
- Y. Li, T. Yue, K. Yang and X. Zhang, Molecular modeling of the relationship between nanoparticle shape anisotropy and endocytosis kinetics, Biomaterials, 2012, 33, 4965–4973 CrossRef CAS.
- P. Choo, T. Liu and T. W. Odom, Nanoparticle shape determines dynamics of targeting nanoconstructs on cell membranes, J. Am. Chem. Soc., 2021, 143, 4550–4555 CrossRef CAS PubMed.
- Q. Zhang, Y. Zhao, F. Du, H. Cai, G. Wang and H. Shi, Microplastic fallout in different indoor environments, Environ. Sci. Technol., 2020, 54, 6530–6539 CrossRef CAS PubMed.
- Y. Li, H. Yuan, A. Von Dem Bussche, M. Creighton, R. H. Hurt, A. B. Kane and H. Gao, Graphene microsheets enter cells through spontaneous membrane penetration at edge asperities and corner sites, Proc. Natl. Acad. Sci. U. S. A., 2013, 110, 12295–12300 CrossRef CAS.
- P. Liu, L. Qian, H. Wang, X. Zhan, K. Lu, C. Gu and S. Gao, New insights into the aging behavior of microplastics accelerated by advanced oxidation processes, Environ. Sci. Technol., 2019, 53, 3579–3588 CrossRef CAS PubMed.
- A. Roshanzadeh, S. Park, S. E. Ganjbakhsh, J. Park, D.-H. Lee, S. Lee and E.-S. Kim, Surface charge-dependent cytotoxicity of plastic nanoparticles in alveolar cells under cyclic stretches, Nano Lett., 2020, 20, 7168–7176 CrossRef CAS.
- S. Dai, R. Ye, J. Huang, B. Wang, Z. Xie, X. Ou, N. Yu, C. Huang, Y. Hua and R. Zhou, Distinct lipid membrane interaction and uptake of differentially charged nanoplastics in bacteria, J. Nanobiotechnol., 2022, 20, 191 CrossRef CAS.
- S. A. Kulkarni and S.-S. Feng, Effects of particle size and surface modification on cellular uptake and biodistribution of polymeric nanoparticles for drug delivery, Pharm. Res., 2013, 30, 2512–2522 CrossRef CAS.
- N. Yan, B. Z. Tang and W.-X. Wang, Cell cycle control of nanoplastics internalization in phytoplankton, ACS Nano, 2021, 15, 12237–12248 CrossRef CAS PubMed.
- Y. Zhang, Y. Luo, X. Yu, D. Huang, X. Guo and L. Zhu, Aging significantly increases the interaction between polystyrene nanoplastic and minerals, Water Res., 2022, 118544 CrossRef CAS PubMed.
- P. Chen, Z. Huang, J. Liang, T. Cui, X. Zhang, B. Miao and L.-T. Yan, Diffusion and directionality of charged nanoparticles on lipid bilayer membrane, ACS Nano, 2016, 10, 11541–11547 CrossRef CAS PubMed.
- K. A. Dawson and Y. Yan, Current understanding of biological identity at the nanoscale and future prospects, Nat. Nanotechnol., 2021, 16, 229–242 CrossRef CAS PubMed.
- J. Ren, N. Andrikopoulos, K. Velonia, H. Tang, R. Cai, F. Ding, P. C. Ke and C. Chen, Chemical and Biophysical Signatures of the Protein Corona in Nanomedicine, J. Am. Chem. Soc., 2022, 144, 9184–92005 CrossRef CAS PubMed.
- M. P. Monopoli, C. Åberg, A. Salvati and K. A. Dawson, Biomolecular coronas provide the biological identity of nanosized materials, Nat. Nanotechnol., 2012, 7, 779–786 CrossRef CAS PubMed.
- A. E. Nel, L. Mädler, D. Velegol, T. Xia, E. M. Hoek, P. Somasundaran, F. Klaessig, V. Castranova and M. Thompson, Understanding biophysicochemical interactions at the nano–bio interface, Nat. Mater., 2009, 8, 543–557 CrossRef CAS PubMed.
- A. Banerjee and W. L. Shelver, Micro-and nanoplastic induced cellular toxicity in mammals: A review, Sci. Total Environ., 2021, 755, 142518 CrossRef CAS PubMed.
- J.-B. Fleury and V. A. Baulin, Microplastics destabilize lipid membranes by mechanical stretching, Proc. Natl. Acad. Sci. U. S. A., 2021, 118, e2104610118 CrossRef CAS PubMed.
- D. M. Engelman, Membranes are more mosaic than fluid, Nature, 2005, 438, 578–580 CrossRef CAS PubMed.
- W. Hild, K. Pollinger, A. Caporale, C. Cabrele, M. Keller, N. Pluym, A. Buschauer, R. Rachel, J. Tessmar and M. Breunig, G protein-coupled receptors function as logic gates for nanoparticle binding and cell uptake, Proc. Natl. Acad. Sci. U. S. A., 2010, 107, 10667–10672 CrossRef CAS PubMed.
- Z. Gu, L. D. Plant, X.-Y. Meng, J. M. Perez-Aguilar, Z. Wang, M. Dong, D. E. Logothetis and R. Zhou, Exploring the nanotoxicology of MoS2: a study on the interaction of MoS2 nanoflakes and K+ channels, ACS Nano, 2018, 12, 705–717 CrossRef CAS PubMed.
- E. J. Tollefson, C. R. Allen, G. Chong, X. Zhang, N. D. Rozanov, A. Bautista, J. J. Cerda, J. A. Pedersen, C. J. Murphy and E. E. Carlson, Preferential binding of cytochrome c to anionic ligand-coated gold nanoparticles: A complementary computational and experimental approach, ACS Nano, 2019, 13, 6856–6866 CrossRef CAS PubMed.
- S. Grafmueller, P. Manser, L. Diener, P.-A. Diener, X. Maeder-Althaus, L. Maurizi, W. Jochum, H. F. Krug, T. Buerki-Thurnherr and U. Von Mandach, Bidirectional transfer study of polystyrene nanoparticles across the placental barrier in an ex vivo human placental perfusion model, Environ. Health Perspect., 2015, 123, 1280–1286 CrossRef CAS PubMed.
- R. Kariuki, R. Penman, S. J. Bryant, R. Orrell-Trigg, N. Meftahi, R. J. Crawford, C. F. McConville, G. Bryant, K. Voïtchovsky and C. E. Conn, Behavior of Citrate-Capped Ultrasmall Gold Nanoparticles on a Supported Lipid Bilayer Interface at Atomic Resolution, ACS Nano, 2022, 16, 17179–17196 CrossRef CAS PubMed.
- F. Liu, S. Li, H. Feng, L. Li, T. Yue and B. Yan, Modulation of cell uptake and cytotoxicity by nanoparticles with various physicochemical properties after humic acid adsorption, Environ. Sci.: Nano, 2021, 8, 3746–3761 RSC.
- S. Li, S. Wang, B. Yan and T. Yue, Surface Properties of Nanoparticles Dictate Their Toxicity by Regulating Adsorption of Humic Acid Molecules, ACS Sustainable Chem. Eng., 2021, 9, 13705–13716 CrossRef CAS.
- M. Hu and D. Palić, Micro-and nano-plastics activation of oxidative and inflammatory adverse outcome pathways, Redox Biol., 2020, 37, 101620 CrossRef CAS PubMed.
Footnote |
† Electronic supplementary information (ESI) available: Component models and system setup, the melting-annealing procedure for construction of nanoplastics, BPA leakage from nanoplastics, fiber-shaped nanoplastics piercing through the membrane, and rearrangement of molecules at the charged nanoplastic–cell membrane interface. See DOI: https://doi.org/10.1039/d2en00800a |
|
This journal is © The Royal Society of Chemistry 2023 |