Environmental evaluation of carbon dot and fullerene formation in atmospheric particulate matter (PM10): observations from their free radical scavenging and electrochemical studies†
Received
20th June 2022
, Accepted 18th November 2022
First published on 22nd November 2022
Abstract
Human exposure to atmospheric particulate matter (PM) is of great interest owing to its carbonaceous nature with potential health impact. Carbonaceous particulate matter (PM) is mainly composed of black carbon and organic carbon. The abundance of carbon in atmospheric PM can also lead to the formation of carbon nanomaterials under different environmental conditions. In this innovative work, we evaluated the formation of water-soluble carbon dots (wsCDs) and onion-like fullerenes (wsOLFs) from atmospheric particulate matter (PM) in an oxidizing environment. The synthesized wsCDs were quasi-spherical in shape with un-agglomerated or uniformly distributed particles with an average size of 4–6 nm. The wsOLFs were spherical and/or quasi-spherical in shape, with corresponding particle size distribution of an average diameter ranging from 8–12 nm. High resolution transmission electron microscopy images also showed concentric spherical shells like onions and fringes of graphitic planes with interplanar distances of 0.232–0.297 nm, which are smaller than that of graphite (0.336 nm). The PM-derived carbon dots and fullerenes were observed to be effective antioxidants from their free radical scavenging studies. Further, promising electrochemical properties are also detected with these materials indicating their potential prospects for development of environmental nanotechnology.
Environmental significance
Particulate matter (PM) is known to be hazardous to human health and lots of studies have been carried out to evaluate its monitoring and assessment. However, there are not any scientific studies attempted on its aspects of mitigation. Therefore, our current research work reports the turning of air pollutants (PM) into water soluble and useful carbon nanomaterials (CNs) under oxidising environmental conditions leading to environmental sustainability and reduction of the environmental risk of PM. The biological activities of the derived CNs, including free radical scavenging activities, are also reported.
|
1. Introduction
Atmospheric particulate matter (PM) consists of carbonaceous fractions including graphitic elemental carbon to large organic carbon (polycyclic aromatic hydrocarbons) which are highly functionalized and hard to identify and remove.1,2 The dark components of atmospheric PM, also called soot particles or black carbon, are mostly generated from different man-made sources and measured as light absorbing particles including organic matter as well as a mixture of graphite particles.3–5 Atmospheric pollution has become a major concern in urban areas, affecting human health and climate on a global scale. Atmospheric particulate matter (PM) with an aerodynamic diameter smaller than 10 μm is one of the major pollutant categories termed as inhalable PM10 due to its small size and inhalability into the human respiratory system. PM is generally categorized into coarse (PM10–2.5), fine (PM2.5), and ultrafine (PM1) particles.6,7 According to the Global Burden of Disease Report, 4.1 million premature deaths were caused worldwide by the exposure to outdoor particles (PM) in 2016.8 These atmospheric particles generally arise from various human activities such as incomplete combustion of fossil fuels, traffic, and industrial emissions which are major activities in urban areas.7,9–12 Previous studies reported that the average 24 h concentration (274.17 ± 78.77 μg m−3) of PM10 in urban areas of northeast India is two times higher than the Indian standard limit (NAAQ, category).7 Particulate matter is directly emitted into the air (primary aerosol) or formed through gas-to-particle conversion (secondary aerosol) in the atmosphere.13 PM greatly impacts weather, climate, human health, visibility, and the ecosystem and these effects are mainly dependent on the concentration, chemical composition, size of the particles, oxidants, and reactions of precursor gases or PM transmission paths in the atmosphere.1,2,6,7
Few attempts have been made to extract or fabricate carbon-based nanomaterials (CNs) from different types of carbonaceous aerosols;3,14–17 however, very limited studies have been carried out to demonstrate the detailed chemical conversion of atmospheric PM10 into carbon dots (CDs) as well as fullerenes.17,18,20–22 In our study, a simple chemical oxidation method is used for the fabrication of water soluble CNs like fullerenes (wsOLFs) and carbon dots (wsCDs) from atmospheric PM10 collected from an urban area. In recent years, CNs such as fullerenes and carbon dots (CDs) have drawn worldwide attention due to their unique physical or optical, mechanical, and thermal properties.18,19 On the basis of purity, these nanomaterials have great market potential in different applications including catalysis, composites, electronics, semiconductors, drug delivery, bio-imaging, bio-sensing, and environmental applications.17,18 Ishiguro et al.20 reported different types of graphitic layers from diesel fuel combustion and correlated the particle size and porosity with the burn off weight of diesel soot particles. Vander Wal et al.21 fabricated various types of graphitic structures with lamellae synthesized from diesel soot particles of two different diesel engines. Tripathi et al.17 synthesized water-soluble CDs from diesel engine exhaust and used these CDs for sensing and bio-imaging applications. CNs were synthesized from different types of carbonaceous precursors; distinctive methods have been established using both the top-down and bottom-up approaches, which are discussed in detail elsewhere.22 In general, CDs can be produced by using hydrothermal synthesis, laser ablation, surfactant extraction, and ultrasound-assisted chemical oxidation methods. Fullerene molecules were synthesized by using laser or heat induced graphite evaporation or chemical synthesis of bottom-up methods from smaller aromatic molecules.23 Soot-based synthesis of CNs is simple and effective. Soot-based CNs produce mixtures of particles with different types of fluorescence emission colours or properties. Ray et al.24 established a soot-based method for the synthesis of fluorescent CDs and observed that the particle sizes ranging from 2–6 nm are useful for fluorescence-based applications such as bio-imaging. However, it is observed that most of the established/reported methods to produce fullerenes and CDs involve high energy, high temperature and the use of toxic solvents. In contrast, our present study provides a simple and environmentally friendly method for direct conversion of PM10 into two types of CNs. The main objectives of the present study are: (1) synthesis of water soluble carbon dot (wsCD) and onion-like fullerene (wsOLF) nanostructures from atmospheric PM (PM10) using simple chemical oxidation methods, (2) identification and characterization of the as-synthesized CNs (wsCDs and wsOLFs) with advanced analytical techniques like high resolution-transmission electron microscopy (HR-TEM), X-ray diffraction (XRD), attenuated total reflection Fourier transform infrared (ATR-FTIR) spectroscopy, X-ray photoelectron spectroscopy (XPS), ultraviolet-visible spectroscopy (UV-visible), fluorescence spectroscopy (FL), and Raman spectroscopy, (3) evaluation of the electrochemical properties of the synthesized CNs as liquid electrolytes for electrochemical energy storage devices, and (4) evaluation of the antibacterial and free radical scavenging properties of the as-synthesized CNs (wsCDs and wsOLFs). The novelty of the present work is the simple chemical synthesis of water soluble CNs (wsCDs and wsOLFs) from atmospheric pollutants. This is the first time that we have evaluated the antioxidant as well as the electrochemical properties of CNs derived from PM, which may provide a new direction of using such waste materials in multifarious applications like bioimaging or biomedical and energy storage devices. Thus, this study demonstrates an innovative path for conversion of air pollutants into high-value carbon nanomaterials promoting waste to wealth and most importantly devising economic green technologies for a cleaner environment.
2. Experimental section
2.1. Sampling of PM10
Sampling of atmospheric particulate matter (PM10) was carried out with the help of a respirable dust sampler (RDS; APM-460 NL, Envirotech, India) on pre-weighed filter paper (QMA filters) around the CSIR-NEIST (Jorhat) campus (26°44′N and 94°9′E).4 Particulate matter sampling was performed on a 24-hour basis every alternate day (12th February to 11th March, 2020). The sampler (PM10) was operated with a flow rate of 1.1 m3 min−1 and the details of the sampling/study area is shown in Fig. S1 (see the ESI†). The average temperature and wind speed were reported to be 24 °C and 1.1 m s−1 with a prevailing direction in northwest (NW). Due to its carbonaceous nature with a total carbon content of 45–90% of mass concentration, PM10 has been used as a source of carbon or precursor for the synthesis of CNs. Previous studies also reported the presence of different nanostructures in atmospheric aerosol or soot particulates.14–17 Following the sampling guidelines as provided by CPCB, India,4 the PM10 deposited filters were stored and kept in desiccators for subsequent experiments for synthesis of CNs as described in the subsequent sections.
2.2. Formation of carbon dots and fullerenes from atmospheric particulate matter (PM10)
2.2.1 Carbon dots.
In our oxidizing experiment, the PM10 samples (approx. 10 g) recovered from the filter paper were mixed with hydrogen peroxide (30%) under ice-cold conditions. The mixture was then ultrasonicated in a microprocessor-based ultrasonicator (Power Sonic-520) at a frequency of 40 kHz for about 4 h at atmospheric temperature and pressure. The resultant sonicated extract was then cooled to room temperature and neutralized with dropwise addition of ammonium solution till the extract became neutral pH. The extract was then filtered through a polytetrafluoroethylene membrane (0.22 μm) followed by ultracentrifugation (model: Thermo Scientific; wX + Ultra Series) to remove the impurities. The supernatant was passed through an ultrafiltration system (SYR2-U10-A; Spectrum; KrosFlo TFF system) and finally the products were concentrated by using a rotary evaporator to obtain the purified final CDs (denoted as wsCDs).
2.2.2 Fullerenes.
For synthesis of fullerenes, a 10 g PM10 sample was subjected to Soxhlet extraction using acetone as a solvent for about 10 h (4 cycles per h). The purified extract (100 ml) was then oxidized with 100 ml HNO3 (1 N) in a beaker and continuously stirred using a magnetic stirrer at 120 °C for 5 h. After the oxidative treatment, the extracted solution was centrifuged (model: Thermo Scientific; wX + Ultra Series) and the supernatant was collected. Then the centrifuged extract was evaporated in a water bath following the addition of water to neutralize the solution. The extract solution was further filtered through the ultrafiltration technique (SYR2-U10-A; Spectrum; KrosFlo TFF system) and finally concentrated by using a rotary evaporator to obtain the final purified water-soluble fullerene product (wsOLFs).
2.3. Advanced-level analytical characterization of CNs
The high performance liquid chromatography (HPLC) technique was performed to determine the concentration of PAHs in the PM samples after the Soxhlet extraction process. For microscopy characterization and examination of the formation of CNs, the HRTEM (High-resolution transmission electron microscope; model: JEOL JEM 2100) technique was employed. The dispersed colloidal solutions of all the synthesized CN samples (wsCDs and wsOLFs) were drop-cast onto a standard copper grid which was coated with carbon and further kept in a desiccator overnight to determine the microstructure of the CNs. The HRTEM analysis was performed at a resolution of 1.9 to 1.4 Å with an accelerating voltage of 60–200 kV. The energy dispersive X-ray spectroscopy (EDS) analysis technique was also used to determine the elemental composition of the CNs. The TEM images and selected area diffraction (SAED) pattern were further processed with the help of “Image J” software (version: 1.47). The dynamic light scattering (DLS; model: Nano ZS, MALVERN, UK) technique was also used to determine the hydrodynamic or colloidal particle size of the synthesized CNs. The X-ray diffraction (XRD) analysis was performed by using an X-ray diffractometer (model: Rigaku, Ultima IV) with a starting angle of 5° and an ending angle of 100°, and the step angle was 0.02° at a scanning rate of 1° min−1 (target Cu-Kα; λ = 1.54056 Å). The peaks were identified with the help of the Rigaku PDXL 1.2.0.1′ library database and the processing of diffractograms was mainly operated using the XG operation RINT 2200′ program associated with the equipment. Attenuated total reflection Fourier transform infrared spectroscopic (ATR-FTIR) analysis of CNs was carried out on an FTIR spectrometer (Spectrum-Two; Perkin Elmer) in the range of 400–4000 cm−1 to determine the functional groups present in the synthesized CN products. Raman spectroscopic analysis of CNs was carried out on a Smart Raman spectrometer (Thermo-Scientific DXR2) using an excitation wavelength of 532 nm. X-ray photoelectron spectroscopic (XPS) analysis was performed on a spectrometer (Thermo-Scientific ESCALAB Xi+) with a monochromatic Al Kα X-ray source (1486.6 eV) and a spherical energy analyzer that operates in the CAE (constant analyzer energy) mode using the mode of electromagnetic lens. The CAE for high-resolution spectra is 50 eV and that for survey spectra is 100 eV. A UV-visible spectrophotometer (model: LABINDIA 1000+) and a fluorescence spectrophotometer (Horiba Fluorlolog-3) were used to evaluate the photo-physical properties of the CNs.
2.4. Fluorescence quantum yield
The fluorescence quantum yield (QY) of the synthesized CNs (wsCDs and wsOLFs) was calculated by using the following equation:19 | Φ = Φr × I/Ir × Ar/A × η/ηr | (1) |
where “Φ” signifies the relative quantum yields of the CNs with respect to the quantum yield of the quinine sulphate (0.1 mg L−1 in 0.1 M H2SO4 solution) standard (Φr). “I” indicates the integrated emission FL intensity of the CNs, “A” stands for absorbance, and the refractive index of the solvent is denoted by “η”.
2.5. Electrochemical characterization of synthesized CNs
The electrochemical performances of the synthesized wsCD and wsOLF samples were determined in an “electrochemical workstation” (VSP3; BioLogic Science). The electrochemical analysis of the synthesized CNs was performed using a three-electrode system. The working electrode was a glassy carbon electrode. As the reference and counter electrodes, an Ag/AgCl electrode and a Pt wire were adopted. All three electrodes were purchased from BioLogic Science. Cyclic voltammetry experiments were performed to study the kinetics of electron transfer in electrolytes (synthesized CNs) and the overall capacitive/redox behaviour of the synthesised CNs.25
2.6. Biological studies
2.6.1 Antibacterial activity.
The antibacterial activity of the as-synthesized CNs (wsCDs and wsOLFs) was determined against a Gram-positive bacterial species (Staphylococcus aureus). For the assessment of antibacterial activity, 100 μL of the bacterial culture was inoculated onto nutrient agar plates. Three wells (6 mm) were made in the plate using a sterilized cork-borer and 20 μL of each test sample was placed in it. The as-prepared bacterial plates were then incubated at 37 °C for 24 h and the zone of inhibition was recorded.19
2.6.2 DPPH free radical scavenging activity assay.
The free radical scavenging capacity of wsCDs and wsOLFs was evaluated by measuring their scavenging activity on the 2,2-diphenyl-1-picrylhydrazil (DPPH) radical as reported previously with some modifications.26 The synthesized wsCD and wsOLF samples were used as catalysts for scavenging activity that were mixed at various concentrations with 100 μL of 125 μM DPPH solution in a final reaction volume of 200 μL in a 96 well microplate. Each experiment was repeated three times independently with quadruplet wells. The absorbance of the test and the blank wells having the vehicle alone were recorded after 1 hour at a 37 °C incubator using ascorbic acid as the positive control. The reduction of absorbance of DPPH was measured at 515 nm in a microplate reader (EPOCH-2, Biotek, India). The percentage of radical scavenging activity (RSC) was calculated using the following equation: | %RSC = [Absorbance (Blank) − Absorbance (Test)]/[Absorbance (Blank)] × 100 | (2) |
3. Results and discussion
3.1. Micro-structural properties of atmospheric wsCDs and wsOLFs
The morphological structure/image of atmospheric PM (PM10) and its chemical compositions in the initial state (pre-oxidation) are presented in Fig. S2 (see the ESI†). The nanostructures and compositions of the as-synthesized CNs derived from atmospheric PM were extensively studied by using the high resolution transmission electron microscopy (HR-TEM) technique. The particle size distribution of the CNs is depicted in Fig. 1. The synthesized wsCDs are homogeneous, quasi-spherical in shape, and are mono-dispersed or uniformly distributed without any agglomeration of particles (see Fig. 1a and b). Fig. 1c shows the histogram of the particle size distribution of the synthesized wsCDs demonstrating the particle size distribution with an average range of 4–6 nm. The atomic composition and elemental mapping of wsCDs (see the inset of Fig. 1a) were qualitatively identified by the TEM-energy-dispersive spectroscopic technique and found to predominantly contain carbon and oxygen atoms which was also confirmed by XPS atomic analysis (see Table S1†). The selected area electron diffraction (SAED) analysis shows that the synthesized wsCDs are mostly amorphous in nature (see Fig. 1d) with no observed crystal lattices in the particles. The H2O2 mediated oxidation process leads to the exfoliation of graphitized and nongraphitized polyaromatic hydrocarbons (PAHs) present in PM. Thereafter the PAH fragments were further converted into carbon dots on ultrasonication. The presence of hydrogen in atmospheric PM also plays a key role in carbon dot formation.19
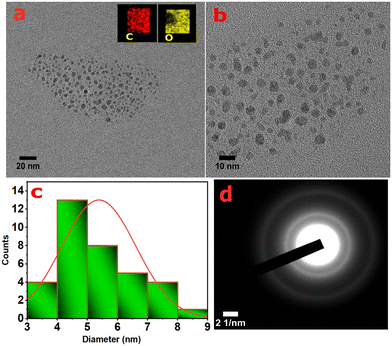 |
| Fig. 1 TEM micrographs of synthesized wsCDs: (a) overall view of particles at 20 nm scale showing a uniform distribution; (b) magnified view at 10 nm scale; (c) size distribution of carbon nanomaterials (wsCDs) having an average size of 4–6 nm; (d) the corresponding SAED pattern of wsCDs indicating that the particles are amorphous in nature. | |
The micro-structural morphology and size distribution of wsOLFs are presented in Fig. 2a (see the inset at the upper side) showing the aggregated particles. The particles are spherical or quasi-spherical in shape, with their corresponding particle size distribution having an average diameter ranging from 8–12 nm (see the inset at the lower side of Fig. 2a). The outer diameter of these wsOLF nanostructures ranges from a few to several tens of nanometers. The presence of the spherical morphology and onion-like structures of fullerenes is seen in Fig. 2a and b. At high resolutions, it is also observed that the synthesized wsOLFs exhibit a nanostructure containing short and irregular-shaped layers termed as lamellae (see Fig. 2c). Depending on the source of particulate matter, the length, curvature and inter-layer spacing of these layers (lamellae) varied.27 The nanostructure of wsOLFs exhibits graphitization at high resolutions and the graphite sheets appeared to be wavy (see Fig. 2c and d). It was reported that graphite sheets act as building blocks during the growth of wsOLFs.18
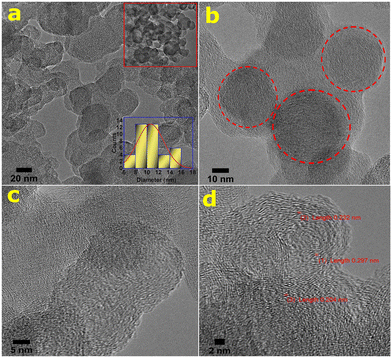 |
| Fig. 2 TEM/HR-TEM images of wsOLFs: (a) the particle morphology at 20 nm scale; the upper side inset figure shows the particle morphology of wsOLFs at 50 nm scale indicating that some particles are agglomerate types and the lower inset shows the particle size distribution with an average diameter ranging from 8–12 nm. (b) Onion-like structure of wsOLFs at 10 nm scale; (c) nanostructure of wsOLFs showing the formation of lamella-like structural organisation at 5 nm scale; (d) the quasi-spherical onion-like fullerene having the crystal lattice plane in the range of 0.232–0.297 nm. | |
In this study, the Soxhlet extraction process separated the organic carbon (i.e., PAHs) from atmospheric PM4 and the oxidation process helped in its conversion into fullerene particles.28 After Soxhlet extraction of PM, the concentration of total PAHs was observed to be 53.38 μg ml−1 as measured by the HPLC technique. It was well reported that PAHs can transform into fullerenes, when they are unable to stack into a regular form so as to create a graphitic structure.27 The graphitic-like structure can be broken and separated by means of chemical oxidation.18 Generally, it was also mentioned that the PAHs are joined by weak links and are preferentially oxidized during the synthesis procedure with HNO3 acid, leading to the formation of partially ordered and defective carbon layers. The heterogeneous nature of atmospheric PM, e.g., the presence of some ions (e.g., Na+ species) intercalated between the ordered and disordered layers in the extracted organic carbon structure, and rearrangement of the layers may also occur.18 PAHs in atmospheric particles and their random chaotic assemblage can lead to formation of a disorganized nanostructure particle core like the fullerene structure which is observed in our study. Fig. 2c and d show the fullerene rings (onion-like) overlay with each other while some are fused with other OLF rings owing to the fact that the net carbon particles growing rapidly are likely to close imperfectly and start a second shell before the first is completed.18 These micrographs (see Fig. 2d) also display onion-like concentric spherical shells and fringes of graphitic planes having interplanar distances in the range of 0.232–0.297 nm, which are smaller than that of graphite (0.336 nm) due to the cage curvature and pressure applied during the synthesis of CNs as reported elsewhere.29–31 Thus, the HR-TEM analysis clearly confirms the presence of an onion-like fullerene structure in the PM-derived products (wsOLFs).
The dynamic light scattering (DLS) results for particle size measurement of the CN (wsCDs and wsOLFs) samples are presented in Fig. S3 (see the ESI†). The mean hydrodynamic particle size is observed to be 141 nm and 166.4 nm for wsCDs and wsOLFs, respectively. The discrepancy in particle size in the TEM and DLS analyses is attributed to agglomeration, the larger hydrodynamic size of wsCDs and wsOLFs or the relatively smaller number of particles sampled during the TEM measurements. Such a discrepancy was also observed by other researchers.32,33 To further confirm the formation of wsCDs and wsOLFs from PM10, both the CNs were further investigated with the help of XRD, ATR-FTIR, Raman, XPS, UV-visible spectroscopy, and FL spectroscopy.
3.2. Observations from XRD and Raman analyses
The X-ray diffraction (XRD) analysis was carried out to determine the crystallinity nature of the synthesized wsCDs and wsOLFs (see Fig. 3a and b). The synthesized wsCDs showed a broad peak positioned at around 25.02° and wsOLFs showed a broad peak at 25.81° corresponding to the (002) plane of graphite. The d-spacing values of wsCDs and wsOLFs are calculated to be 3.61 Å and 3.55 Å, respectively, which are slightly higher than the d-spacing value (3.36 Å) of graphite.18,33 This may be due to the presence of amorphous carbon particles with oxygen-containing functional groups (like hydroxyl and carboxyl groups) and the existence of structural curvature and some structural defects in the synthesized CNs.18,33,34 Thus, XRD analysis, along with the results obtained from TEM, confirmed that the as-synthesized CNs contain both amorphous and crystalline structures. The degree of graphitization (G) was calculated from the XRD analysis following the Franklin equation:35,36 | G = (3.440 − d002)/(3.440 − 3.354) × 100% | (3) |
where 3.440 is the interplanar spacing in carbon having a turbostratic structure, d002 is the interlayer spacing value obtained from the XRD analysis, and 3.354 is the value of interplanar spacing in an ideal defect-free crystal of graphite. The as-synthesized wsCDs and wsOLFs have a degree of crystallinity/graphitization of around 42% and 36%. Table 1 summarizes the XRD characteristics of both the CN products, and the crystallinity results of wsCDs and wsOLFs are similar as reported elsewhere.36
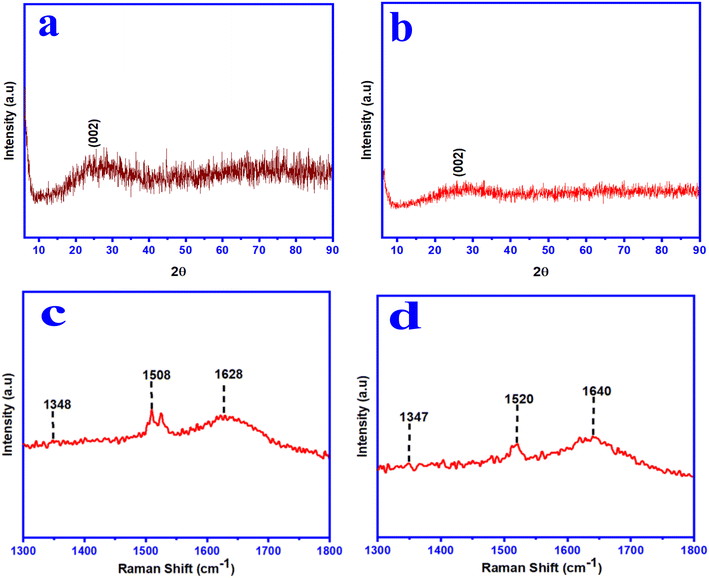 |
| Fig. 3 XRD analysis of PM10-derived wsCDs (a) and wsOLFs (b); as-synthesized wsCDs show a broad peak positioned at around 25.02° and wsOLFs show a broad peak at 25.81° corresponding to the (002) plane of graphite. Raman analysis of synthesized wsCDs (c) and wsOLFs (d). As-synthesized CN (wsCDs and wsOLFs) samples show the presence of both the D band (1347–1348 cm−1) and G band (1508–1640 cm−1) attributed to the sp3 and sp2 hybridized structure of carbon atoms. | |
Table 1 XRD analysis and degree of graphitization of CNs
Sample |
2θ (°) |
d
002 (nm) |
Single peak area |
Area of all peaks (crystalline + amorphous) |
FWHM (2θ°) |
G (%) |
wsCDs |
25.02 |
0.361 |
310.62 |
735.20 |
1.009 |
42 |
wsOLFs |
25.81 |
0.355 |
290.38 |
797.85 |
0.608 |
36 |
Raman spectroscopic analysis is one of the most effective and powerful techniques for characterizing carbon-based nanomaterials in identification of different carbon types, including carbon dots and fullerene-like carbon. In our study, the Raman spectra have mainly three absorption bands for both the synthesized CNs (wsCDs and wsOLFs) (see Fig. 3c and d). The characteristic bands appearing at 1348 cm−1 (D-band), 1508 cm−1 (G-band), and 1628 cm−1 (G-band) for wsCDs; and 1347 cm−1 (D-band), 1520 cm−1 (G-band), and 1640 cm−1 (G-band) for wsOLFs are attributed to the sp2 hybridized carbon (G-band) along with sp3 defects (D-band) in both the synthesized CNs, respectively. The D-band is attributed to vibrations of carbon atoms having dangling bonds in the termination plane of disordered graphite and they are mainly associated with lattice defects with sp3 carbon.18,37 The G-band arises from the vibrations of sp2 carbon atoms in the two-dimensional hexagonal lattice. The Raman peak observed at 1347–1348 cm−1 in both the synthesized CNs is due to the phonon confinement effect,3,38 and this peak corresponds to the residual ill-organised graphite.39 The characteristic bands observed between 1508 and 1640 cm−1 are attributed to the splitting of the E2g stretching mode of the graphite structure. The ratio of the intensities of the D and G-bands (i.e. ID/IG) was measured to be 0.69 for the synthesized wsCDs and 0.57 for the synthesized wsOLFs, respectively, signifying the well-graphitized structure in both the CNs formed from PM.37,40 Thus, the carbon structures are less defective in the synthesized CNs from PM10 by this chemical process. From this study, it is revealed that the as-synthesized CNs contain a nanocrystalline graphitic core surrounded by a disorganized/amorphous shell and our results show that the as-synthesized nano-products acquire a graphitic structure without any major surface defects.
3.3. Functional groups and chemical bonding of the PM-derived CNs
The surface functional groups and chemical bonding environment present in the as-synthesized CNs were studied by using the ATR-FTIR spectroscopic technique. The ATR-FTIR spectrum of the synthesized wsCDs shows that the presence of a broad peak position at ∼3453 cm−1 is associated with the stretching vibration of the –OH group (see Fig. 4a). The characteristic peaks at ∼1650 and ∼1100 cm−1 are due to the stretching vibration of the C
C and C–O groups, respectively. The peak observed at 619 cm−1 is attributed to the stretching vibration of the sp2 and sp3 C–H group in the synthesized wsCD product (see Fig. 4a). The FT-IR spectrum of the synthesized wsOLFs shows mainly two major peaks at ∼3297 and ∼1632 cm−1 corresponding to –OH and C
C stretching vibrations, respectively (see Fig. 4b). These FT-IR analysis data show similar trends to those of other studies of synthesized CDs15,35 and OLFs18,41 and confirm the dominance of oxygen containing functional groups on the surface of the synthesized CNs.
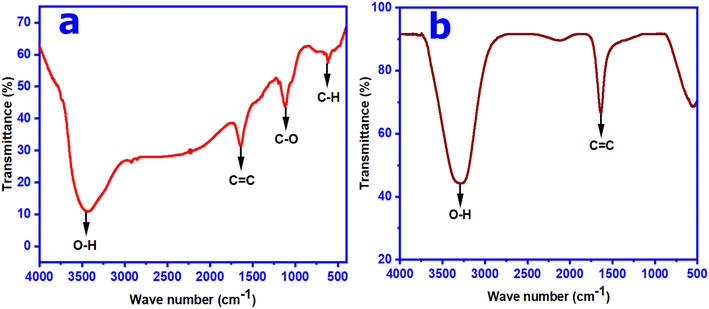 |
| Fig. 4 ATR-FTIR analysis of PM10 derived wsCDs (a) and wsOLFs (b). Both the as-synthesized (wsCDs and wsOLFs) samples present the dominance of oxygen-containing functional groups on the surface of synthesized CNs. | |
X-ray photoelectron spectroscopy (XPS) analysis was carried out to glean information on the structure or fraction of atoms (sp3 and sp2 hybridized structure) and the nature of chemical bonding in the synthesized CNs (wsCDs and wsOLFs). The survey spectra obtained in the XPS analysis for both the synthesized CN products are depicted in Fig. 5a and b; two major characteristic peaks are observed at 284 eV and 531 eV, corresponding to the presence of C (1s) and O (1s) elements, respectively. These survey spectra reveal the presence of N1s, Na1s, K2p, Si2p, and Cl2p in the synthesized wsCDs and N1s, S2p, Na1s, Si2p, and Ca2p in the wsOLFs. The atomic percentages and detail of the elements present in the synthesized CNs are summarized in Tables S1 and S2 in the ESI.†
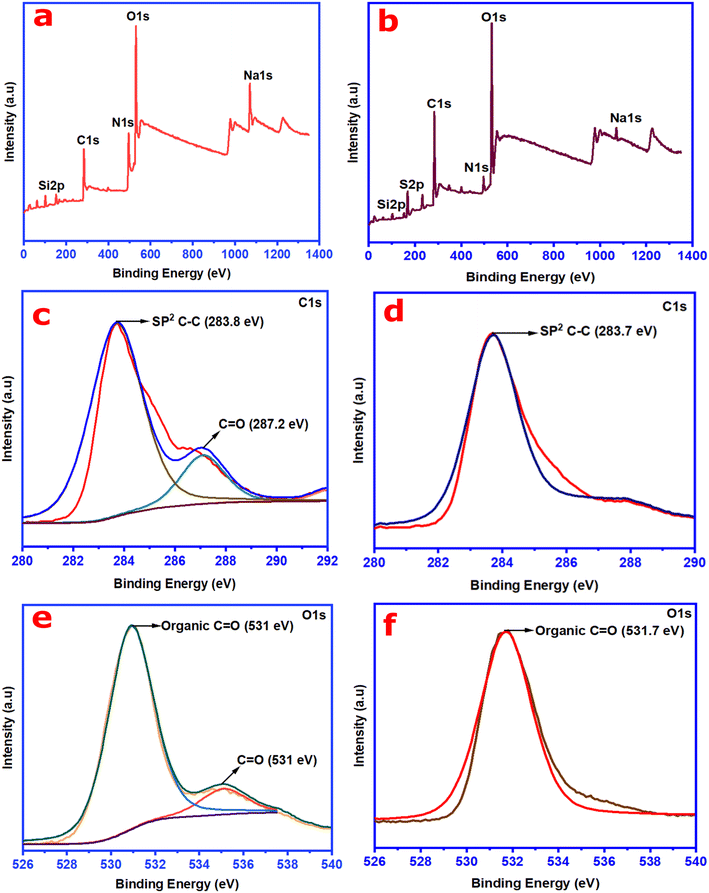 |
| Fig. 5 XPS survey spectra of PM10-derived wsCDs (a) and wsOLFs (b) illustrate the presence of C and O as major elements; deconvoluted XPS C1s spectra of PM10-derived wsCDs (c) and wsOLFs (d); deconvoluted XPS O1s spectra of PM10-derived wsCDs (e) and wsOLFs (f). The deconvoluted XPS spectra demonstrate that the as-synthesized CNs contain enriched oxygen having functional groups such as C–O, C O, and O–H, and structural aromatic fragments with sp2 hybridization. | |
Fig. 5c and d show the high resolution XPS deconvoluted spectra of C1s for the synthesized CNs from the atmospheric PM10 samples. As observed in Fig. 5c, the XPS spectrum of the C1s peak splits into two major peaks at 283.8 eV and 287.2 eV, attributed to the sp2 hybridized structure of carbon (C–C) and C
O for wsCDs.35 The C1s spectrum of the synthesized wsOLFs from the atmospheric PM10 is also deconvoluted having one characteristic peak positioned at 283.7 eV, corresponding to the sp2 hybridized structure of carbon (C–C) (see Fig. 5d). On the other hand, the deconvoluted O1s spectrum of wsCDs (see Fig. 5e) exhibits two major binding states of oxygen at 531 eV and 535 eV, representing the organic C
O group in wsCDs.3,19 However, in the case of wsOLFs, the deconvoluted spectrum of O1s displays only one binding state (see Fig. 5f) located at ∼531.7 eV corresponding to the organic C
O group41 The presence of the C
O/COO− group in both the synthesized CNs confirms the oxidation of the graphitic framework.41 Thus, from both the ATR-FTIR and XPS analyses, it is observed that the synthesized CNs contain enriched oxygen having functional groups such as C–O, C
O, and O–H as well as structural fragments of aromatic groups with sp2 hybridization. Therefore, the synthesized CNs (wsCDs and wsOLFs) are found to be water-soluble in nature.
3.4. Photophysical properties of the CNs derived from PM
The photo-physical characteristics of the as-synthesized CNs from atmospheric PM10 were investigated with the help of ultraviolet (UV-visible) spectroscopy and FL spectroscopy techniques. A fascinating characteristic of both the as-synthesized CNs (i.e. wsCDs and wsOLFs) is observed when they are irradiated with or exposed to UV light (365 nm wavelength). They exhibit bright blue fluorescence which can be easily seen in diluted colloidal solutions (see Fig. 6A(f)). To confirm the presence of conjugated π-domains with photophysical properties, the CNs were further investigated by UV-visible spectroscopy.
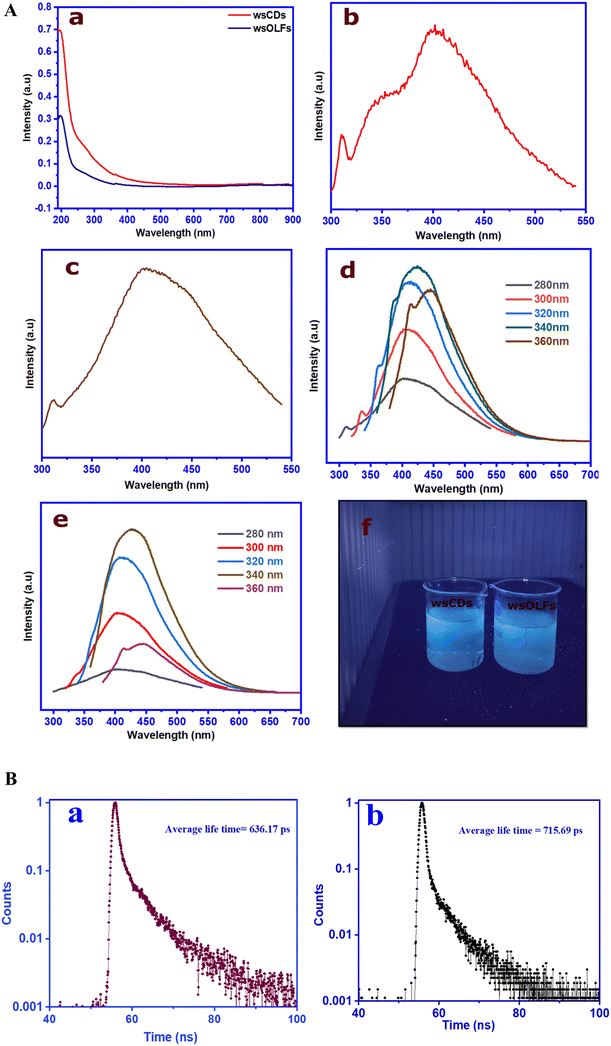 |
| Fig. 6 A (a) UV-visible spectra of the PM10-derived wsCDs and wsOLFs; FL spectra (excitation at 320 nm) of PM10-derived wsCDs (b) and wsOLFs (c); FL spectra of PM10-derived wsCDs (d) and wsOLFs (e) in different excitation states (280 nm, 300 nm, 320 nm, 340 nm, and 360 nm) showing excitation dependence; (f) image of wsCD and wsOLF extract solutions which are excited or radiated under UV light at 365 nm showing bright blue fluorescence. B Time-resolved single-photon counting spectra of the synthesized wsCDs (a) and wsOLFs (b) at neutral pH. | |
Fig. 6A(a) summarizes the UV-visible spectroscopic analysis of the synthesized CNs (wsCDs and wsOLFs) from atmospheric PM10 samples, indicating the presence of carbon nanostructures or similar shape.3,17,42,43 Both the as-synthesized CNs show absorption in the range of 200–210 nm (∼6.04 eV) due to the π-electron excitation (π–π*) of the aromatic π system and the n–π* transition of the C
C and C
O bonds present in the synthesized CNs (see Fig. 6A(a)). The synthesized CDs show higher intensities than the wsOLFs due to the presence of varied particle sizes and surface functionalized groups, as observed in the TEM and functional group analyses.14,17
Fig. 6A (b and c) show the fluorescence (FL) spectra of both the as-synthesized CNs at the excitation wavelength of 300 nm. Both wsCDs and wsOLFs have maximum FL intensity at around 402 nm, attributed to the blue region due to the higher intensity of the O–H group as compared to the C
O group. The n–π* transition is associated with the carboxylic group with a nonbonding orbital.35 The fluorescence properties of the synthesized CNs (wsCDs and wsOLFs) were also examined at different excitation wavelengths of 280–360 nm, at an increment of 20 nm (see Fig. 6A(d and e)). Both the synthesized CNs are found to be excitation dependent, and both the FL peaks produce a considerable blue-shift with the increase of the excitation wavelength stepwise from 280–340 nm due to the non-uniform particles of the CNs. Both the synthesized CNs show almost similar FL characteristics but the intensity of wsCDs is observed to be higher than that of wsOLFs which may be due to the high Raman ID/IG value of wsCDs obtained in the Raman analysis.18 The fluorescence characteristics of the synthesized CNs show similar results as compared to other related nanomaterial studies.15,19,35Fig. 6B presents the time-resolved single-photon counting spectroscopy of the synthesized wsCDs and wsOLFs at neutral pH. It is found that the average FL lifetimes of wsCDs and wsOLFs are different with values of 636.17 and 715.69 ps, respectively. It should be mentioned that the difference in the lifetimes of the as-synthesized CNs is due to the presence of different fluorophores and their impact on the surface of the carbon nanostructures.15,19 The observed lifetimes of the CNs also suggest that the synthesized wsCDs and wsOLFs can have potential applications in optoelectronics and bio-imaging.19
3.5. Quantum yield of the PM-derived CNs
The FL emission quantum yield (QY) of the PM-derived wsCDs and wsOLFs was found to be 3.35% and 6.09%, respectively. The yield of wsOLFs was observed to be higher as compared to that of the synthesized wsCDs. The difference in quantum yields between the wsCDs and wsOLFs is due to the use of different synthesis procedures with oxidants such as hydrogen peroxide for wsCDs and nitric acid for wsOLFs. The synthesized wsCDs show a similar yield to those reported in earlier studies.14,15 Thulashi et al.15 reported that the 3% quantum yield for CDs is good enough for different sensing-based applications.
3.6. Electrochemical application of the PM-derived CNs
The different applications of PM-derived carbon dots including their methods for synthesis and the QYs reported in other studies are also compared and summarized in Table 2. In addition, our approach is simple and transforms one of the toxic air particulate materials, PM10 as the precursor. To have a new and preliminary understanding of the electrochemical properties of the PM10-derived CN products, the cyclic voltammetry (CV) technique was used to evaluate their oxidation–reduction and capacitive behavior. For both the samples, the CV test was performed at varied scan rates of 20, 50, and 100 mV s−1 in a three-electrode system. Fig. 7 depicts the CV curves of these electrolyte solutions (i.e., synthesized CN samples) within the potential window of 1.6 V (cathodic scan) and −0.8 V (anodic scan) for the wsCDs, and 1 V (cathodic scan) and −0.5 V (anodic scan) for the wsOLF samples, respectively. From Fig. 7a, it is observed that the CV curve of the carbon dot solution has an irreversible reduction peak at cathode −0.4 V without an oxidation peak and is also symmetric, revealing the stable capacitive behavior of wsCDs.25,44–46 The results also showed that the cathodic peaks are similar at the same potential windows with varied scan rates, indicating that the electron transfer kinetics is irreversible.47–49 So, the electrolytes made of wsCDs show irreversible reduction at cathodic potentials due to the absence of the complete redox couple.50 The voltammetric behavior of the highly oxygen-functionalized wsCD sample is caused by electron transfer reactions on the nanoparticle surface, as seen in the CV curve, indicating that the redox reactions happened at the electrode potentials.51,52 Furthermore, as the scan rate is increased, the reduction peak currents rise substantially. The findings indicate that the wsCD electrolytes are electrochemically active and have the potential to be used as a promising electrolyte for small/minor electronic devices.53
Table 2 Comparison of CNs synthesized from different types of PM (synthesis methodologies, quantum yields, and applications)
Precursors |
Method of synthesis (time) |
Types of CNMs |
Average size (nm) |
Yield conc. of CNs (mg ml−1) |
Quantum yield (%) |
Applications |
Ref. |
Soot particles |
Hydrothermal (10 h) |
CDs |
4.2 |
— |
5.63 |
TNT sensing |
14
|
Soot particles |
Hydrothermal (8 h) |
CDs |
5.6 |
— |
3.25 |
TNT sensing |
14
|
Soot particles |
Acid refluxion (12 h) |
CDs |
∼4 |
— |
3 |
Tetrazine detection |
15
|
Black carbon |
Soxhlet assisted oxidation (6 h) |
Onion like carbon |
30 ± 5 |
— |
— |
Removal of dye like methylene blue |
37
|
PM
10
|
Ultrasound assisted oxidation (4 h)
|
wsCDs
|
4–6 nm
|
0.8
|
3
|
2-NP degradation, antibacterial and antioxidant activity, liquid electrolyte for small energy storage device
|
Present study
|
PM
10
|
Soxhlet assisted oxidation (10 h)
|
wsOLFs
|
8–12
|
1.39
|
6.09%
|
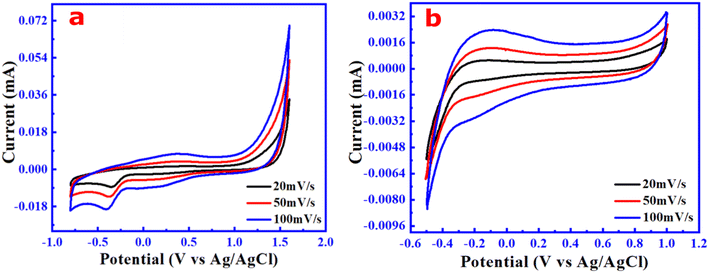 |
| Fig. 7 Cyclic voltammetry (CV) curves of a glassy carbon electrode (working electrode) in solutions containing (a) wsCDs (redox peak indicates the good capacitive behaviour) and (b) wsOLFs (non-faradaic nature curve indicates the good charge storage performance) as liquid electrolytes at different scan rates of 20, 50, and 100 mV s−1 (three-electrode system). | |
In contrast, the CV curve of the wsOLF solution (see Fig. 7b) generated quasi-rectangular shapes with no significant oxidation and reduction peak that indicates a stable capacitive behavior.52 The CV curves without redox peaks (non-faradaic nature) within the potential window ranging from −0.5 V to 1 V indicate the formation of electrical double layer charge propagation at the working electrode (glassy carbon)/electrolyte (wsOLFs) interface. This accessibility and transmission of electrons in the wsOLFs are due to the mesoporous nature of the fullerene (C60) microstructure.54,55
Thus, from the electrochemical studies, it is established that the as-synthesized wsCDs and wsOLFs from the atmospheric particulate matter (PM10) could be potential candidates as liquid electrolytes for electrochemical energy storage devices with some optimization. The as-synthesized wsCDs and wsOLFs with stable capacitive behaviour may be suitable for small (minor/general) electronic devices (0.1–10 F).56 However, to increase the conductive qualities of such materials, electrolyte production at a high operating potential window must be given special attention.
3.7. Biological applications of CNs (wsCDs and wsOLFs) formed from PM
The antibacterial properties of PM (ND-1) and the synthesized wsCDs (ND 2) and wsOLFs (ND 3) against a Gram-positive bacterial species (Staphylococcus aureus) were determined (see Fig. S4†). Fig. S4† clearly shows that the atmospheric particulate matter (PM) in water suspension (ND1) and its derived CNs like wsCDs could not inhibit the bacterial growth, significantly indicating that the atmospheric PM and its derived wsCDs (20 μl) do not have any antibacterial properties. Similar results are also demonstrated in other studies.19 On the other hand, the PM derived wsOLFs (ND 3) show the bacterial growth inhibition zone (see Fig. S4†). The as-synthesized wsOLFs show that the antimicrobial properties due to the presence of functional groups like carboxyl groups associated with fullerenes (anionic fullerenes) can affect the growth of bacteria as reported elsewhere.57
In addition, the anti-oxidant capacity of wsCDs and wsOLFs was also investigated by assessing their free radical scavenging potential using the DPPH molecule with the standard methodology where scavenging is reflected by reduction in absorbance due to the change of the original dark violet colour of the DPPH solution to a pale yellow or colourless state. Fig. 8a and b show the percent free radical scavenging activity of the wsCDs and wsOLFs where a dose-dependent increase in scavenging activity was observed. Though wsCDs and wsOLFs are nearly equivalent in terms of their DPPH radical scavenging activity, wsOLFs showed slightly better potential over wsCDs. Thus it can be considered that both wsCDs and wsOLFs possess some degree of anti-oxidant capacity which can have a favourable role in preventing cellular oxidative stress. Further, cell-based assays and animal studies are necessary to understand the impact of their anti-oxidant potential on health and diseases.
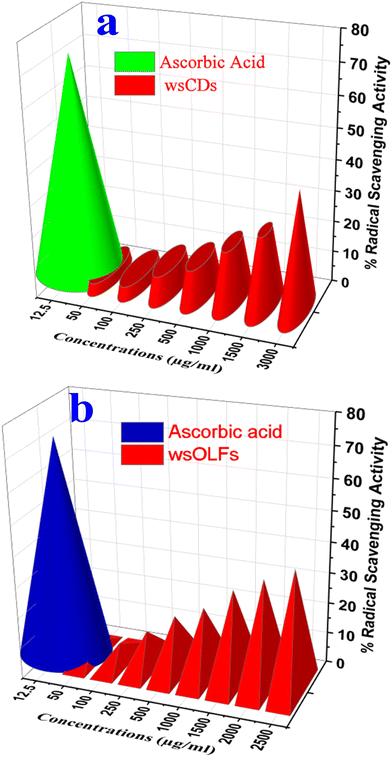 |
| Fig. 8 Free radical scavenging activity of (a) wsCDs and (b) wsOLFs using the DPPH assay. The wsCD and wsOLF samples were mixed at various concentrations (μg ml−1) with 100 μL of 125 μM DPPH solution and the percent radical scavenging activity (RSC) was calculated from the absorbance measured at 515 nm. The presented percent RSC ± SE is derived from the mean of three independent experiments with quadruplet wells in each experiment. | |
4. Summary
In summary, atmospheric carbonaceous particulate matter (PM10) turns into water soluble blue-photoluminescent carbon nanomaterials (i.e., wsCDs and wsOLFs) upon chemical oxidation with different oxidants opening up a new avenue for air pollution mitigation. The biological and environmental studies on these PM-derived CNs revealed their potential applications as antioxidants having a favourable role in preventing cellular oxidative stress. Alternatively, the fluorescence properties of these PM-derived carbon nanomaterials endow them with promising applications in biomedical research in the near future.
Conflicts of interest
There are no conflicts to declare.
Acknowledgements
The authors are grateful to the Director (CSIR-NEIST) for his keen interest in this environmental nanotechnology research. The authors are also thankful to MoEFCC (Govt. of India) and CSIR (OLP-2059) for funding, and Nazrul Islam also expresses thanks to CSIR for his Senior Research Fellowship to conduct the study. We also acknowledge the assistance received from Pankaj Barman, Joyshil Tamuly, and Santhi Maria Benoy during the research work.
References
- S. R. Mesquita, B. L. van Drooge and C. Reche,
et al., Toxic assessment of urban atmospheric particle-bound PAHs: Relevance of composition and particle size in Barcelona (Spain), Environ. Pollut., 2014, 184(555–562), 605 Search PubMed.
- N. Islam, A. Dihingia, P. Khare and B. K. Saikia, Atmospheric particulate matters in an Indian urban area: Health implications from potentially hazardous elements, cytotoxicity, and genotoxicity studies, J. Hazard. Mater., 2020, 384, 121472 CrossRef CAS.
- GBD 2016 Risk Factors Collaborators, Global, Regional, and National Comparative Risk Assessment of 84 Behavioural, Environmental and Occupational, and Metabolic Risks or Clusters of Risks, 1990−2016: A Systematic Analysis for the Global Burden of Disease Study 2016, Lancet, 2017, 390(10100), 1345–1422 CrossRef.
- N. Islam, S. Rabha, L. F. O. Silva and B. K. Saikia, Air quality and PM10-Associated poly-aromatic hydrocarbons around the railway traffic area: statistical and Air Mass trajectory approaches, Environ. Geochem. Health, 2019, 41, 2039–2053 CrossRef CAS.
- W. Yue, X. Li, J. Liu, Y. Li, X. Yu, B. Deng, T. Wan, G. Zhang, Y. Haung, W. He, W. Hua, L. Shao, W. Li and S. Yang, Characterization of PM2.5 in the ambient air of Shanghai city by analyzing individual particles, Sci. Total Environ., 2006, 368, 916–925 CrossRef CAS PubMed.
- L. D. Gladis, A. P. Antonio, C. R. Arturo, C. R. Telma, A. M. Omar and V. P. Rafael, Chemical and morphological characterization of PM2.5 collected during MILAGRO campaign using scanning electron microscopy, Atmos. Pollut. Res., 2012, 3, 289–300 CrossRef.
- X. Feng, Z. Dang, W. Huang, L. Shao and W. Li, Microscopic morphology and size distribution of particles in PM2.5 of Guangzhou City, J. Atmos. Chem., 2010, 64, 37–51 CrossRef.
- R. Zhang, A. Khalizov, L. Wang, M. Hu and W. Xu, Nucleation and Growth of Nanoparticles in the Atmosphere, Chem. Rev., 2012, 112, 1957–2011 CrossRef CAS PubMed.
- J. X. Yin and R. M. Harrison, Pragmatic mass closure study for PM1.0, PM2.5 and PM10 at roadside, urban background and rural sites, Atmos. Environ., 2008, 42, 980–988 CrossRef CAS.
- S. Fuzzi, M. O. Andreae, B. J. Huebert, M. Kulmala, T. C. Bond, M. Boy, S. J. Doherty, A. Guenther and M. Kanakidou,
et al., Critical assessment of the current state of scientific knowledge, terminology, and research needs concerning the role of organic aerosols in the atmosphere, climate, and global change, Atmos. Chem. Phys., 2006, 6, 2017–2038 CrossRef CAS.
- N. Islam, A. Dihingia, P. Manna, T. Das, J. K. Kalita, H. P. Dekaboruah and B. K. Saikia, Environmental and toxicological assessment of nanodiamond-like materials derived from carbonaceous aerosols, Sci. Total Environ., 2019, 679, 209–220 CrossRef CAS PubMed.
- D. C. Parashara, R. Gadi, T. K. Mandal and A. P. Mitraa, Carbonaceous aerosol emissions from India, Atmos. Environ., 2005, 39, 7861–7871 CrossRef.
- M. O. Andreae and P. Merlet, Emission of trace gases and aerosols from biomass burning, Global Biogeochem. Cycles, 2001, 15, 955–966 CrossRef CAS.
- S. Devi, R. K. Gupta, A. K. Paul, V. Kumar, A. Sachdev, P. Gopinath and S. Tyagi, Ethylenediamine mediated luminescence enhancement of pollutant derivatized carbon quantum dots for intracellular trinitrotoluene detection: soot to shine, RSC Adv., 2018, 8, 32684–32694 RSC.
- S. Thulashi, A. Kathiravan and M. A. Jhonsi, Fluorescent Carbon Dots Derived from Vehicle Exhaust Soot and Sensing of Tartrazine in Soft Drinks, ACS Omega, 2020, 5, 7025–7031 CrossRef PubMed.
- S. Rabha and B. K. Saikia, An environmental evaluation of carbonaceous aerosols in PM10 at micro- and nano-scale levels reveals the formation of carbon nanodots, Chemosphere, 2020, 244, 125519 CrossRef CAS PubMed.
- K. M. Tripathi, A. K. Sonker, S. K. Sonkar and S. Sarkar, Pollutant soot of diesel engine exhaust transformed to carbon dots for multicoloured imaging of E. coli and sensing cholesterol, RSC Adv., 2014, 4, 30100–30107 RSC.
- T. Das, P. K. Boruah, M. R. Das and B. K. Saikia, Formation of onion-like fullerene and chemically converted graphene-like nanosheets from low-quality coals: application in photocatalytic degradation of 2-nitrophenol, RSC Adv., 2016, 6, 35177–35190 RSC.
- T. Das, B. K. Saikia, H. P. Dekaboruah, M. Bordoloi, D. Neog, J. J. Bora, J. Lahkara, B. Narzarya, S. Roy and D. Ramaiah, Blue-fluorescent and biocompatible carbon dots derived from abundant low quality coals, J. Photochem. Photobiol., B, 2019, 195, 1–11 CrossRef CAS PubMed.
- T. Ishiguro, N. Suzuki, Y. Fujitani and H. Morimoto, Microstructural changes of diesel soot during oxidation, Combust. Flame, 1991, 85, 1–6 CrossRef CAS.
- R. L. Vander Wal, A. Yezerets, N. W. Currier, D. H. Kim and C. M. Wang, HRTEM Study of diesel soot collected from diesel particulate filters, Carbon, 2007, 45, 70–77 CrossRef CAS.
-
M. Saikia and B. K. Saikia, Carbon Dots Derived from Natural Carbon Sources: Preparation, Chemical Functionalization, Characterization, and Applications, All-carbon Composites and Hybrids, Royal Society of Chemistry, 2021 Search PubMed.
- L. T. Scott, M. M. Boorum, B. J. McMahon, S. Hagen, J. Mack, J. Blank, H. Wegner and A. de Meijere, A rational chemical synthesis of C-60, Science, 2002, 295, 1500–1503 CrossRef CAS.
- S. C. Ray, A. Saha and N. R. Jana,
et al., Fluorescent Carbon Nanoparticles: Synthesis, Characterization, and Bioimaging Application, J. Phys. Chem. C, 2009, 113, 18546–18551 CrossRef CAS.
- M. Bora, S. M. Benoy, J. Tamuly and B. K. Saikia, Ultrasonic-assisted chemical synthesis of activated carbon from low-quality subbituminous coal and its preliminary evaluation towards supercapacitor applications, J. Environ. Chem. Eng., 2021, 9, 104986, DOI:10.1016/j.jece.2020.104986.
- M. S. Blois, Antioxidant determinations by the use of a stable free radical, Nature, 1958, 181, 1199–1200 CrossRef CAS.
- A. J. Tiwari, M. Ashraf-Khorassani and L. C. Marr, C60 fullerenes from combustion of common fuels, Sci. Total Environ., 2016, 547, 254–260 CrossRef CAS.
- A. Nimibofa, E. A. Newton, A. Y. Cyprain and W. Donbebe, Fullerenes: Synthesis and Applications, J. Mater. Sci. Res., 2018, 7, 3 Search PubMed.
- Z. Czigany and L. Hultman, Interpretation of electron diffraction patterns from amorphous and fullerene-like carbon allotropes, Ultramicroscopy, 2010, 110, 815–819 CrossRef CAS PubMed.
- F. Banhart, The transformation of graphitic onions to diamond under electron irradiation, J. Appl. Phys., 1997, 81, 3440 CrossRef CAS.
- V. D. Blank, B. A. Kulnitskiy and I. A. Perezhogin, Structural peculiarities of carbon onions, formed by four different methods: Onions and diamonds, alternative products of graphite high-pressure treatment, Scr. Mater., 2009, 60, 407–410 CrossRef CAS.
- F. Rigodanza, M. Burian, F. Arcudi, L. Đorđević, H. Amenitsch and M. Prato, Snapshots into carbon dots formation through a combined spectroscopic approach, Nat. Commun., 2021, 12(1), 1–9 CrossRef.
- N. Javed and D. M. O'Carroll, Long-term effects of impurities on the particle size and optical emission of carbon dots, Nanoscale Adv., 2021, 3, 182–189 RSC.
- Y. Dong, J. Lin, Y. Chen, F. Fu, Y. Chi and G. Chen, Graphene quantum dots, graphene oxide, carbon quantum dots and graphite nanocrystals in coals, Nanoscale, 2014, 6, 7410–7415 RSC.
- R. Ye, C. Xiang, J. Lin, Z. Peng, K. Huang, Z. Yan, N. P. Cook, E. L. G. C. Samuel, C. Hwang, G. Ruan, G. Ceriotti, A. R. O. Raji, A. A. Martí and J. M. Tour, Coal as an abundant source of graphene quantum dots, Nat. Commun., 2013, 4, 2943 CrossRef PubMed.
- M. Saikia, T. Das, N. Dihingia, X. Fand, L. F. O. Silva and B. K. Saikia, Formation of carbon quantum dots and graphene nanosheets from different abundant carbonaceous materials, Diamond Relat. Mater., 2020, 106, 107813 CrossRef CAS.
- D. Hou, K. Li, R. Ma and Q. Liu, Influence of order degree of coaly graphite on its structure change during preparation of graphene oxide, J. Materiomics, 2020, 6, 628–641 CrossRef.
- T. N. J. I. Edison, R. Atchudan, M. G. Sethuraman, J. Shima and Y. R. Lee, Microwave assisted green synthesis of fluorescent N-doped carbon dots: Cytotoxicity and bio-imaging applications, J. Photochem. Photobiol., B, 2016, 161, 154–161 CrossRef CAS.
- L. Himics, S. Tóth, M. Veres, Z. Balogh and M. Koós, Creation of deep blue light emitting nitrogen-vacancy center in nanosized diamond, Appl. Phys. Lett., 2014, 104, 093101 CrossRef.
- A. Aqel, K. M. M. El-Nour, R. A. A. Ammar and A. Al-Warthan, Carbon nanotubes, science and technology part (I) structure, synthesis and characterisation, Arabian J. Chem., 2012, 5, 1–23 CrossRef CAS.
- R. Atchudan, A. Pandurangan and J. Joo, Synthesis of multilayer graphene balls on mesoporous Co-MCM-41 molecular sieves by chemical vapour deposition method, Microporous Mesoporous Mater., 2013, 175, 161–169 CrossRef CAS.
- J. Gunture, A. K. Kaushik, D. Garg, P. Saini and S. K. Khare, Sonkar, Pollutant Diesel Soot Derived Onion-like Nanocarbons for the adsorption of Organic Dyes and Environmental Assessment of treated wastewater, Ind. Eng. Chem. Res., 2020, 59, 12065–12074 CrossRef CAS.
- A. D. Lueking, H. R. Gutierrez, D. A. Fonseca, D. L. Narayanan, D. Van Essendelft, P. Jain and C. E. B. Clifford, Combined HydrogenProduction and Storage with Subsequent Carbon Crystallization, J. Am. Chem. Soc., 2006, 128, 7758–7760 CrossRef CAS.
- A. D. Lueking, H. R. Gutierrez, P. Jain, D. T. Van Essandelft and C. E. Burgess-Clifford, The effect of HCl and NaOH treatment on structural transformations in a ball-milled anthracite after thermal and chemical processing, Carbon, 2007, 45, 2297–2306 CrossRef CAS.
- X. Gu, Y. Yang, Y. Hu, M. Hu, J. Huang and C. Wang, Nitrogen-doped graphene composites as efficient electrodes with enhanced capacitive deionization performance, RSC Adv., 2014, 4, 63189–63199 RSC.
- T. Das, M. Bora and J. Tamuly,
et al., Coal-derived humic acid for application in acid mine drainage (AMD) water treatment and electrochemical devices, Int. J. Coal Sci. Technol., 2021, 8(6), 1479–1490 CrossRef CAS.
-
M. Rotami, Synthesis of Carbon Quantum Dots (CQDs) from Coal and Electrochemical Characterization, Doctoral dissertation, Ohio University, 2019 Search PubMed.
- L. Tian, D. Ghosh, W. Chen, S. Pradhan, X. Chang and S. Chen, Nanosized Carbon Particles From Natural Gas Soot, Chem. Mater., 2009, 21, 2803–2809 CrossRef CAS.
- Z. Zeng, W. Zhang, D. M. Arvapalli, B. Bloom, A. Sheardy, T. Mabe, Y. Liu, Z. Ji, H. Chevva, D. H. Waldeck and J. Wei, A fluorescence-electrochemical study of carbon nanodots (CNDs) in bio- and photoelectronic applications and energy gap investigation, Phys. Chem. Chem. Phys., 2017, 19(30), 20101–20109 RSC.
- T. S. Hernandez, M. Alshurafa, M. T. Strand, A. L. Yeang, M. G. Danner, C. J. Barile and M. D. McGehee, Electrolyte for Improved Durability of Dynamic Windows Based on Reversible Metal Electrodeposition, Joule, 2020, 4, 1501–1513 CrossRef CAS.
- M. S. Javed, S. Dai, M. Wang, D. Guo, L. Chen, X. Wang, C. Hu and Y. Xi, High performance solid state flexible supercapacitor based on molybdenum sulfide hierarchical nanospheres, J. Power Sources, 2015, 285, 63–69 CrossRef CAS.
- S. Zhang, Y. Li, H. Song, X. Chen, J. Zhou, S. Hong and M. Huang, Graphene quantum dots as the electrolyte for solid state supercapacitors, Sci. Rep., 2016, 6, 19292 CrossRef CAS PubMed.
- L. Yesappa, M. Niranjana, S. P. Ashokkumar, H. Vijeth, M. Basappa, J. Dwivedi, V. C. Petwal, S. Ganesh and H. Devendrappa, Optical properties and ionic conductivity studies of an 8 MeV electron beam irradiated poly(vinylidene fluoride-co-hexafluoropropylene)/LiClO4 electrolyte film for opto-electronic applications, RSC Adv., 2018, 8, 15297–15309 RSC.
- A. J. Khan, M. Hanif, M. S. Javed, S. Hussain and Z. Liu, Synthesis, characterization and charge storage properties of C60 fullerene microparticles as a flexible negative electrode for supercapacitors, J. Mater. Sci.: Mater. Electron., 2019, 30, 8568–8576 CrossRef CAS.
- Z. Shuoqing, T. Liu, D. Hou, W. Zeng, B. Miao, S. Hussain, X. Peng and M. S. Javed, Controlled synthesis of hierarchical birnessite-type MnO2 nanoflowers for supercapacitor applications, Appl. Surf. Sci., 2015, 356, 259–265 CrossRef.
-
P. Mars, A survey of supercapacitors, their applications, power design with supercapacitors, and future directions 2011, in IEEE Technology Time Machine Symposium on Technologies Beyond, 2020 Search PubMed.
- Y. J. Tang,
et al., Charge-Associated Effects of Fullerene Derivatives on Microbial Structural Integrity and Central Metabolism, Nano Lett., 2007, 7, 754–760 CrossRef CAS PubMed.
Footnote |
† Electronic supplementary information (ESI) available: Sampling locations, XPS, DLS, SEM-EDS, and antibacterial results including table and figures/images. See DOI: https://doi.org/10.1039/d2en00588c |
|
This journal is © The Royal Society of Chemistry 2023 |
Click here to see how this site uses Cookies. View our privacy policy here.