DOI:
10.1039/D2EN00545J
(Critical Review)
Environ. Sci.: Nano, 2023,
10, 11-40
Carbon nanomaterial-based membranes for water and wastewater treatment under electrochemical assistance
Received
10th June 2022
, Accepted 13th September 2022
First published on 16th September 2022
Abstract
Membrane separation has been widely used in water and wastewater treatment due to its relatively low cost, easy operation and high efficiency. However, current membranes suffer from a trade-off between selectivity and permeability as well as membrane fouling. Recently, numerous studies have focused on the development of carbon nanomaterial (typically, carbon nanotube and graphene)-based membranes with high performance for water and wastewater treatment. Many studies have demonstrated that benefiting from the good electrical conductivity of carbon nanomaterial-based membranes, their permselectivity and fouling resistance can be further improved under electrochemical assistance. Herein, we review the recent progress in the preparation, mechanisms and applications of electroconductive nanocarbonaceous membranes for water purification and wastewater treatment, aiming at improving the fundamental understanding of the combination of membrane separation and electrochemistry. Firstly, we summarize the recent methods for the preparation of some typical electroconductive membranes, for example, carbon nanotube membranes and graphene membranes. Subsequently, we discuss the underlying mechanisms (e.g., electro-oxidation, electro-adsorption, and electrostatic interactions) for the electrochemically enhanced permselectivity, antifouling and regeneration performance, and finally highlight the practical limitations of membrane/electrochemistry systems and outline possible solutions.
Environmental significance
Carbon nanomaterial-based membranes exhibit fascinating permselectivity for water and wastewater treatment because of their atomic-scale smooth graphitic pore walls and/or ultrathin thickness. Their permselectivity and fouling resistance can be further improved under electrochemical assistance, benefiting from their good electrical conductivity. This review summarizes the preparation methods and structures of typical carbon nanomaterial-based membranes and discusses their improved performance and new functions under electrochemical assistance. More importantly, we present a profound understanding of the motivation, mechanisms and challenges of coupling membrane separation with electrochemistry, which can help develop excellent membranes for water or wastewater treatment.
|
1. Introduction
Water is one of the basic elements required for economic and societal development. However, in the past decades, rapid urbanization and industrialization have caused a global water crisis in terms of increasing domestic, agricultural, industrial, and energy-related requirements for water resources.1 Besides the overall water shortage, water pollution is another worldwide problem posing threat to humans, resulting in a lack of access to suitable drinking water and proper sanitation. Therefore, significant efforts have been devoted to developing novel technologies for the production of fresh and potable water.
Membrane separation is considered to be a promising approach to supply potable and clean water through wastewater treatment and saline water desalination.2–4 Based on the pore-sieving mechanism, microfiltration (MF) can remove particulate pollutants (e.g., suspended solids and pathogens), while ultrafiltration (UF) is used to reject colloids, viruses, and macromolecular organic matter from water.2,4–6 In conjunction with the Donnan effect from the surface charge on the membrane, nanofiltration (NF) can efficiently remove multivalent ions and small charged contaminants.7,8 In the case of monovalent ions, reverse osmosis (RO) usually presents a removal efficiency of higher than 99% after overcoming the osmotic pressure.9 Also, some non-pressure driven membrane processes, such as membrane distillation (MD) and forward osmosis (FO), have recently attracted significant attention due to their high selectivity (theoretical value of 100%) for most substances in feed water, including salt ions and dissolved small organic molecules.10–12 Although membrane separation exhibits high performance for the production of fresh water to address the global water challenge with minimum environmental impact, it still suffers from two major problems of membrane fouling and trade-off between permeability and selectivity, hindering its further application. Membrane fouling, arising from the accumulation of rejected matter (e.g., natural organic matter, waterborne pathogens, colloids, and minerals) on the surface or in the pore channels of the membrane, usually causes a serious decline in flux, increase in maintenance cost, and shortened membrane life-time. According to the pore-sieving mechanism, the selectivity can be improved by reducing the pore size, but it would increase the energy-intensive operation pressure to obtain a high permeate flux. In addition, some small neutral molecular contaminants cannot be removed efficiently by the aforementioned pressure-driven membrane processes.
Electroconductive membranes have recently received significant attention due to their feasibility for the mitigation of membrane fouling and enhancement in rejection ability by integrating with electrochemistry.13–19 Due to the electrochemical reactions (e.g., electrooxidation, electroreduction, and electro-Fenton), electrochemically assisted membranes can degrade small-sized organic pollutants in a timely manner, which fail to be rejected by conventional membrane separation. Also, this electro-degradation behavior can prevent contaminants from adhering to the surface of the membrane and weaken the interaction between the membrane surface and deposited foulants to promote the fouling layer falling off from the membrane surface under other forces (e.g., shear force in cross flow). Alternatively, the electrostatic force can prevent charged foulants with same charge from depositing on the membrane surface and entering the membrane pores, thus mitigating membrane fouling. In addition, electrokinetic phenomena (e.g., electrophoresis, electroosmosis, and electromigration) have been confirmed to efficiently suppress fouling and improve the permeate quality. Thus, these developments in electrochemically assisted membranes have opened a new avenue to improve the separation efficiency and antifouling ability. Among the various materials for the construction of electroconductive membranes, carbon nanomaterials (typically, carbon nanotubes (CNTs), graphene, and graphene oxide (GO)) are most commonly used. These carbonaceous materials possess sp2 hybridized carbon atoms with delocalized π–π electrons, endowing them with high electroconductivity and catalytic activity. Meanwhile, nanocarbon-based membranes usually exhibit fascinating permselectivity due to their atomic-scale smooth graphitic pore wall and/or ultrathin thickness.20–22
Herein, we review the recent progress in the advancement of nanocarbonaceous membranes and electrochemically assisted membrane separation processes. In this review, firstly, we summarize the preparation, structure and performance of some typical nanocarbonaceous membranes. Then, we present diverse electrochemical approaches for minimizing the trade-off between selectivity and permeability and improving their antifouling ability and discuss the corresponding mechanisms. Finally, we critically discuss the challenges associated with the current nanocarbonaceous membranes and electrochemically assisted membrane processes, and present an outline on future solutions. Compared with previous reviews on the advancements of electroconductive membranes,23–30 this review specially focuses on carbon-nanomaterial-based membranes, with emphasis on the fundamental understanding of electrochemical membrane processes, including our understanding on why electrochemistry should be introduced in membrane processes and how it can improve the membrane performance. Additionally, this review will supplement the state-of-the-art research developments not covered in previous reviews. We aim to provide a guide to develop excellent electroconductive membranes using carbon nanomaterials and offer a profound understanding of the underlying mechanisms for the electrochemical assistance in water and wastewater treatment.
2. Motivation for coupling membrane separation with electrochemistry
In a typical membrane-based water or wastewater treatment process, water molecules penetrate the membrane, while pollutants are rejected. Thus, to maximize the filtration efficiency, water molecules are expected to pass through the membrane as quickly as possible, while all pollutants are removed. However, this is restricted by the properties (e.g., hydrophilicity and zeta potential) and structure (e.g., thickness, porosity and pore structure) of the membrane, as well as the feed conditions including pH, ionic strength, concentration and type of pollutants. Many of these factors affect the membrane performance through electrostatic interactions, for example, the electrostatic repulsion between the membrane and ions (Donnan effect), the electrical double layer at the membrane/solution interface, the electrostatic adsorption of ions and charged molecules by the membrane, and the ionization of carboxyl groups on the membrane. Accordingly, the rational regulation of the electrostatic interactions may significantly improve the membrane performance, for example, the increased rejection toward ions or charged molecules as a result of the enhanced Donnan effect during nanofiltration.
To remove both colloid particles and pollutant molecules using microfiltration and ultrafiltration membranes, a combination of membrane processes and AOPs have been proposed to construct integrated membrane/AOP systems.31–43 This is achieved by anchoring catalysts on the membranes, by which pollutants can be oxidatively decomposed with their electrons transferring to oxidants. Interestingly, electron-transfer-induced electrostatic interactions and chemical reactions are the focus of electrochemistry. This strongly suggests that electrochemistry can be integrated with membranes to enhance their performance by regulating a series of interfacial, electrostatic and reactive processes (Fig. 1), which has stimulated the attention of researchers in this field.44
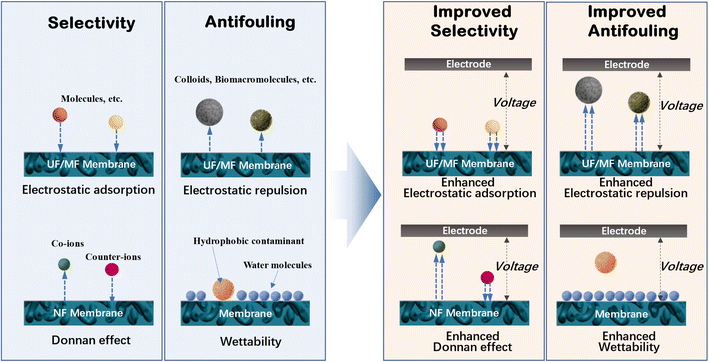 |
| Fig. 1 Scheme showing the motivation for coupling membrane separation with electrochemistry. | |
3. System of electrochemically assisted membrane process
To construct an electrochemically assisted membrane system, the conductive membrane should additionally function as an electrode, while another electrode needs to be placed opposite the membrane as a counter electrode, usually with a distance of several centimeters from each other, as schematically shown in Fig. 2a. When acting as an anode, the counter electrode should be an electrochemically stable metal (e.g., platinum, Ru–Ir–Ti alloy and titanium) or carbon (e.g., graphite and carbon cloth) plate/mesh. Alternatively, as the cathode, the counter electrode is usually a cheaper metal such as stainless steel. The voltages across these two electrodes are usually supplied by DC power. To precisely control the potential applied to the membrane, a reference electrode (e.g., Ag/AgCl electrode and saturated calomel electrode) needs to be introduced in the system to construct a three-electrode electrochemical system (Fig. 2b). The potential of the conductive membrane is usually provided by an electrochemical workstation. The system is usually used in a non-pressure-driven membrane process. It should be emphasized that, Fig. 2a and b show the simplest configuration of an electrochemically assisted membrane system, which is the foundation for the construction of more complex systems, for example, hollow-fiber-membrane-based electrochemical systems. Electrochemically assisted membrane filtration is carried out using a dead-end or cross-flow mode (Fig. 2c and d), which is similar to that used in the traditional membrane filtration process.
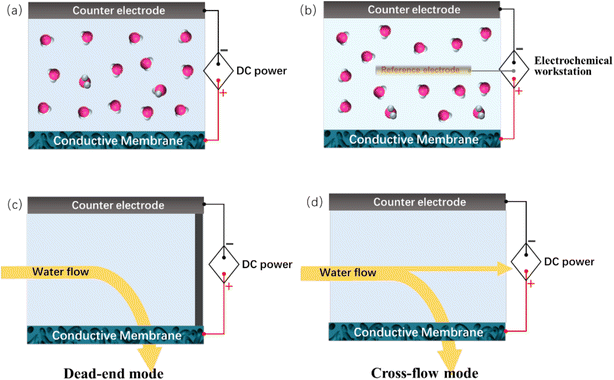 |
| Fig. 2 Schematic illustration of two-electrode system (a) and three-electrode system (b) of electrochemically assisted membrane process. Schematic illustration of dead-end mode (c) and cross-flow mode (d) of electrochemically assisted membrane process. | |
4. Preparation of electrically conductive nanocarbonaceous membranes
To achieve the integration of membrane separation and electrochemistry, the membranes should possess excellent electrochemical properties such as good electrical conductivity and electrochemical stability to allow electron transfer and long-time operation in water.45 Carbon materials, especially carbon nanomaterials, usually possess excellent electrical conductivity and stability because of their delocalized π-electron system and sp2 hybridized honeycomb networks with strong in-plane σ-bonds. Additionally, they have an atomically smooth surface or inner channels, or atomically thin structure, and therefore can be used to construct high-performance membranes with excellent selectivity and permeability.46–50 The advantages of these membranes have motivated the introduction of electrochemistry to further increase their performance and endow them new functions.
4.1 CNT-based membranes
CNTs are one-dimensional hollow fibrous nanomaterials, with inner/outer graphitic walls. This type of structure is very similar to the protein channels51,52 because they share some common features, for example, narrow hydrophobic pore channels with a selective tube entrance enriched with charged residues.53 Molecular dynamics and theoretical studies show the single-file confinement of water molecules in the hydrophobic CNT inner channels,54–56 which is similar to the water wires observed in aquaporins.51,52 Experimental observations evidenced that the aligned CNT membranes, whose pore channels are constructed by their inner walls, can allow ultrafast but selective mass transport.53,57–61 Besides the extreme confinement, the atomically smooth graphitic inner surface is also considered to be an important contributor to the super-rapid mass transport.62 The outer wall of CNTs can be also used to construct membrane pore channels, by stacking them randomly. The obtained membranes generally possess high porosity and high permeace,63,64 and are usually employed as a platform for other functional membranes, for example, antibacterial membranes65 and photocatalysis membranes.66 Despite the fact that the current CNT membranes (membranes whose pore channels are constructed by CNTs) possess diverse structures and morphologies, they can be intrinsically classified into two categories, i.e., aligned CNT membranes (Fig. 3a) and nonwoven CNT membranes (Fig. 3b).
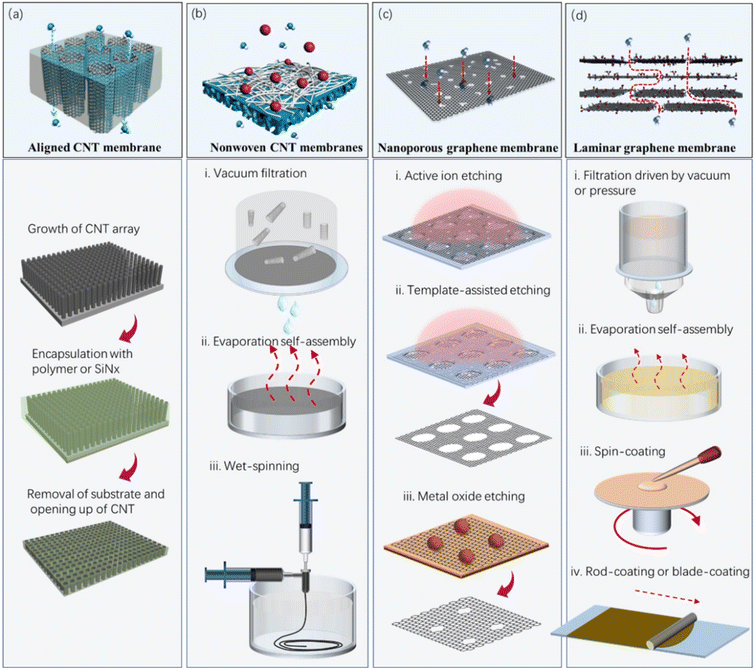 |
| Fig. 3 Schematic illustration of some typical nanocarbonaceous membranes and their main preparation methods: (a) aligned CNT membrane; (b) nonwoven CNT membrane; (c) nanoporous graphene membrane; (d) laminar graphene membrane. | |
4.1.1 Preparation and structure of aligned CNT membranes.
The preparation of aligned CNT membranes mainly involves three steps, as follows: (i) growth of an aligned CNT array, (ii) filling in the gap among nanotubes with a matrix, and (iii) opening of the CNT tips. CNT arrays are usually fabricated via the chemical vapor deposition (CVD) method, in which the fundamental mechanism is that the carbon atoms derived from the pyrolysis of organic compounds can rearrange on catalysts (e.g., iron, cobalt, and nickel) to continuously grow CNTs. Catalysts are usually introduced in the CVD system through their pre-deposition on smooth substrates or vapor-phase delivery. To force water to flow in the inner channels of CNTs, the gap among nanotubes has to be filled with a matrix, for example, polystyrene and Si3N4. Subsequently, it is necessary to remove the excess matrix and open the CNT tips to form permeable pores. This is usually accomplished via a plasma-enhanced oxidation process or mechanical cutting method.
During the preparation of some typical aligned CNT membranes, the key fabrication procedures vary. Hinds' group sealed the CNT gaps by spin-coating a 50 wt% solution of polystyrene and toluene on the surface of an aligned CNT forest.58 They removed excess polymer from the top surface and open the CNT tips via an H2O plasma-enhanced oxidation process. Later, Holt's group designed a more complicated but elaborate fabrication process, in which Si3N4 was used to fill the gaps via low-pressure CVD.60 The excess silicon nitride was removed from both sides of the membrane by ion milling, and the ends of the nanotubes were opened by reactive ion etching. Different from the reports by the Hinds and Holt groups, Mi67 and Baek68 separately developed physical methods (mechanical polishing and cutting) to remove excess filler and open the CNTs.
Aligned CNT membranes are initially fabricated for the purpose of investigating the transport behavior of fluids inside CNTs on the nanoscale, and thus the gap among the CNTs has to be blocked with matrix. Another idea of using aligned CNT forests for the construction of membranes has also been proposed. For example, Yu et al. reported a high-density, vertically aligned CNT membrane.69 Different from the membranes with sealed interstitial pores reported by the Hinds and Holt groups,57,60 the CNT membranes have no matrix, and consequently allow the permeation of water and gas through both the CNTs and interstitial pores. To achieve a uniform pore size and increased pore density, they compressed an as-fabricated CNT array into a dense array via solvent-evaporation-induced capillary forces. The average space between the CNTs after shrinkage was ∼3 nm, which is comparable to the pore size of the CNTs. The gas permeabilities of these membranes were 1–4 orders of magnitude higher than that of other aligned membranes reported in the literature. Alternatively, dense and matrix-free aligned CNT membranes could be obtained by mechanical compression.70–72
4.1.2 Preparation and structure of nonwoven CNT membranes.
The preparation of nonwoven CNT membranes typically begins with a well-dispersed CNT dispersion. It is well-known that CNTs have a strong tendency to aggregate due to van der Waals interactions. To disperse them in a suitable solvent, some physical and chemical strategies have to be employed, for example, ultrasonication, shear mixing, covalent functionalization of the CNT surface by strong-acid treatment and non-covalent functionalization with surfactant, polymer and biomolecules.64 The choice of the dispersion procedures should be in accordance with the type of CNTs and the final applications and should not adversely affect the desired CNT properties. The following procedure is the self-assembly of CNTs, which forms a macrostructure composed of randomly entangled CNTs. This process is typically achieved by evaporation, filtration or crossflow deposition73 of the CNT dispersion. The resultant CNT membranes typically have a nanometer-thin structure and can also be thick enough to act as a free-standing structure. Additionally, self-assembly fabrication endows a tunable pore size from several to hundreds of nanometers, which determines the membrane thickness and CNT diameters.
A porous support is usually essential for the majority of nonwoven CNT membranes during the filtration process because of their thin structure. In 2014, Quan's group developed free-standing CNT hollow fiber membranes composed entirely of CNTs.74 The membranes were prepared by first depositing CNTs on copper wires under a voltage, and then removing the template in a solution of FeCl3/HCl. To reduce the material-related cost and simplify the preparation procedures, they further developed a wet spinning method for the high-throughput preparation of CNT hollow fiber membranes.63 This method does not involve the self-assembly of CNTs on any surface as typically occurs in other methods, instead it molds the hollow fiber structure with polymers. Poly(vinyl butyral) is preferably chosen given that it can be easily removed in non-liquid phase conditions, avoiding the collapse of the hollow fiber structure. Most importantly, this process allows rapid and high-throughput fabrication.
To increase the mechanical strength of nonwoven CNT membranes, some organic or inorganic materials are usually used to strengthen them by crosslinking adjacent CNTs.75–77 Alternatively, CNTs are mixed with polymers to fabricate electrically conductive composite membranes.78–81 However, although these membranes have higher mechanical strength, they usually feature lower porosity and higher resistance than that composed entirely of CNTs. Thus, in the future, extensive efforts should focus on the development of low-cost and high-throughput methods for the preparation of the structurally stable, highly electroconductive and high-performance CNT membranes.
4.2 Graphene-based membranes
Graphene is a single layer of carbon atoms arranged in a sp2-bonded aromatic structure.82 Since its discovery in 2004,83 graphene has received great attention in the fields of basic physics, chemistry, materials science and device applications because of its unique two-dimensional monatomic layer structure, excellent mechanical strength and chemical stability. It is known from the Hagen–Poiseuille equation that the water flux of a conventional membrane is inversely proportional to its thickness.84 Graphene is the thinnest material in existence, and thus atomically thin graphene-based membranes can theoretically possess ultrahigh permeance.85 Experimental results show that the permeance of nanoporous graphene membranes for gas and water molecules is several orders of magnitude higher than that of the state-of-the-art polymer membranes. GO and reduced graphene oxide (rGO), as two derivatives of graphene, can also be used to fabricate high-performance membranes for water and wastewater treatment.
4.2.1 Preparation and structure of nanoporous graphene membranes.
The preparation of nanoporous graphene membranes involves two key procedures, i.e., chemical vapor deposition (CVD) growth of graphene and its perforation. CVD can yield large-area and high-quality single- or few-layer graphene,86 which is very important for the preparation of nanoporous graphene membranes. Graphene usually needs be transferred to a porous substrate under the assistance of a polymer for further processing. Theoretical and experimental investigations show that the defect-free graphene is almost impermeable to molecules and ions. To create nanopores for water permeation, some carbon atoms are removed from graphene by active ion irradiation85,87–90 or carbothermal reaction20 (Fig. 3c), which results in the formation of nanoporous graphene membranes. However, is still a great challenge for current technologies to fabricate large-area nanoporous graphene membranes, which is largely due to the difficulties in obtaining large-area graphene.
4.2.2 Preparation and structure of lamellar graphene (oxide) membranes.
As a derivative of graphene, graphene oxide has more defects and oxygen-containing groups than graphene fabricated by the CVD method, which is the result of the chemical oxidation of graphite. GO can be well dispersed in water, benefiting from its oxygen-containing groups such as –OH and –COOH. The preparation of lamellar GO membranes starts with a stable dispersion, and then GO nanosheets are stacked on a porous substrate through vacuum-filtration,91–95 interfacial self-assembly,96–98 spin-coating99 and rod-coating100 (Fig. 3d). In GO membranes, the pristine (unoxidized) regions of GO form atomically smooth nanochannels, with oxygen-containing groups on oxidized regions as spacers.99,101 Consequently, the narrow-distribution nanochannels allow an almost frictionless transport of water molecules, which largely accounts for the high permeance and selectivity of GO membranes.
GO membranes are prone to swell in water because of their high hydrophilicity, which results in an obvious expansion of their interlayer spacing and a decline in their separation performance.102 Thus, many attempts have been made to prevent GO membranes from swelling, including mechanical confinement,103,104 molecular crosslinking,105–108 cation–π interaction109–112 and chemical reduction.113–116 Molecular crosslinking and cation–π interaction can regulate the interlayer spacing of GO membranes by controlling the sizes of the molecular crosslinkers or cations. Their interlayer spacing can be also controlled by adjusting the degree of reduction of GO.117,118 RGO membranes are usually prepared by stacking rGO nanosheets or the reduction of GO membranes using chemicals, heat or laser.119,120 rGO membranes have a smaller interlayer spacing, higher structural stability and higher electrical conductivity than uncrosslinked GO membranes. Although the structurally perfect graphene has an outstanding conductivity, the rGO nanosheets fabricated by the oxidative exfoliation of graphite and subsequent chemical reduction has a large number of defects and residual oxygen groups, and therefore have relatively low conductivity.121 Thus, to increase the conductivity of rGO- and GO-based membranes, highly conducive nanomaterials (e.g., CNT) are usually embedded in their interlayers.122–124
4.3 Graphene-based composite membranes
In addition to higher structural stability, rGO membranes theoretically have a higher permeance than GO membranes as a result of the partial removal of their oxygen groups, which provides more nanochannels and decreases the hydrogen bonding interactions between water molecules and the hydroxyl groups, as demonstrated by molecular dynamics simulations.125,126 However, the removal of some oxygen-containing groups induces the restacking of some pristine graphene regions into graphite structures, which can reduce the number of the nanochannels for water transport, and also lower the hydrophilicity to increase the energy barrier for water molecules entering the nanochannels. Accordingly, the insertion of some hydrophilic nanomaterials (e.g., CNTs,122,127,128 carbon dots,129 metal–organic frameworks,130 and metal oxide131) in the rGO layer as spacers to prepare rGO-based composite membranes, to a certain extent, addresses the above-mentioned problems. Composite membranes are usually prepared via the vacuum filtration of a mixture of rGO and spacers122,127–129 or in situ growth of the spacers in the rGO layer.130
5. Mechanisms of membrane/electrochemistry integrated system
5.1 Electrically enhanced adsorption/desorption
Adsorption is a promising technique for the removal of low-concentration aqueous contaminants due to its easy operation and environmental friendliness. Carbon-based adsorbents, especially activated carbon power or granules, have been practically used in air and water clean-up. During the last few decades, carbon nanomaterials (e.g., carbon nanotubes and graphene) with graphitic walls have gained significant attention for adsorption due to their high specific surface area and chemical inertness. They provide abundant active sites for molecule or ion adsorption though hydrophobic interactions, π–π bonds, electrostatic interactions and hydrogen bonds.132 However, their irregular diffusion delays the arrival of the adsorbate at adsorption sites, resulting in slow adsorption kinetics.133 Membrane-based adsorption processes (membrane adsorption) significantly improve the adsorption kinetics, which benefiting from pressure-driven mass transport. Alternatively, the adsorption kinetics can be improved via electrochemical assistance. For example, if a positive potential is applied to the adsorbent materials, their adsorption performance towards anions and negatively charged molecules can be significantly enhanced as a result of the enhanced electrostatic attraction between the adsorbate and anode.134 Consequently, a combination of membranes and electrochemistry to form electrically assisted membrane adsorption systems has been designed (Fig. 4a) based on electroconductive membranes, such as CNT membranes, and evaluated in terms of kinetics and capacity. Experimental results have revealed a significant enhancement in the adsorption kinetics and capacity of CNT-based membranes when they are applied with a potential.133,135
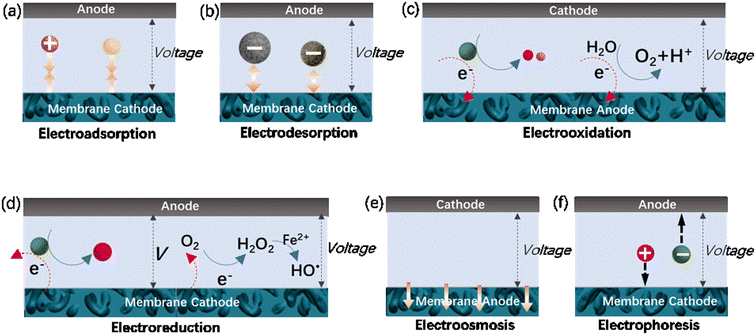 |
| Fig. 4 Some mechanisms of membrane/electrochemistry systems: (a) electroadsorption; (b) electrodesorption; (c) electrooxidation; (d) electroreduction; (e) electroosmosis; (f) electrophoresis. | |
Under electrochemical assistance, enhanced membrane desorption is also achieved by an opposite potential (Fig. 4b), given that electrostatic repulsion destroys the noncovalent interactions between the membrane and the adsorbate. Based on the mechanism that electroconductive membranes with different polarities prompt or prevent the formation of noncovalent interactions with charged targets, Quan's group developed a very simple biomimetic membrane system exhibiting a gated transmembrane performance with CNT hollow fiber membranes.135 The electrically assisted membrane system could typically regulate the transport of nanoparticles across its pore channels by their “opening” or “closing”, which is highly efficient and especially allows dynamic control. The gated system can also function for charged molecules, which can be efficiently trapped from water by electrochemically enhanced adsorption and rapidly released by electro-desorption to form a high-concentration solution for recovery, thus achieving water treatment and membrane renewal.
5.2 Electrically enhanced electrostatic repulsion
An electrostatic repulsive force will arise from the approach of ions to the like-charged membrane surface. This force is related to the distance between the membrane surface and ions, as well as the membrane surface charge densities. It is generally accepted that a larger surface charge density results in a higher electrostatic repulsive force and a stronger Donnan effect. Therefore, a membrane with a higher surface charge density usually exhibits a higher ion rejection.136 The application of a potential to the membrane has been found valid to further increase its charge density.122,136 According to the Donnan steric pore model, for a charged membrane, counter-ions will accumulate at the membrane–solution interface due to electrostatic adsorption, resulting in a higher concentration than that in the bulk solution. In contrast, the concentration of co-ions at the interface is significantly lower than that in the bulk solution, which creates a Donnan potential difference between the membrane and bulk solution. This potential difference can prevent both co-ions and counter-ions from transferring into the membrane.136 The application of an additional potential to the membrane can increase its surface charge density and enlarge the Donnan potential difference, thus enabling an improvement in membrane selectivity.
Electrostatic repulsive force can also occur between charged molecules (or colloids and particles) and the membrane. If enhanced by an applied potential, this force will prevent foulants from contacting or adhering the membrane surface, resulting in a much slower decline in flux than charged groups anchored on the membrane surface.80,137–143 For a particle, the electrostatic repulsion force, Fer, acting on it can be calculated using the surface elementary integration method (eqn (1)), where δFe/δx is the derivative of the free energy function at a separation distance x, where a is a constant partial radius and r is the variable radius, over which integration is performed.80,144 The van der Waals force (Fvdw), an attraction force of a particle toward a surface, can be calculated using eqn (2), where A is the Hamaker constant, r is particle diameter, and z is the ratio of separation distance to particle diameter (x/d).80 The overall electrostatic force (Ftotal) is the sum of all the forces with repulsive forces and van der Waals force as the positive and negative force, respectively (eqn (3)). It has been modeled that a potential of −1.519 V can produce a maximum repulsive force of 1.12 nN towards alginic acid particles, and evidence has shown that this repulsive force is greatly affected by the ion strength due to the charge screening effect.80
|  | (1) |
| 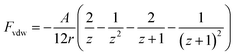 | (2) |
5.3 Electrochemical oxidation reactions on membranes
The rejection by membranes will inevitably result in blockage of their pores with inorganic foulants (e.g., inorganic salt and colloids), organic foulants (e.g., dyes, oils, natural organic matter and biomolecules) and biofoulants (e.g., bacteria and viruses).145 Membrane fouling significantly reduces the water flux temporarily or permanently, and increases the cost due to the increase in energy consumption and membrane replacement. In principle, membrane fouling cannot be completely eliminated but can be retarded by pre-treatment of raw water, optimization of the operation conditions, and development of antifouling membranes with reasonable structures, special surface morphology and chemistry. These strategies essentially aim at a reduction in the amount of foulant on/in the membrane during filtration, while maintaining high selectivity.
Other efforts have been also made to fabricate functional membranes that synergistically couple membrane separation and AOPs. Among these membranes, electrocatalytic CNT membranes have attracted significant attention due to their remarkable performance in pollutant removal and fouling mitigation by the electrochemical oxidation of pollutant molecules, natural organic matter and biomolecules rejected on the surface or in the channels of the membrane, as schematically shown in Fig. 4c.16,77,78,146–157 The decomposition of molecules can occur on the membrane anodes (M) through direct electron transfer or the generation of physically adsorbed “active oxygen” (adsorbed hydroxyl radicals, M(HO˙)) and/or chemisorbed “active oxygen” (oxygen in the oxide lattice, MO).158–162 Adsorbed hydroxyl radicals can be generated by water electrolysis (eqn (4)), and in some cases may interact with the active anode to form higher oxide MO (eqn (5)).158,162 The oxidation of organics (R) can be mediated by M(HO˙) or/and MO (eqn (6) and (7)).158–162 Organic foulants can be also destroyed by electrochemically produced prominent oxidants, such as peroxide, Fenton's reagent, Cl2, hypochlorite, peroxodisulfate and ozone.81,159 As an example of indirect oxidation, Cl− species in wastewater can be converted on the anode into Cl˙ or ClO2 with high oxidative ability.
| M + H2O → M(HO˙) + H+ + e− | (4) |
| M(HO˙) → M(O) + H+ + e− | (5) |
| M(HO˙) + R → M + mCO2 + nH2O + H+ + e− | (6) |
Benefiting from the electrochemical oxidation and other effects, improved pollutant removal and slower flux decline are experimentally observed during membrane filtration.76,77,156 Electrochemistry can alleviate membrane fouling; in turn, a pressure-driven membrane process is generally capable of accelerating the reaction dynamics.149,151 This can be evidenced by the fact that the current density increased by 6-fold during the electrochemical filtration of dyes by a CNT membrane compared to batch electrochemistry.151 Convection-enhanced mass transfer to the electrode surface is determined to be the primary factor for the increased current density.151 The reactive depth with phenol electro-polymerization as a direct probe also evidenced the convective mass transfer by membrane filtration.149 The oxidation kinetics and thermodynamics are also affected by the liquid flow rate, electrolyte concentration, target molecule concentration, anode potential and cathode material.153 With Fe(CN)64−/3− as a model soluble and nonadsorbing redox system, it was observed that the electrochemical kinetics increased linearly with an increase in the flow rate, while the electrolyte concentration had a negligible effect in the flow configuration due to the convective replenishment of Fe(CN)64−/3− and electrolyte to the CNT surface.153 The cathode material may affect the kinetics by altering the electron transfer path because of the cathodic production of H2. It has been also observed that a CNT cathode displayed a significant improvement in energy efficiency compared to a Ti cathode.153 The energy consumption for electrochemical membrane filtration was calculated at a total cell potential of 1.5–2.5 V to be 0.7–1.75 kW h kgCOD−1, which is much lower than that of the state-of-the-art electrochemical oxidation processes with energy consumption in the range of 5–100 kW h kgCOD−1.155
Additionally, direct oxidation and indirect oxidation can induce the inactivation of viruses and bacteria, preventing their multiplication on the membrane surface, which causes serious fouling.77,147,150 The primary mechanisms are similar to that underlying the electrochemical decomposition of organics.147,158 For the direct oxidation of pathogens (P), the first step involves their contact with the membrane anode (eqn (8)). Due to the negative charge of pathogens, their contact can be promoted by electrostatic attraction and an external electric field. Then, the electrons transfer from the pathogen to the membrane anode, featuring a multielectron process (eqn (9)). The indirect oxidation process mainly involves two steps, the first being the anodic one-electron production of active species, for example, HO˙, Cl˙ and SO4˙− in presence of H2O, Cl− and SO42−, respectively (eqn (10)). Secondly, the pathogen is oxidized (inactivated) by these species (eqn (11)). Evidence suggests that direct oxidation dominates the inactivation mechanism at a low voltage of <2 V.
| M(h+) + Ox−[H2O, Cl−, SO42−] → M + Ox˙[HO˙, Cl˙, SO4˙−] | (10) |
During the desalination process by nanofiltration or reverse osmosis, mineral scaling (typically CaCO3 and CaSO4 crystals) usually occurs on the membrane surface, resulting in a significant decrease in the water flux and salt rejection. Besides hydroxyl radicals, the electrochemical oxidation of water can also yield proton (H+, schematically shown in Fig. 4c, eqn (12)), which can dissolve the deposited CaCO3 crystals.81
| 2H2O → O2 + 4H+ + 4e− | (12) |
CNT membrane/electrooxidation systems have achieved the successful oxidative removal of a variety of targets such as dyes,148 anions,148 ferrocyanide,153 tetracycline antibiotic,155 natural organic matter76 and microcystin-LR.157 However, they are less effective for the electrochemical filtration of aqueous phenols because they can be electrochemically polymerized on the membrane electrode surface, resulting in its passivation.16 Accordingly, increasing the anode potential has been experimentally proven to be a feasible method to prevent polymer formation.152 Especially, a potential of >2.1 V is necessary for the complete oxidation of phenol to CO2 and H2O.152 However, this high potential will promote the generation of HO˙, which can oxidize CNTs, resulting in the instability of the membrane structure.163,164 Furthermore, the oxygen evolution reaction can occur on the CNTs and compete with the generation of reactive oxygen species, substantially reducing the current efficiency. Thus, there have been attempts to increase the resistance of CNT membranes to anodic potentials by anchoring or coating metal nanoparticles (Co3O4),165 bismuth-doped SnO2,166 antimony-doped SnO2,167 bismuth-and antimony-codoped SnO2 (ref. 167) or electroconductive polymer (polyaniline)168 on the surface of the CNTs, and results demonstrate good stability at a potential of up to 2.2 V (vs. Ag/AgCl).165,166
5.4 Electrochemical reduction reactions on membranes
The oxidation of pollutants to mitigate fouling and removal improvement can be also achieved using membranes as the cathode.169–171 This manipulation aims to achieve the production of hydrogen peroxide (H2O2), a relatively strong oxidant (E0 = 1.763 V vs. SHE), through the oxygen reduction reaction (ORR, Fig. 4d, eqn (13)). The in situ cathodic production of H2O2 may avoid the risks associated with its handling, transport and storage processes. Further improvement in the oxidative ability of the system was subsequently attempted by introducing a small amount of Fe2+ to construct an electro-Fenton system.171 To maintain the electro-Fenton reactions, the membrane structure should be specifically designed, often with asymmetric geometry. A typical example is the CNT-based electro-Fenton membranes consisting of 1) a CNT network cathode for O2 reduction to H2O2, 2) a CNT–COOFe2+ cathode for the chemical reduction of H2O2 to HO˙ and HO− and in situ regeneration of Fe2+ (eqn (14) and (15)), 3) a porous PVDF or PTFE insulating separator, and 4) a CNT filter anode for the remaining oxidation intermediates.171 | O2 + 2H+ + 2e− → H2O2 | (13) |
| H2O2 + Fe2+ → Fe3+ + HO− + HO˙ | (14) |
The other applications of electroreduction for fouling mitigation include the generation of H2 bubbles by the electrochemical reduction of water (eqn (16)) rather than O2. These microbubbles generated at the solid–liquid interface can destroy the filtration cake layer and lift foulants from the membrane surface. The effectiveness of this idea was experimentally confirmed by the significant increase in flux recovery with electrolysis.172,173 It should be noted that the HO˙ generated during the electro-Fenton reaction can react with many materials (for example, graphene and CNTs) and lower the membrane performance. Additionally, the formation and growth of H2 microbubbles on the cathode will probably destroy the membrane structure because of the weak van der Waals interactions among the nanomaterials in some nanocarbonaceous membranes. Despite the achievement that the structural stability of membranes can be enhanced by cross-linking with poly(vinyl alcohol) chains,78 more efforts should be still made to develop other solutions because cross-linking may decrease the electroconductivity and affect the surface chemistry of the membrane.
5.5 Electrically induced membrane wetting
Electrochemical control of surface wetting has generated significant interest due to the fundamental interest in surface science and its many applications in nanofluidic and separation technologies.174 The conformational reorientation of polyelectrolytes with hydrophobic alkyl chains and hydrophilic tips has been used for wetting control. As an example, a self-assembled monolayer of 16-mercapto-hexadecanoic acid deposited on a gold surface exhibited tunable hydrophilicity by choosing the exposure of the carboxylate tips (the molecule is straight) or alkyl chain (the molecule is bent), which was realized by the electroattraction or electrorepulsion between the charged carboxylate tips with gold substrate.175 Electrochemical reduction/oxidation of polyelectrolyte brushes or their electroactive counterions could also achieve wetting control. A typical example is that the neutral polypyrrole is usually slightly hydrophilic but the oxidized polypyrrole film shows hydrophobicity.176 Regulation of the charges at the solid/liquid interface or the direct oxidation of a solid surface is another electrowetting method to control the surface wettability.177,178 It has been reported that simple CNT papers (or films) can undergo a superhydrophobic to superhydrophilic switch177–182 if given a potential higher than the threshold.
Electrochemical wetting can find important applications in membrane filtration, ranging from improving the resistance to fouling and increasing the permeability to on-demand oil/water separation. Generally, hydrophilic membranes have a hydration layer on their surface, which weakens the hydrophobic interactions between the solutes or microbial cells and membrane, and therefore impart high fouling resistance.145 This has been confirmed by experimental investigations.183–185 Additionally, it is undeniable that compared with hydrophobic membranes, hydrophilic membranes are wetted more easily by water, and therefore provide more available pore channels for water transport under the same conditions. This means that the water permeance of originally hydrophobic or poor hydrophilic membranes can be significantly increased once they are electrochemically wetted. For oil/water separation, electrochemical wetting can render the membrane special functions. As an example, carbon fiber membranes decorated with poly-3-methylthiophene (P(3-MTH)) could undergo reversible switching between superhydrophobicity and superhydrophilicity, which was controlled by electrochemical doping or dedoping of ClO4− in P(3-MTH). This allowed on-demand oil/water separation (superhydrophobicity for the separation of water-in-oil emulsions and superhydrophilicity for the separation of water-in-oil emulsions).186
5.6 Electrochemically gated transport
The controlled transport of ions or molecules through some membranes (e.g., CNT membrane, MXene membrane, electro-conductive organic membrane, GO membranes and graphene) has been achieved using a voltage,187–194 demonstrating an electro-gating phenomenon (Fig. 5). Voltage-gated membranes usually feature variable channels or special molecular tethers, which are responsible for generating a voltage. For example, aligned CNT membranes functionalized with charged molecular tethers (diazonium salts) at the CNT tip entrances or along the CNT core showed voltage-gated control of ionic transport.187 The application of a positive bias could draw the negative-charged tethered molecules at the tips into the CNT core at a bias. For the molecules grafted along the CNT core, a negative bias extended the tethered molecules into the core. Thus, voltage-gated ion channels were obtained based on electrostatically actuated tether-induced steric hindrance, which makes the aligned CNT membranes a promising platform to mimic natural protein channels with a well-defined scaffold.
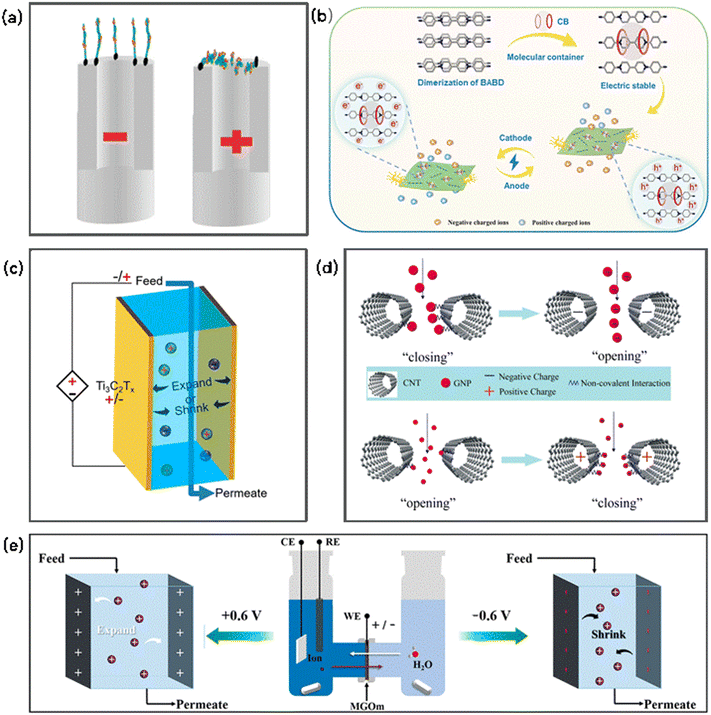 |
| Fig. 5 Schematic of electrochemically gated transport: (a) electrostatically actuated tethers induce steric hindrance in the CNT core to mimic voltage-gated ion channels (reproduced with permission from ref. 187. Copyright 2007, the American Chemical Society). (b) Scheme showing a voltage-gated membrane employing the host–guest interaction between a viologen and CB7 molecular containers (reproduced with permission from ref. 189. Copyright 2022, the American Chemical Society). (c) Intercalation and deintercalation of cations induced a variation in the interlayer spacing of Ti3C2Tx membranes (reproduced with permission from ref. 188. Copyright 2018, the American Chemical Society). (d) Schematic illustration of the mechanism responsible for the voltage-gated transport of nanoparticles across CNT membranes (reproduced with permission from ref. 135. Copyright 2015, the American Chemical Society). (e) Schematic diagram of voltage-gated ion transport behavior across an MXene/graphene oxide membrane (reproduced with permission from ref. 191. Copyright 2021, the American Chemical Society). | |
5.7 Electroosmosis flow in membrane
Electroosmotic flow, relying on a high density of fixed surface charge and an electric field that pumps neutral solvent and solutes in the flow (schematically shown in Fig. 4e), has found various application including lab-on-chip diagnostics, medical implants, drug delivery and chemical separations. It is considered that the ideal conditions for efficient electroosmotic flow in nanoporous membranes or channels are a high surface charge density, small diameter and slippery interface to efficiently transmit flow through the membrane.195 CNT membranes are an excellent candidate because they possess atomically smooth graphitic cores, allowing fast fluid, the ability to covalently functionalize their pore entrances, and good electro-conductance, allowing for the concentration of the electric field at the CNT tips. Experimental results showed that neutral caffeine molecules can be efficiently pumped via electroosmosis into CNT membranes (inner diameter ranging from 1.5–7 nm) functionalized via electrochemical diazonium grafting and carbodiimide coupling reaction, with an electroosmotic velocity as high as 0.16 cm s−1 V−1.179 Due to the many advantages of CNT membranes, the power efficiencies were 25–110 fold improved compared to the related nanoporous materials.195 Another investigation has demonstrated that the rate and direction of electroosmotic flow across template-prepared CNT membranes can be controlled by changing the magnitude and the sign of the transmembrane current.196 Membrane-based electroosmosis is expected to have important applications in chemical separation and compact medical devices.
5.8 Electrophoretic motion of ions in nanochannels
Electrophoresis refers to the phenomenon that charged substances move through liquids and pores in the presence of an electric field, as schematically shown in Fig. 4f. Electrophoresis-based micro- and nanofluidic devices have been used for applications such as inorganic ion detection, DNA sequencing and translocation, peptide and protein separation and nanofluidic transistors and diodes. Membranes based on CNTs are attractive for these devices and their associated applications because of their many excellent properties, for example, atomically smooth graphitic walls and good electrical conductance. It has been reported that the electrophoretic mobilities of ions in these membranes are approximately 3-times higher than the bulk mobility. Moreover, the induced electro-osmotic velocities are 4-orders of magnitude faster than that measured in conventional porous materials.197 By choosing single-walled CNTs (average inner diameter of ∼0.9 nm) and large anion/cation pairs (diameter of ∼1 nm), the membrane can function as a rectifying diode due to the ionic steric effects in the nanotubes.197
6. Improved permselectivity of carbonaceous membranes under electrochemical assistance
Benefiting from the aforementioned fascinating electrochemical functions, electrochemically assisted nanocarbonaceous membranes have been investigated for the removal of various pollutants from water, such as inorganic ions, organic matter and waterborne pathogens.
6.1 Inorganic ion removal
Heavy metal ions, which are common in the aqueous environment, are very dangerous to human beings due to their carcinogenicity and teratogenicity.198–200 However, the conventional MF and UF membranes fail to reject them efficiently, given that their pore size is much larger than these ions. NF and RO membranes can purify the water containing heavy metal ions, but usually with fairly low permeability. Given that these ions are charged in water, they can be electrochemically adsorbed on carbonaceous MF and UF membranes with opposite charges, after which an electrochemical reaction occurs for the detoxification of the absorbed metal ions.201 This integrated separation mechanism of electro-adsorption and electrochemical reduction/oxidation was successfully used to rapidly and effectively remove arsenic and Sb(III) ions using an electroconductive TiO2–CNT membrane.19,202 In comparison with the batch mode, the flow-through mode enabled convection-enhanced mass transport and a higher adsorption capacity. Additionally, the electromigration and electrostatic interactions enhanced the near-surface transport of ions, which accounts for the improved Sb(III) sorption kinetic and capacity under electrochemical assistance. Although CNTs showed negligible specific affinity for Sb(III), the transformation of Sb(III) to the less toxic Sb(V) could occur on the TiO2 nanoparticles. Meanwhile, the synergistic effects between the CNT membrane and TiO2 significantly improved the Sb(III) removal in a recirculated filtration system. The electroactive TiO2–CNT membrane could work effectively over a wide pH range and even in the presence of sulfate, chloride, and carbonate ions. Based on this removal mechanism, Liu et al. achieved the one-step detoxification and sequestration of As(III) to low toxic As(V) at a positive potential with a sea urchin-like FeOOH-functionalized CNT membrane.203
When carbonaceous UF membranes worked as a cathode during the separation process, hexavalent chromium was efficiently removed as a result of the electrostatic repulsion and electrochemical reduction.204 Also, the electro-Fenton process occurring near the cathodic membrane could achieve the rapid detoxification of heavy ions.205,206 For a Ti–Ce modified carbonaceous membrane/electrochemistry system, the in situ-generated H2O2 reacted with a Ti–Ce binary oxide to produce hydroperoxide complexes, enabling the efficient transformation of Sb(III) to the less toxic Sb(V) (τ < 2 s) at neutral pH. Besides, the complete Sb(III) detoxification in a flow-through electro-Fenton system was achieved through the enhanced production of HO˙ and HO2˙ because of effective Fe3+/Fe2+ cycling.206
Besides heavy metal ions, electrochemically assisted membrane filtration can also recover non-metallic elements. Kekre et al. recovered N and P elements from livestock wastewater as struvite powder using an electrically conductive CNT membrane.207 Jung et al. enhanced the boron rejection by a CNT-coated RO membrane through local electrochemical pH modification.208 Also, the rapid transformation of ammonia to nitrogen was effectively achieved through a continuous-flow chloride-radial-mediated electrochemical system based on an anodic carbonaceous membrane.209
In general, NF membranes reject multivalent ions and charged molecule through both pore-sieving and Donnan effects, but usually suffer from a trade-off between permeability and selectivity. Zhang et al. found that optimizing the surface charge and pore size could co-enhance the permeability and selectivity of an rGO/CNT NF membrane.128 It was found that an external cathodic potential could significantly improve the rejection ability of NF membranes toward ions without sacrificing their permeability.129,136,210,211 The Donnan steric pore model analysis suggested that the Donnan potential difference between the membrane and bulk solution increased under electrochemical assistance, leading to an increase in ion transfer resistance for ion rejection. Furthermore, the electrokinetically driven water transport velocity could be significantly increased with an increase in external voltage applied across the membrane and counter electrode.210 Consequently, both the rejection rate of NaCl and the water flux were improved under electrochemical assistance. Numerical analysis confirmed that the increased rejection rate was attributed to the enhancement in the electrokinetic transport of water through the membrane and ion partitioning between the membrane and bulk solution. Although the permselectivity could be significantly improved by a potential, charge screening is still the major problem for desalting high-salinity water, in which the Donnan effect is weakened as a result of the compressed thickness of the electric double layer.
Chlorine-resistance is one of the critical properties that dominate the long-term operation of RO membranes. Khanzada et al. prepared a conductive CNT-embedded RO membrane for the prevention of both Cl attachment and degradation of the active polyamide layer, which was achieved by the timely electrochemical reduction of chlorine on the membrane surface.212 This electrochemically assisted RO membrane exhibited a much higher stability in salt rejection than that without electrochemical assistance after long-term chlorination at acidic pH.
6.2 Organic pollutant removal
In recent decades, various toxic organics have been frequently detected in the aquatic environment, thus receiving increasing attention.213–216 The dissociative organic molecules are difficult to be rejected by conventional MF or UF membranes. However, they can be effectively removed by nanocarbonaceous MF or UF membranes coupling with electro-adsorption, electrooxidation, electroreduction and/or electro-Fenton process.24,217–225 During the electrochemically assisted processes, the molecules are captured and/or decomposed quickly when they migrate toward or pass through the nanocarbonaceous membranes, achieving high permselectivity.219,226–229 Moreover, the convection in the membrane pore efficiently improves the mass transfer to promote the electrochemical reactions.230 The removal of dye molecules via electroconductive nanocarbonaceous membrane has been systematically investigated by both experiments and numerical simulations.119,223,231–233 Direct electron transfer is the major mechanism for dye oxidation, which is affected by the charge properties of dye molecules. Meanwhile, the crossflow mode is beneficial for the removal of organic molecules due to the presence of shear flow, which allows a consistent surface coverage on the electrochemical membrane.234 Despite the fact that the produced water was colorless during electrochemical separation, the dye was merely decolorized because of azo bond breaking. Meanwhile, the membrane anode was passivated during long-term application in phenol removal.149,152 Thus, to address these problems, some element such as boron was doped in the electrically active membranes to improve their oxidation ability and stability.16 Compared with the undoped CNT membranes, the boron-doped CNT membranes showed a higher resistance to passivation and a long-time operation. The specific oxygen functional groups on the CNT surface have a strong effect on the anode performance.235 It was found that the anodic performance of a CNT membrane anode could be increased by 2-fold in regards to anodic phenol mineralization after the CNT surface was modified with hydroxyl (–OH), while its performance decreased by 2-fold if carboxyl (–COOH) was decorated on the CNT surface. This is attributed to the fact that CNT–COH membranes have a lower phenol electrooxidation overpotential and lower resistance to charge transfer.
The modification of carbonaceous membranes with other active materials is an alternative to prevent electro-corrosion during the electrochemical mineralization of organic pollutants. Some researchers developed an anodically stable polyaniline–carbon nanotube (PANI–CNT) composite membrane using PANI as a protection layer.168,224 This membrane required only 2.5 kW m−3 power to electrooxidize methylene blue (5 ppm) with a contact time of <1 s.168 However, the PANI–CNT membrane only worked at neutral and basic pH because of the sensitivity of PANI to electrooxidation (first-order rate constants of ∼10−3 s−1) under acidic conditions. Besides, semiconductive materials have been also used to improve the oxidation ability and stability of carbonaceous membranes under positive potentials. The deposition of SnO2–Sb on a carbon nanofiber membrane could increase the oxygen evolution potentials and degradation of tetracycline at a voltage of 2.5 V.236 Bismuth-doped SnO2 (BTO)-coated CNT membranes exhibited improved anode stability and efficiency for flow-through organic electrooxidation.166 Additionally, a BTO coating significantly suppressed the oxidative corrosion of CNTs in aqueous solution even at an anodic potential of >2.2 V, while the uncoated CNT was stable at a potential of <1.4 V.
When a negative potential is applied to nanocarbonaceous membranes, they can also degrade partial organic matters through electrochemical transformation.201,237 Researches have demonstrated that they can achieve the simultaneous dehalogenation and oxidation of halide.237 Because oxygen can be cathodically reduced into H2O2 on carbonaceous catalysts,238–240 the integration of electro-Fenton and membrane separation has drawn increasing attention to remove various organic pollutants (Fig. 6a), such as dye molecules,241,242 persistent oxalate,170,171 and antibiotics.123,243–245 For example, a graphene-modified electro-Fenton catalytic membrane (EFCM) was utilized for the in situ degradation of low-concentration antibiotic florfenicol.17 It was demonstrated that the EFCM could function as both a cathode for electro-Fenton oxidation process in continuous mode and membrane barrier to concentrate florfenicol to enhance the mass transfer. The removal rate of florfenicol by EFCM was 2–4 times higher than that of the single filtration and batch electro-Fenton processes. Similarly, during the treatment of paracetamol, a pharmaceutical pollutant, the mineralization current efficiency increased remarkably by 165% and remained stable for 3 consecutive cycles in comparison with electro-Fenton batch experiments.246
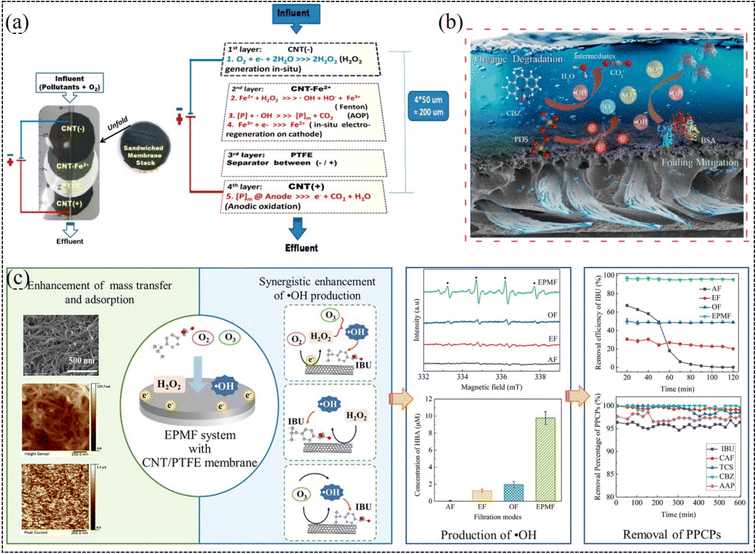 |
| Fig. 6 (a) Electro-Fenton-assisted membrane separation (reproduced with permission from ref. 171. Copyright 2015, the American Chemical Society). (b) Electro-filtration-activated peroxydisulfate system (reproduced with permission from ref. 249. Copyright 2022, Elsevier). (c) Electro-peroxone-assisted membrane separation (reproduced with permission from ref. 250. Copyright 2022, Elsevier). | |
When a carbonaceous membrane worked as both a separation membrane and cathode, other AOPs could be also integrated in the membrane/electrochemistry system to effectively remove organic pollutants (Fig. 6b). Previous studies have demonstrated that in a membrane/electrochemistry/persulfate system, a series of radical and nonradical reactions could collectively result in the degradation of persistent organic pollutants at an ultra-fast rate.247–249 The electroreduction reaction ensures the efficient redox cycle of the catalyst for long-term operation. When integrated with ozonation, nanocarbonaceous membranes exhibited enhanced organic mineralization and toxicity reduction under electrochemical assistance (Fig. 6c).250–252 However, although the ozonation process could completely remove tetracycline, it only removed 9.1% total organic carbon (TOC) in 20 min. Alternatively, when an electrochemical membrane process was added together with the ozonation process, the TOC removal ratio of effluent reached 77.1% under the optimal conditions. More importantly, the energy consumption of the coupled process was 101.5 kW h kgTOC−1, which is lower than that of single ozonation (241.2 kW h kgTOC−1) and electrochemical membrane process (121.5 kW h kgTOC−1).251
6.3 Disinfection
Waterborne pathogens including viruses and bacteria usually cause serious diseases and death. It has been reported that nanocarbonaceous membranes can inactivate almost 100% viruses and bacteria with the application of a suitable potential.120,149,253–255 The positive potential-induced viral disinfection was immune from natural organic matter and calcium salt, resulting in minimal fouling to the membrane.150 The possible disinfection mechanism was that the virus was firstly adsorbed through electrostatic interactions between the viral particles and the anodic membrane, and then inactivated because of intracellular protein leakage caused by direct oxidation, indirect oxidation, permeabilization and electroporation of the cells. Some researchers consider that bacteria inactivation may be also caused by the water electrolysis altering the local acidic and alkaline environment.
7. Electrochemical fouling mitigation, fouling monitoring and regeneration of nanocarbonaceous membranes
Although membrane fouling can be significantly mitigated using various technologies, it is still an inevitable problem during membrane separation processes. Thus, more efficient antifouling strategies are required to lower the total economic consumption of the membrane separation processes associated with membrane regeneration and replacement. Recently, electrochemistry-based approaches for fouling mitigation have received great attention given that they are generally nondestructive to membranes.256,257
7.1 Electrochemical fouling mitigation of nanocarbonaceous membranes
7.1.1 Improved resistance to organic fouling.
Natural organic matter is ubiquitous in the water environment due to the decay of plants and animals. These organic matter can cause serious fouling during membrane separation as a result of their physicochemical interactions with the membrane surface.258,259 Therefore, suitable strategies are required to prevent these organic foulants from depositing on the membrane surface and entering the membrane pores. It was found that the electrochemically assisted membrane process experienced very little organic fouling during the long-term treatment of anaerobic reactor effluent.260,261 The enhanced antifouling performance was attributed to the in situ membrane cleaning.147,262 The H2 and O2 microbubbles generated from water electrolysis also reduced membrane fouling by preventing the formation of a cake layer.263
For the charged organic foulants in water, a common antifouling method is to impart a same-polarity potential to the membrane, aiming to electrostatically repel these foulants. When carbonaceous membranes were used as the cathode in an electrochemical filter cell, they showed good resistance to organic fouling at a suitable voltage.264 As a result of the like-charge repulsion between the foulant and the membrane, the flux loss was much lower than that without a potential over the same period. Recently, Xu et al. designed a novel electrocoagulation membrane reactor (ECR-ECM) integrating electrocoagulation and membrane separation in one reactor and attempted to mitigate membrane fouling under electrochemical assitance.265 Because of the coexistence of electrocoagulation, electrostatic repulsion and bubble generation, the fouling layer formed was very loose, which resulted in a decrease in flux decline. This was consistent with the result calculated from the Kozeny–Carman equation. The antifouling mechanisms were also used in non-pressure driven membrane processes based on electrically conductive membranes, such as membrane distillation (MD) and forward osmosis (FO).140,143,266–269 For MD membranes, organic fouling usually results in pore wetting and loss-of-function of hydrophobic membranes. Under electrochemical assistance, this undesirable behavior can be significantly inhibited on the carbonaceous MD membranes due to the electro-repulsive force, which was confirmed by XDLVO theory analysis.269 Additionally, oil fouling was also successfully mitigated during oil/water separation by electrochemically enhanced electrostatic repulsion (Fig. 7a).270,271
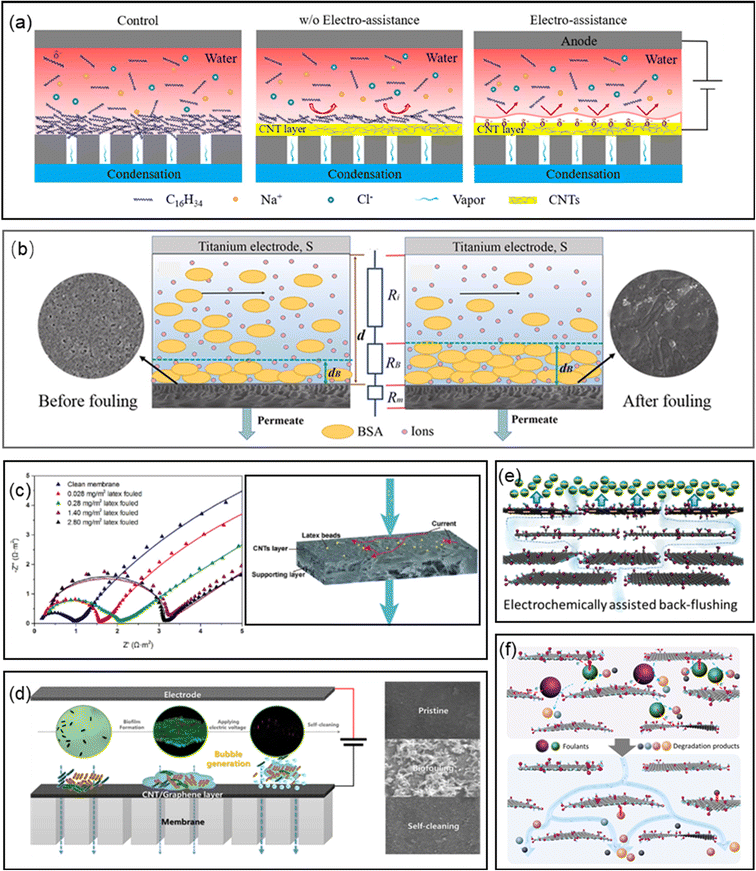 |
| Fig. 7 (a) Electrically enhanced fouling resistance in oil/water separation (reproduced with permission from ref. 270. Copyright 2021, Elsevier). (b) Schematic mechanism of fouling monitoring through linear sweep voltammogram (reproduced with permission from ref. 286. Copyright 2019, Elsevier). (c) Detection of fouling on electrically conductive membranes by electrical impedance spectroscopy (reproduced with permission from ref. 287. Copyright 2020, Elsevier). (d) Self-cleaning of biofouling on electrochemically assisted membrane (reproduced with permission from ref. 289. Copyright 2022, Elsevier). (e) Schematic illustration of electrochemistry-assisted back-flushing (reproduced with permission from ref. 290. Copyright 2019, the American Chemical Society). (f) Schematic illustration of electrochemical cleaning of the rGO/CNT/PVDF membranes (reproduced with permission from ref. 291. Copyright 2020, the American Chemical Society). | |
7.1.2 Improved resistance to biofouling.
Although biofouling is more complex because of cell reproduction, it was found that the flux decrease for an electroconductive membrane was only caused by the deposition of bacteria, rather than bacterial attachment.45,272,273 In contrast, the membranes without potentials failed to achieve a good antifouling performance and their flux recovery after cross-flow rinse. To illustrate the antifouling mechanism under electrochemical assistance, Jiang et al. systematically investigated the antifouling behavior in both capacitor mode and resistor mode.274 The capacitor mode exhibited stronger prevention ability from biomass accumulation than the resistor mode. In capacitor mode, fouling mitigation was dominated by both competitive electrostatic repulsion among the bacteria cells on the cathodic membrane and cell-disruption effect of generated electro-catalyzed reactive oxygen species (ROS). The antifouling ability of the resistor mode arose from the temperature increase near the membrane surface caused by Joule heating effects. The inhibition of biofouling from low surface-charged bacteria could be also achieved by H2O2 production through the electroreduction of oxygen.275,276 The electrochemically produced H2O2 reduced the microbial cell viability and increased cellular permeability, preventing bacterial attachment and ensuring biofilm-free conditions. Additionally, finite element modeling model demonstrated that both electrophoresis and dielectrophoresis are anti-biofouling mechanisms for electroconductive membranes.277 Additionally, the contribution of a low-voltage electric field was evaluated for the prevention of bacterial attachment and cell inactivation.278 It was found that an alternating current (AC) was more effective than a direct current (DC) in terms of both biofouling reduction compared to the cathodic DC and cell inactivation compared to the anodic DC. According to the electrochemical impedance spectroscopy (EIS) analysis, different from the redox reactions in the capacitance mode, the pure resistor behavior resulted in the bacteria detachment and inactivation for bio-fouling mitigation in the resistance mode.
7.1.3 Improved resistance to scaling.
Inorganic fouling (mainly scaling) is one of the major obstacles in the application of RO membranes.279–281 Duan et al. achieved the electrochemical prevention and removal of CaSO4 and CaCO3 mineral scales on an electrically conducting CNT–PA RO membrane, and qualitatively explained the mechanism based on a modified Poisson–Boltzmann equation.81 When the membranes acted as an anode, water splitting led to proton formation, resulting in the dissolution of the deposited CaCO3 crystals. Meanwhile, the CaSO4 scale formation was significantly retarded through the formation of a thick layer of counter-ions along the positively charged membrane surface, which pushed CaSO4 crystal formation away from the membrane surface, allowing the formed crystals to be carried away by cross flow. Besides, CaSO4 and silicate scaling could almost be completely eliminated on a carbonaceous membrane by an energy-efficient electrophoretic mixing method during electrochemically assisted membrane distillation.282
7.2 Electrochemical fouling monitoring of nanocarbonaceous membranes
Fouling monitoring is very important because it can provide a suitable means to achieve flux recovery of membranes.283–285 Compared with the method for monitoring flux changes with a hysteretic nature, some electrochemical analysis methods are alternatives to analyze the fouling formation process timely. For example, when a negatively charged foulant gradually accumulates on the membrane surface, a fouling layer forms and becomes thicker and denser as the separation time increases. The fouling layer contains abundant charged foulant and co-exiting ions and has a lower electric resistance than the feed solution. Consequently, the total resistance between the conductive membrane and counter electrode decreased with an increase in the thickness of the fouling layer. Therefore, the formation and growth of the fouling layer could be monitored by measuring the total resistance with linear sweep voltammetry. This novel and effective online membrane fouling monitoring system was developed based on nanocarbonaceous membranes (Fig. 7b), considering their excellent electrochemical properties.286 The relationship and trends between the permeate flux and membrane resistance can provide a theoretical basis for practical applications for regenerating fouled membranes at an appropriate time. Consequently, the simulation results of the membrane fouling mechanisms, such as monolayer adsorption and pore constriction at either the active or support layer, and intermediate pore blockage at the outer surface, could be confirmed by distinguishable EIS spectra (Fig. 7c).287
7.3 Electrochemical regeneration of nanocarbonaceous membranes
Given that membrane fouling is unavoidable in long-term operation, membrane recovery is very important to maintain the separation performance with minimum deterioration. Besides the conventional physical and chemical methods, electrochemical assistance is considered to be a non-destructive, continuous and renewable approach for in situ membrane self-cleaning.288 The electrochemical effects on the performance regeneration of membranes suggested that the membrane performance was recovered after the filtration of protein, humic acid and dyes under the assistance of electrochemical oxidation, bubble generation and ionic pumping (Fig. 7d–f).172,265,289–291 Besides, the movements of large cations in the CNT core (denoted as ionic pump) were considered to remove the adsorbed foulants in the membrane pores.172 In addition, the hydroxide ions generated from water electrolysis on the membrane surface efficiently dissolved the silicate scaling developed during the MD process, negating the need for chemical cleaning.292 Meanwhile, periodic electrolysis was used to remove/prevent membrane fouling from CaCO3 and yeast suspension occurred on the cathodic membrane.293 The cleaning mechanism was explained by the evolution of gases to form micro-bubbles on the membrane surface, which removed the foulant from the membrane. The same approach was also used to achieve the self-cleaning of biofouling on electroconductive membranes.173 In addition, backwashing under an alternating current enabled a flux recovery of up to 96%.277 Although it was found that the application of a potential did not impact the NF membrane fouling, the flux of the membrane with a negative potential was recoverable after simply rinsing with water, while the membrane with a positive potential was not completely recovered due to the polymerization of the fouling molecules on the membrane surface.261 Therefore, it can be concluded that electrolysis is an alternative approach for the in situ performance regeneration of fouled electrochemical membranes.293
8. Applications of membrane/electrochemistry systems in bioelectrical systems
Membrane bioreactors (MBR), which provide stable and high-quality effluent, can overcome the drawbacks of conventional biological treatment such as sludge loss and large occupation area. However, the performance of the current MBR processes should be further improved to promote their applications. Due to their excellent antifouling and electrochemical activity, nanocarbonaceous membranes have been used in some MBR processes. It was found that a poly(ether-b-amide)/CNT-based membrane could drive the reactions of hydrogen evolution and oxygen reduction, making it a cheaper and greener substitute for platinum-based cathodes in microbial bioelectrochemical systems.294 Water flux and molecular weight cut off measurements indicated that the electrochemically active coating layer did not affect the separation performance of the membrane. By in situ utilization of the generated electricity for fouling control, an antifouling electrochemical membrane bioreactor (EMBR) was developed, in which the generated electric field inhibited the deposition of sludge on the membrane surface by enhancing the electrostatic repulsive force between the membrane and sludge.295,296 The H2O2 produced on the membrane cathode also contributed to the fouling mitigation by the in situ removal of foulants.297 For a CNT hollow fiber membrane-based MBR for the removal of bovine serum albumin (BSA), sodium alginate (SA) and supernatant of anaerobic bioreactor (SAB),141 the cathodic membrane exhibited significant fouling resistance at the optimal voltage of 1.2 V, given that the electrochemically enhanced electrostatic repulsion prevented migration of the negatively charged molecules toward the membrane surface. The removal efficiencies of BSA, SA, and SAB were 92.1%, 87.3% and 56.8%, respectively. All of them were higher than that of the sole membrane. In an anaerobic EMBR (AnEMBR) system, the electro-polarized CNT hollow fiber membranes could promote energy recovery (CH4 production) at low temperature (15–20 °C).142 The Simpson and Shannon indexes indicated that the stability of the microbial community was significantly improved in AnEMBR. At a voltage of 1.2 V (membrane as the cathode), the EMBR system produced more CH4 because both Methanomicrobia and H2-utilizing methanogens were more than that in the conventional AnMBR with polymeric hollow fiber membranes. Meanwhile, the AnEMBR exhibited a better antifouling and COD removal performance compared with the two control AnMBRs during an almost 100 day operation period.
When carbonaceous membranes work as the anode, electrochemical oxidation can be used to improve the water quality and mitigate membrane fouling in the AnEMBR system.156,298 The COD and the concentration of NH4+-N in the effluent from this AnEMBR system were lower than 40 and 3 mg L−1, respectively, which were lower than that in the effluent from the conventional AnMBR. The hydraulic cleaning times of the fouled membranes was shortened by 60–75% compared to the two control AnMBR systems during a 60 day operation. Moreover, the permeate flux could be recovered from ∼390 to ∼2020 LMH per bar (near the pure water flux of 2200 LMH per bar) after a short-time electrooxidation treatment.
Recently, Yang et al. reported a novel electro-Fenton membrane bioreactor (EFMBR) with an enhanced treatment performance (Fig. 8).299,300 The in situ produced H2O2 from the electroreduction of dissolved O2 through the two-electron pathway was transformed into HO˙, which was catalyzed by the pre-loaded Fe2+ on the CNT hollow fiber membrane. More importantly, the Fe3+/Fe2+ cycle was realized quickly on the cathodic membrane, avoiding ferrous ion loss and extra-addition. During an over 100 day operation, this EFMBR system had removal efficiencies of 93% for COD and 88% for NH4+-N at an HRT of 8 h. More importantly, the membranes were structurally stable, as evidenced by the fact that they were the same as the fresh CNT hollow fiber membrane after a 100 day operation in EFMBR. Meanwhile, the permeate flux of the fouled membrane was completely regenerated by applying a low potential of 0.8 V on the cathodic membrane for a short time of 15 min.
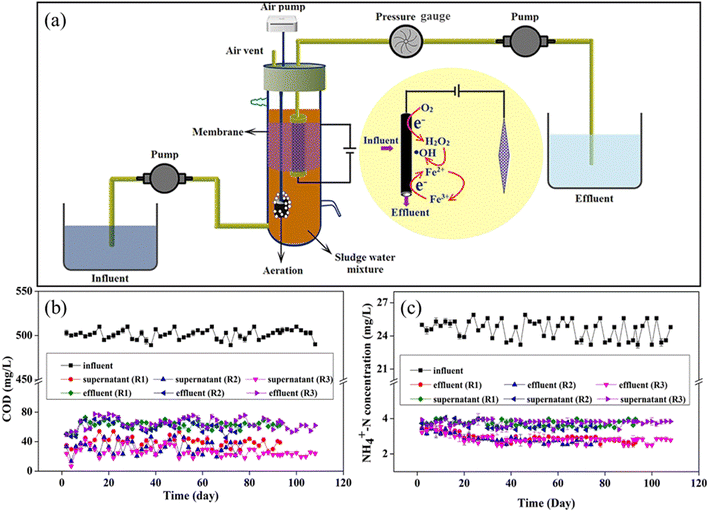 |
| Fig. 8 Schematic diagram (a), removal efficiency of COD (b) and NH4+-N (c) of EFMBR (reproduced with permission from ref. 299. Copyright 2020, the American Chemical Society). | |
Microbial fuel cells (MFCs) can achieve green and sustainable energy conversion by integrating bacterial biofilms in electrochemistry systems to produce electricity from organic waste. Zuo et al. compared three types of electroconductive membranes (nitrogen-doped CNT (N-CNT) membrane, Pt-coated CNT (Pt-CNT) membrane and pristine CNT membrane) as the air cathode and filtration materials of MFCs.301 The N-CNT membrane-based MFC achieved the best effluent quality and power generation, which was mainly attributed to its special morphology and rich N-functional groups. The high nitrogen content and high specific surface area enhanced the O2 reduction by increasing the mass transfer in the micropores and improving the proton transfer from the anode to cathode. Moreover, a separate experiment demonstrated that the cathodic membrane showed high antifouling behavior under high current operation.
9. Outlook and perspectives
Nanocarbonaceous membranes have excellent permeability and selectivity and can provide a platform to integrate membrane separation and electrochemistry to enable promising technology for water purification and wastewater treatment, arising from their excellent reactive, antifouling, and self-cleaning properties. The electrochemically assisted separation processes extend the membrane functionalities beyond the single pore-exclusion separation. The enhancements in pollutant and salt removal demonstrate their potential applications in producing potable and clean water from wastewater and saline water. The convection-enhanced mass transfer promotes rapid organic micropollutant degradation through electrochemical reactions (e.g., electrooxidation, electroreduction, and electro-Fenton process) during the flow-through membrane processes. Additionally, the inevitable membrane fouling can be significantly mitigated by the electrostatic repulsion and electrokinetic phenomena (e.g., electroosmosis, electrophoresis, and electromigration). However, before the practical application of nanocarbonaceous membranes and electrochemically assisted membrane technology, there are some issues that should be addressed.
Firstly, it is still a great challenge to achieve the low-cost and high-throughput preparation of nanocarbonaceous membranes with an ideal structure, for example, large-area thin aligned CNT membrane and large-area defect-free nanoporous graphene membrane. In addition, the current nanocarbonaceous membranes usually have low mechanical strength.
Secondly, the performance and energy consumption of nanocarbonaceous membranes and electrochemically assisted membrane processes for the treatment of real water or wastewater should be systematically investigated. The recent studies were usually performed using simulated wastewater with a specific and simple component. However, the constituents of natural water and real wastewater are complex, and their quality (e.g., ion strength, total organic carbon and pH) can significantly affect the electrochemical reactions and electrokinetic phenomena. The electrochemical adsorption, oxidation and reduction of non-contaminants will compete with that of contaminants, which can greatly lower the treatment efficiency and increase the energy consumption. Moreover, the metal ions in water can be easily electrochemically reduced by the membrane cathode, whose pores can be blocked by the resultant metal crystals. This will weaken the membrane performance and electrochemical reactivity.
Finally, the structural stability of nanocarbonaceous membranes in water should be further improved. Nanocarbonaceous membranes are usually present in the form of a separation layer on a substrate and generally need to be cross-linked by organic polymers. However, although carbon nanomaterials are chemically stable, the cross-linker may age in water. Thus, to date, no nanocarbonaceous membrane has been reported to be stable in water for several years. Additionally, during electrochemical oxidation or electro-Fenton process, the generated highly reactive oxygen species such as HO˙ can also oxidize the membranes, which can result in their performance decline and structural damage.
Efforts should be also made in the future to design large-scale membrane modules that allow high-efficiency membrane filtration and electrochemical assistance. It is believed that the advancement of carbon-nanomaterial-based membranes can also greatly contribute to many other fields, for example, energy production, synthesis and purification of chemicals. The future looks extremely promising for these membranes and electrochemically assisted membrane technology considering their many advantages over conventional membrane processes.
Author contributions
The manuscript was written through contributions of all authors. All authors have given approval to the final version of the manuscript.
Conflicts of interest
The authors declare no competing financial interest.
Acknowledgements
This work was supported by the National Key Research and Development Program of China (2020YFA0211001).
References
- D. Jassby, T. Y. Cath and H. Buisson, The role of nanotechnology in industrial water treatment, Nat. Nanotechnol., 2018, 13, 670–672 CrossRef CAS PubMed.
- A. G. Fane, R. Wang and M. X. Hu, Synthetic Membranes for Water Purification: Status and Future, Angew. Chem., Int. Ed., 2015, 54, 3368–3386 CrossRef CAS PubMed.
- K. Goh, H. E. Karahan, L. Wei, T. H. Bae, A. G. Fane, R. Wang and Y. Chen, Carbon nanomaterials for advancing separation membranes: A strategic perspective, Carbon, 2016, 109, 694–710 CrossRef CAS.
- M. T. M. Pendergast and E. M. V. Hoek, A review of water treatment membrane nanotechnologies, Energy Environ. Sci., 2011, 4, 1946–1971 RSC.
- J. Caro, Hierarchy in inorganic membranes, Chem. Soc. Rev., 2016, 45, 3468–3478 RSC.
- X. B. Ke, H. Y. Zhu, X. P. Gao, J. W. Liu and Z. F. Zheng, High-Performance Ceramic Membranes with a Separation Layer of Metal Oxide Nanofibers, Adv. Mater., 2007, 19, 785–790 CrossRef CAS.
- A. Anand, B. Unnikrishnan, J. Y. Mao, H. J. Lin and C. C. Huang, Graphene-based nanofiltration membranes for improving salt rejection, water flux and antifouling–A review, Desalination, 2018, 429, 119–133 CrossRef CAS.
- V. Sharma, G. Borkute and S. P. Gumfekar, Biomimetic nanofiltration membranes: Critical review of materials, structures, and applications to water purification, Chem. Eng. J., 2022, 433, 133823 CrossRef CAS.
- M. Elimelech and W. A. Phillip, The Future of Seawater Desalination: Energy, Technology, and the Environment, Science, 2011, 333, 712–717 CrossRef CAS PubMed.
- B. D. Coday, B. G. M. Yaffe, P. Xu and T. Y. Cath, Rejection of Trace Organic Compounds by Forward Osmosis Membranes: A Literature Review, Environ. Sci. Technol., 2014, 48, 3612–3624 CrossRef CAS.
- S. F. Zhao, L. D. Zou, C. Y. Y. Tang and D. Mulcahy, Recent developments in forward osmosis: Opportunities and challenges, J. Membr. Sci., 2012, 396, 1–21 CrossRef CAS.
- L. M. Camacho, L. Dumée, J. H. Zhang, J. D. Li, M. Duke, J. Gomez and S. Gray, Advances in Membrane Distillation for Water Desalination and Purification Applications, Water, 2013, 5, 94 CrossRef.
- X. B. Zhu and D. Jassby, Electroactive Membranes for Water Treatment: Enhanced Treatment Functionalities, Energy Considerations, and Future Challenges, Acc. Chem. Res., 2019, 52, 1177–1186 CrossRef CAS.
- K. P. Katuri, S. Kalathil, A. Ragab, B. Bian, M. F. Alqahtani, D. Pant and P. E. Saikaly, Dual-function electrocatalytic and macroporous hollow-fiber cathode for converting waste streams to valuable resources using microbial electrochemical systems, Adv. Mater., 2018, 30, 1707072 CrossRef.
- Y. B. Liu, G. D. Gao and C. D. Vecitis, Prospects of an Electroactive Carbon Nanotube Membrane towards Environmental Applications, Acc. Chem. Res., 2020, 53, 2892–2902 CrossRef CAS PubMed.
- G. D. Gao and C. D. Vecitis, Doped Carbon Nanotube Networks for Electrochemical Filtration of Aqueous Phenol: Electrolyte Precipitation and Phenol Polymerization, ACS Appl. Mater. Interfaces, 2012, 4, 1478–1489 CrossRef CAS.
- W. L. Jiang, X. Xia, J. L. Han, Y. C. Ding, R. H. Muhammad and A. J. Wang, Graphene modified electro-Fenton catalytic membrane for in-situ degradation of antibiotic florfenicol, Environ. Sci. Technol., 2018, 52, 9972–9982 CrossRef CAS PubMed.
- C. M. Werner, K. P. Katuri, A. R. Hari, W. Chen, Z. P. Lai, B. E. Logan, G. L. Amy and P. E. Saikaly, Graphene-Coated Hollow Fiber Membrane as the Cathode in Anaerobic Electrochemical Membrane Bioreactors-Effect of Configuration and Applied Voltage on Performance and Membrane Fouling, Environ. Sci. Technol., 2016, 50, 4439–4447 CrossRef CAS PubMed.
- Y. B. Liu, P. Wu, F. Q. Liu, F. Li, X. Q. An, J. S. Liu, Z. W. Wang, C. S. Shen and W. Sand, Electroactive Modified Carbon Nanotube Filter for Simultaneous Detoxification and Sequestration of Sb(III), Environ. Sci. Technol., 2019, 53, 1527–1535 CrossRef CAS PubMed.
- G. L. Wei, X. Quan, S. Chen and H. T. Yu, Superpermeable Atomic-Thin Graphene Membranes with High Selectivity, ACS Nano, 2017, 11, 1920–1926 CrossRef CAS.
- G. L. Wei, X. Quan, C. Li, S. Chen and H. T. Yu, Direct growth of ultra-permeable molecularly thin porous graphene membranes for water treatment, Environ. Sci.: Nano, 2018, 5, 3004–3010 RSC.
- B. Q. Yuan, M. X. Wang, B. Wang, F. L. Yang, X. Quan, C. Y. Y. Tang and Y. C. Dong, Cross-linked Graphene Oxide Framework Membranes with Robust Nano-Channels for Enhanced Sieving Ability, Environ. Sci. Technol., 2020, 54, 15442–15453 CrossRef CAS PubMed.
- S. O. Ganiyu, E. D. van Hullebusch, M. Cretin, G. Esposito and M. A. Oturan, Coupling of membrane filtration and advanced oxidation processes for removal of pharmaceutical residues: A critical review, Sep. Purif. Technol., 2015, 156, 891–914 CrossRef CAS.
- C. Trellu, B. P. Chaplin, C. Coetsier, R. Esmilaire, S. Cerneaux, C. Causserand and M. Cretin, Electro-oxidation of organic pollutants by reactive electrochemical membranes, Chemosphere, 2018, 208, 159–175 CrossRef CAS PubMed.
- M. Sun, X. X. Wang, L. R. Winter, Y. M. Zhao, W. Ma, T. Hedtke, J. H. Kim and M. Elimelech, Electrified Membranes for Water Treatment Applications, ACS ES&T Engg, 2021, 1, 725–752 Search PubMed.
- Z. X. Zhang, G. H. Huang, Y. P. Li, X. J. Chen, Y. Yao, S. J. Ren, M. N. Li, Y. W. Wu and C. J. An, Electrically conductive inorganic membranes: A review on principles, characteristics and applications, Chem. Eng. J., 2022, 427, 131987 CrossRef CAS.
- J. J. Patil, A. Jana, B. A. Getachew, D. S. Bergsman, Z. Gariepy, B. D. Smith, Z. M. Lu and J. C. Grossman, Conductive carbonaceous membranes: recent progress and future opportunities, J. Mater. Chem. A, 2021, 9, 3270–3289 RSC.
- F. Ahmed, B. S. Lalia, V. Kochkodan, N. Hilal and R. Hashaikeh, Electrically conductive polymeric membranes for fouling prevention and detection: A review, Desalination, 2016, 391, 1–15 CrossRef CAS.
- Y. H. Mo, L. Zhang, X. Zhao, J. X. Li and L. Wang, A critical review on classifications, characteristics, and applications of electrically conductive membranes for toxic pollutant removal from water: Comparison between composite and inorganic electrically conductive membranes, J. Hazard. Mater., 2022, 436, 129162 CrossRef CAS PubMed.
-
Z. W. Wang, K. H. Choo, C. Y. Y. Tang and T. Waite, Electrochemical Membrane Technology for Water and Wastewater Treatment, Elsevier, 2022 Search PubMed.
- Q. Zhang, S. Chen, X. F. Fan, H. G. Zhang, H. T. Yu and X. Quan, A multifunctional graphene-based nanofiltration membrane under photo-assistance for enhanced water treatment based on layer-by-layer sieving, Appl. Catal., B, 2018, 224, 204–213 CrossRef CAS.
- X. T. Wang, G. L. Wang, S. Chen, X. F. Fan, X. Quan and H. T. Yu, Integration of membrane filtration and photoelectrocatalysis on g-C3N4/CNTs/Al2O3 membrane with visible-light response for enhanced water treatment, J. Membr. Sci., 2017, 541, 153–161 CrossRef CAS.
- J. L. Zhang, H. T. Yu, X. Quan, S. Chen and Y. B. Zhang, Ceramic membrane separation coupled with catalytic ozonation for tertiary treatment of dyestuff wastewater in a pilot-scale study, Chem. Eng. J., 2016, 301, 19–26 CrossRef CAS.
- H. R. Zhang, Y. H. Wan, J. Q. Luo and S. B. Darling, Drawing on membrane photocatalysis for fouling mitigation, ACS Appl. Mater. Interfaces, 2021, 13, 14844–14865 CrossRef CAS PubMed.
- S. C. Wu, P. Cheng, J. J. Han, Y. Chen, X. Yan, X. J. Guo and W. Z. Lang, Construction of two-dimensional Ag/WS2 hybrid membranes with self-cleaning ability by photocatalysis for efficient water filtration, J. Membr. Sci., 2022, 641, 119865 CrossRef CAS.
- H. R. Zhang, J. X. Zhang, J. Q. Luo and Y. H. Wan, A novel paradigm of photocatalytic cleaning for membrane fouling removal, J. Membr. Sci., 2022, 641, 119859 CrossRef CAS.
- A. Rahimpour, S. S. Madaeni, A. H. Taheri and Y. Mansourpanah, Coupling TiO2 nanoparticles with UV irradiation for modification of polyethersulfone ultrafiltration membranes, J. Membr. Sci., 2008, 313, 158–169 CrossRef CAS.
- H. X. Zhao, S. Chen, X. Quan, H. T. Yu and H. M. Zhao, Integration of microfiltration and visible-light-driven photocatalysis on g-C3N4 nanosheet/reduced graphene oxide membrane for enhanced water treatment, Appl. Catal., B, 2016, 194, 134–140 CrossRef CAS.
- S. Laohaprapanon, A. D. Vanderlipe, B. T. Doma Jr and S.-J. You, Self-cleaning and antifouling properties of plasma-grafted poly(vinylidene fluoride) membrane coated with ZnO for water treatment, J. Taiwan Inst. Chem. Eng., 2017, 70, 15–22 CrossRef CAS.
- Y. Q. Zhu, S. Chen, X. Quan, Y. B. Zhang, C. Gao and Y. J. Feng, Hierarchical porous ceramic membrane with energetic ozonation capability for enhancing water treatment, J. Membr. Sci., 2013, 431, 197–204 CrossRef CAS.
- R. Oulton, J. P. Haase, S. Kaalberg, C. T. Redmond, M. J. Nalbandian and D. M. Cwiertny, Hydroxyl radical formation during ozonation of multiwalled carbon nanotubes: performance optimization and demonstration of a reactive CNT filter, Environ. Sci. Technol., 2015, 49, 3687–3697 CrossRef CAS PubMed.
- Y. Guo, B. B. Xu and F. Qi, A novel ceramic membrane coated with MnO2–Co3O4 nanoparticles catalytic ozonation for benzophenone-3 degradation in aqueous solution: fabrication, characterization and performance, Chem. Eng. J., 2016, 287, 381–389 CrossRef CAS.
- Y. Q. Zhu, X. Quan, F. J. Chen, X. F. Fan and Y. J. Feng, CeO2–TiO2 coated ceramic membrane with catalytic ozonation capability for treatment of tetracycline in drinking water, Sci. Adv. Mater., 2012, 4, 1191–1199 CrossRef CAS.
- Y. M. Zhao, M. Sun, L. R. Winter, S. H. Lin, Z. W. Wang, J. C. Crittenden and J. Ma, Emerging challenges and opportunities for electrified membranes to enhance water treatment, Environ. Sci. Technol., 2022, 56, 3832–3835 CrossRef CAS.
- C.-F. de Lannoy, D. Jassby, K. Gloe, A. D. Gordon and M. R. Wiesner, Aquatic biofouling prevention by electrically charged nanocomposite polymer thin film membranes, Environ. Sci. Technol., 2013, 47, 2760–2768 CrossRef CAS PubMed.
- P. Bhol, S. Yadav, A. Altaee, M. Saxena, P. K. Misra and A. K. Samal, Graphene-Based Membranes for Water and Wastewater Treatment: A Review, ACS Appl. Nano Mater., 2021, 4, 3274–3293 CrossRef CAS.
- Y. Li, N. Li, Y. Xia, S. Yuan and X. W. Zhang, Tailoring the physicochemical and geometric properties of two-dimensional graphene membranes for aqueous separation, Desalination, 2022, 530, 115621 CrossRef CAS.
- P. Z. Sun, K. L. Wang and H. W. Zhu, Recent Developments in Graphene-based membranes: structure, mass-transport mechanism and potential applications, Adv. Mater., 2016, 28, 2287–2310 CrossRef CAS PubMed.
- G. P. Liu, W. Q. Jin and N. P. Xu, Graphene-based membranes, Chem. Soc. Rev., 2015, 44, 5016–5030 RSC.
- J. Lee, S. Jeong and Z. W. Liu, Progress and challenges of carbon nanotube membrane in water treatment, Crit. Rev. Environ. Sci. Technol., 2016, 46, 999–1046 CrossRef CAS.
- K. Murata, K. Mitsuoka, T. Hirai, T. Walz, P. Agre, J. B. Heymann, A. Engel and Y. Fujiyoshi, Structural determinants of water permeation through aquaporin-1, Nature, 2000, 407, 599–605 CrossRef CAS.
- H. Sui, B. Han, J. K. Lee, P. Walian and B. K. Jap, Structural basis of water-specific transport through the AQP1 water channel, Nature, 2001, 414, 872–878 CrossRef CAS PubMed.
- F. Fornasiero, H. G. Park, J. K. Holt, M. Stadermann, C. P. Grigoropoulos, A. Noy and O. Bakajin, Ion exclusion by sub-2-nm carbon nanotube pores, Proc. Natl. Acad. Sci. U. S. A., 2008, 105, 17250–17255 CrossRef CAS.
- G. Hummer and A. Berezhkovskii, Single-File Transport of Water Molecules through a Carbon Nanotube, Phys. Rev. Lett., 2002, 89, 064503 CrossRef.
- G. Hummer, J. C. Rasaiah and J. P. Noworyta, Water conduction through the hydrophobic channel of a carbon nanotube, Nature, 2001, 414, 188–190 CrossRef CAS PubMed.
- A. Kalra, S. Garde and G. Hummer, Osmotic water transport through carbon nanotube membranes, Proc. Natl. Acad. Sci. U. S. A., 2003, 100, 10175–10180 CrossRef CAS PubMed.
- B. J. Hinds, N. Chopra, T. Rantell, R. Andrews, V. Gavalas and L. G. Bachas, Aligned multiwalled carbon nanotube membranes, Science, 2004, 303, 62–65 CrossRef CAS PubMed.
- M. Majumder, N. Chopra, R. Andrews and B. J. Hinds, Enhanced flow in carbon nanotubes, Nature, 2005, 438, 44–44 CrossRef CAS PubMed.
- M. Majumder, N. Chopra and B. J. Hinds, Effect of tip functionalization on transport through vertically oriented carbon nanotube membranes, J. Am. Chem. Soc., 2005, 127, 9062–9070 CrossRef CAS PubMed.
- J. K. Holt, H. G. Park, Y. Wang, M. Stadermann, A. B. Artyukhin, C. P. Grigoropoulos, A. Noy and O. Bakajin, Fast Mass transport through sub-2-nanometer carbon nanotubes, Science, 2006, 312, 1034–1037 CrossRef CAS PubMed.
- M. Majumder, N. Chopra and B. J. Hinds, Mass Transport through carbon nanotube membranes in three different regimes: ionic diffusion and gas and liquid flow, ACS Nano, 2011, 5, 3867–3877 CrossRef CAS PubMed.
- S. Joseph and N. Aluru, Why are carbon nanotubes fast transporters of water?, Nano Lett., 2008, 8, 452–458 CrossRef CAS PubMed.
- G. L. Wei, S. Chen, X. F. Fan, X. Quan and H. T. Yu, Carbon nanotube hollow fiber membranes: High-throughput fabrication, structural control and electrochemically improved selectivity, J. Membr. Sci., 2015, 493, 97–105 CrossRef CAS.
- K. Sears, L. Dumée, J. Schütz, M. She, C. Huynh, S. Hawkins, M. Duke and S. Gray, Recent developments in carbon nanotube membranes for water purification and gas separation, Materials, 2010, 3, 127–149 CrossRef CAS.
- P. Gunawan, C. Guan, X. Song, Q. Zhang, S. S. J. Leong, C. Tang, Y. Chen, M. B. Chan-Park, M. W. Chang, K. Wang and R. Xu, Hollow fiber membrane decorated with Ag/MWNTs: toward effective water disinfection and biofouling control, ACS Nano, 2011, 5, 10033–10040 CrossRef CAS PubMed.
- H. Bai, X. Zan, L. Zhang and D. D. Sun, Multi-functional CNT/ZnO/TiO2 nanocomposite membrane for concurrent filtration and photocatalytic degradation, Sep. Purif. Technol., 2015, 156, 922–930 CrossRef CAS.
- W. Mi, Y. S. Lin and Y. Li, Vertically aligned carbon nanotube membranes on macroporous alumina supports, J. Membr. Sci., 2007, 304, 1–7 CrossRef CAS.
- Y. Baek, C. Kim, D. K. Seo, T. Kim, J. S. Lee, Y. H. Kim, K. H. Ahn, S. S. Bae, S. C. Lee, J. Lim, K. Lee and J. Yoon, High performance and antifouling vertically aligned carbon nanotube membrane for water purification, J. Membr. Sci., 2014, 460, 171–177 CrossRef CAS.
- M. Yu, H. H. Funke, J. L. Falconer and R. D. Noble, High Density, Vertically-aligned carbon nanotube membranes, Nano Lett., 2009, 9, 225–229 CrossRef CAS PubMed.
- B. Lee, Y. Baek, M. Lee, D. H. Jeong, H. H. Lee, J. Yoon and Y. H. Kim, A carbon nanotube wall membrane for water treatment, Nat. Commun., 2015, 6, 7109 CrossRef CAS PubMed.
- L. Zhang, B. Zhao, C. Jiang, J. Yang and G. Zheng, Preparation and transport performances of high-density, aligned carbon nanotube membranes, Nanoscale Res. Lett., 2015, 10, 266 CrossRef PubMed.
- K. Lee and H. Park, The most densified vertically-aligned carbon nanotube membranes and their normalized water permeability and high pressure durability, J. Membr. Sci., 2016, 501, 144–151 CrossRef CAS.
- M. J. Larocque, D. R. Latulippe and C. de Lannoy, Formation of electrically conductive hollow fiber membranes via crossflow deposition of carbon nanotubes-Addressing the conductivity/permeability trade-off, J. Membr. Sci., 2021, 620, 118859 CrossRef CAS.
- G. L. Wei, H. T. Yu, X. Quan, S. Chen, H. M. Zhao and X. F. Fan, Constructing all carbon nanotube hollow fiber membranes with improved performance in separation and antifouling for water treatment, Environ. Sci. Technol., 2014, 48, 8062–8068 CrossRef CAS PubMed.
- A. Huang, F. Liu, Z. Cui, H. Wang, X. Song, L. Geng, H. Wang and X. Peng, Novel PTFE/CNT composite nanofiber membranes with enhanced mechanical, crystalline, conductive, and dielectric properties fabricated by emulsion electrospinning and sintering, Compos. Sci. Technol., 2021, 214, 108980 CrossRef CAS.
- X. Fan, H. Zhao, Y. Liu, X. Quan, H. Yu and S. Chen, Enhanced permeability, selectivity, and antifouling ability of cnts/al2o3 membrane under electrochemical assistance, Environ. Sci. Technol., 2015, 49, 2293–2300 CrossRef CAS PubMed.
- X. Fan, H. Zhao, X. Quan, Y. Liu and S. Chen, Nanocarbon-based membrane filtration integrated with electric field driving for effective membrane fouling mitigation, Water Res., 2016, 88, 285–292 CrossRef CAS PubMed.
- C.-F. de Lannoy, D. Jassby, D. D. Davis and M. R. Wiesner, A highly electrically conductive polymer–multiwalled carbon nanotube nanocomposite membrane, J. Membr. Sci., 2012, 415, 718–724 CrossRef.
- W. Duan, G. Chen, C. Chen, R. Sanghvi, A. Iddya, S. Walker, H. Liu, A. Ronen and D. Jassby, Electrochemical removal of hexavalent chromium using electrically conducting carbon nanotube/polymer composite ultrafiltration membranes, J. Membr. Sci., 2017, 531, 160–171 CrossRef CAS.
- A. V. Dudchenko, J. Rolf, K. Russell, W. Duan and D. Jassby, Organic fouling inhibition on electrically conducting carbon nanotube–polyvinyl alcohol composite ultrafiltration membranes, J. Membr. Sci., 2014, 468, 1–10 CrossRef CAS.
- W. Duan, A. Dudchenko, E. Mende, C. Flyer, X. Zhu and D. Jassby, Electrochemical mineral scale prevention and removal on electrically conducting carbon nanotube-polyamide reverse osmosis membranes, Environ. Sci.: Processes Impacts, 2014, 16, 1300–1308 RSC.
- A. K. Geim and K. S. Novoselov, The Rise of Graphene, Nat. Mater., 2007, 6, 183–191 CrossRef CAS PubMed.
- K. S. Novoselov, A. K. Geim, S. V. Morozov, D. Jiang, Y. Zhang, S. V. Dubonos, I. V. Grigorieva and A. A. Firsov, Electric field effect in atomically thin carbon films, Science, 2004, 306, 666–669 CrossRef CAS PubMed.
- Q. Zhang, S. Ghosh, S. Samitsu, X. S. Peng and L. Ichinose, Ultrathin freestanding nanoporous membranes prepared from polystyrene nanoparticles, J. Mater. Chem., 2011, 21, 1684–1688 RSC.
- K. Celebi, J. Buchheim, R. M. Wyss, A. Droudian, P. Gasser, I. Shorubalko, J. Kye, C. Lee and H. G. Park, Ultimate permeation across atomically thin porous graphene, Science, 2014, 344, 289–292 CrossRef CAS PubMed.
- S. S. Xu, L. P. Zhang, B. Wang and R. S. Ruoff, Chemical vapor deposition of graphene on thin-metal films, Cell Rep. Phys. Sci., 2021, 2, 100410 CrossRef CAS.
- C. Cheng, S. A. Iyengar and R. Karnik, Molecular size-dependent subcontinuum solvent permeation and ultrafast nanofiltration across nanoporous graphene membranes, Nat. Nanotechnol., 2021, 16, 989–995 CrossRef CAS PubMed.
- S. P. Surwade, S. N. Smirnov, I. V. Vlassiouk, R. R. Unocic, G. M. Veith, S. Dai and S. M. Mahurin, Water desalination using nanoporous single-layer graphene, Nat. Nanotechnol., 2015, 10, 459–464 CrossRef CAS PubMed.
- L. Shen, Q. Shi, S. P. Zhang, J. Gao, D. C. Cheng, M. Yi, R. Y. Song, L. D. Wang, J. W. Jiang, R. Karnik and S. Zhang, Highly porous nanofiber-supported monolayer graphene membranes for ultrafast organic solvent nanofiltration, Sci. Adv., 2021, 7, eabg6263 CrossRef CAS PubMed.
- Y. B. Yang, X. D. Yang, L. Liang, Y. Y. Gao, H. Y. Cheng, X. M. Li, M. C. Zou, R. Z. Ma, Q. Yuan and X. F. Duan, Large-area graphene-nanomesh/carbon-nanotube hybrid membranes for ionic and molecular nanofiltration, Science, 2019, 364, 1057–1062 CrossRef CAS PubMed.
- K. Huang, G. P. Liu, Y. Y. Lou, Z. Y. Dong, J. Shen and W. Q. Jin, A graphene oxide membrane with highly selective molecular separation of aqueous organic solution, Angew. Chem., Int. Ed., 2014, 53, 6929–6932 CrossRef CAS PubMed.
- R. K. Joshi, P. Carbone, F. C. Wang, V. G. Kravets, Y. Su, I. V. Grigorieva, H. A. Wu, A. K. Geim and R. R. Nair, Precise and ultrafast molecular sieving through graphene oxide membranes, Science, 2014, 343, 752–754 CrossRef CAS PubMed.
- L. Huang, Y. R. Li, Q. Q. Zhou, W. J. Yuan and G. Q. Shi, Graphene oxide membranes with tunable semipermeability in organic solvents, Adv. Mater., 2015, 27, 3797–3802 CrossRef CAS PubMed.
- N. F. D. Aba, J. Y. Chong, B. Wang, C. Mattevi and K. Li, Graphene oxide membranes on ceramic hollow fibers-microstructural stability and nanofiltration performance, J. Membr. Sci., 2015, 484, 87–94 CrossRef CAS.
- D. A. Dikin, S. Stankovich, E. J. Zimney, R. D. Piner, G. H. Dommett, G. Evmenenko, S. T. Nguyen and R. S. Ruoff, Preparation and characterization of graphene oxide paper, Nature, 2007, 448, 457–460 CrossRef CAS PubMed.
- X. B. Cao, D. P. Qi, S. Y. Yin, J. Bu, F. J. Li, C. F. Goh, S. Zhang and X. D. Chen, Ambient fabrication of large-area graphene films via a synchronous reduction and assembly strategy, Adv. Mater., 2013, 25, 2957–2962 CrossRef CAS PubMed.
- C. M. Chen, Q. H. Yang, Y. G. Yang, W. Lv, Y. F. Wen, P.-X. Hou, M. Z. Wang and H.-M. Cheng, Self-assembled free-standing graphite oxide membrane, Adv. Mater., 2009, 21, 3007–3011 CrossRef CAS.
- W. Lv, Z. X. Xia, S. D. Wu, Y. Tao, F.-M. Jin, B. H. Li, H. D. Du, Z.-P. Zhu, Q.-H. Yang and F. Y. Kang, Conductive graphene-based macroscopic membrane self-assembled at a liquid-air interface, J. Mater. Chem., 2011, 21, 3359–3364 RSC.
- R. R. Nair, H. A. Wu, P. N. Jayaram, I. V. Grigorieva and A. K. Geim, Unimpeded permeation of water through helium-leak-tight graphene-based membranes, Science, 2012, 335, 442–445 CrossRef CAS PubMed.
- Z. Liu, Z. Ma, B. Qian, A. Y. H. Chan, X. Wang, Y. Liu and J. H. Xin, A Facile and Scalable Method of Fabrication of Large-Area Ultrathin Graphene Oxide Nanofiltration Membrane, ACS Nano, 2021, 15, 15294–15305 CrossRef CAS PubMed.
- D. W. Boukhvalov, M. I. Katsnelson and Y.-W. Son, Origin of anomalous water permeation through graphene oxide membrane, Nano Lett., 2013, 13, 3930–3935 CrossRef CAS PubMed.
- S. X. Zheng, Q. S. Tu, J. J. Urban, S. F. Li and B. X. Mi, Swelling of graphene oxide membranes in aqueous solution: characterization of interlayer spacing and insight into water transport mechanisms, ACS Nano, 2017, 11, 6440–6450 CrossRef CAS PubMed.
- W. B. Li, W. F. Wu and Z. J. Li, Controlling interlayer spacing of graphene oxide membranes by external pressure regulation, ACS Nano, 2018, 12, 9309–9317 CrossRef CAS PubMed.
- J. Abraham, K. S. Vasu, C. D. Williams, K. Gopinadhan, Y. Su, C. T. Cherian, J. Dix, E. Prestat, S. J. Haigh, I. V. Grigorieva, P. Carbone, A. K. Geim and R. R. Nair, Tunable sieving of ions using graphene oxide membranes, Nat. Nanotechnol., 2017, 12, 546–551 CrossRef CAS PubMed.
- M. Hu and B. X. Mi, Enabling graphene oxide nanosheets as water separation membranes, Environ. Sci. Technol., 2013, 47, 3715–3723 CrossRef CAS PubMed.
- T. Liu, L. Tian, N. Graham, B. Yang, W. Z. Yu and K. N. Sun, Regulating the Interlayer Spacing of Graphene Oxide Membranes and Enhancing their Stability by Use of PACl, Environ. Sci. Technol., 2019, 53, 11949–11959 CrossRef CAS PubMed.
- H. Gao, Z. Guo, X. Li, Y. Chen, C. Wang, N. Wang and C. Xiao, Crosslinked graphene oxide membranes (CGOM) and their asymmetric permeation behaviors, J. Membr. Sci., 2022, 644, 120158 CrossRef CAS.
- X. Chen, E. Deng, X. Lin, A. Manoj Tandel, D. Rub, L. Zhu, L. Huang and H. Lin, Engineering hierarchical nanochannels in graphene oxide membranes by etching and polydopamine intercalation for highly efficient dye recovery, Chem. Eng. J., 2022, 433, 133593 CrossRef CAS.
- L. Chen, G. S. Shi, J. Shen, B. Q. Peng, B. W. Zhang, Y. Z. Wang, F. G. Bian, J. J. Wang, D. Y. Li, Z. Qian, G. Xu, G. P. Liu, J. R. Zeng, L. J. Zhang, Y. Z. Yang, G. Q. Zhou, M. H. Wu, W. Q. Jin, J. Y. Li and H. P. Fang, Ion sieving in graphene oxide membranes via cationic control of interlayer spacing, Nature, 2017, 550, 380–383 CrossRef CAS PubMed.
- X. B. Lv, R. Xie, J. Y. Ji, Z. Liu, X. Y. Wen, L. Y. Liu, J. Q. Hu, X. J. Ju, W. Wang and L. Y. Chu, A novel strategy to fabricate cation-cross-linked graphene oxide membrane with high aqueous stability and high separation performance, ACS Appl. Mater. Interfaces, 2020, 12, 56269–56280 CrossRef CAS PubMed.
- R. Yang, Y. Fan, R. Yu, F. Dai, J. Lan, Z. Wang, J. Chen and L. Chen, Robust reduced graphene oxide membranes with high water permeance enhanced by K+ modification, J. Membr. Sci., 2021, 635, 119437 CrossRef CAS.
- S. Yuan, Y. Li, Y. Xia, C. Selomulya and X. Zhang, Stable cation-controlled reduced graphene oxide membranes for improved NaCl rejection, J. Membr. Sci., 2021, 621, 118995 CrossRef CAS.
- H. Y. Liu, H. T. Wang and X. W. Zhang, Facile fabrication of freestanding ultrathin reduced graphene oxide membranes for water purification, Adv. Mater., 2015, 27, 249–254 CrossRef CAS PubMed.
- X. T. Fan, C. B. Cai, J. Gao, X. L. Han and J. D. Li, Hydrothermal reduced graphene oxide membranes for dyes removing, Sep. Purif. Technol., 2020, 241, 116730 CrossRef CAS.
- K. H. Thebo, X. T. Qian, Q. Zhang, L. Chen, H. M. Cheng and W. C. Ren, Highly stable graphene-oxide-based membranes with superior permeability, Nat. Commun., 2018, 9, 1486 CrossRef PubMed.
- S. Han, W. Li, H. Xi, R. Yuan, J. Long and C. Xu, Plasma-assisted in-situ preparation of graphene-Ag nanofiltration membranes for efficient removal of heavy metal ions, J. Hazard. Mater., 2022, 423, 127012 CrossRef CAS PubMed.
- E. Yang, M. H. Ham, H. B. Park, C. M. Kim, J. H. Song and I. S. Kim, Tunable semi-permeability of graphene-based membranes by adjusting reduction degree of laminar graphene oxide layer, J. Membr. Sci., 2018, 547, 73–79 CrossRef CAS.
- C. A. Amadei, A. Montessori, J. P. Kadow, S. Succi and C. D. Vecitis, Role of Oxygen Functionalities in Graphene Oxide Architectural Laminate Subnanometer Spacing and Water Transport, Environ. Sci. Technol., 2017, 51, 4280–4288 CrossRef CAS PubMed.
- A. P. Straub, D. S. Bergsman, B. A. Getachew, L. M. Leahy, J. J. Patil, N. Ferralis and J. C. Grossman, Highly Conductive and Permeable Nanocomposite Ultrafiltration Membranes Using Laser-Reduced Graphene Oxide, Nano Lett., 2021, 21, 2429–2435 CrossRef CAS PubMed.
- A. K. Thakur, S. P. Singh, M. N. Kleinberg, A. Gupta and C. J. Arnusch, Laser-Induced Graphene–PVA Composites as Robust Electrically Conductive Water Treatment Membranes, ACS Appl. Mater. Interfaces, 2019, 11, 10914–10921 CrossRef CAS PubMed.
- S. F. Pei and H. M. Cheng, The reduction of graphene oxide, Carbon, 2012, 50, 3210–3228 CrossRef CAS.
- C. Z. Hu, Z. T. Liu, X. L. Lu, J. Q. Sun, H. J. Liu and J. H. Qu, Enhancement of the Donnan effect through capacitive ion increase using an electroconductive rGO-CNT nanofiltration membrane, J. Mater. Chem. A, 2018, 6, 4737–4745 RSC.
- W.-L. Jiang, M. R. Haider, J.-L. Han, Y.-C. Ding, X.-Q. Li, H.-C. Wang, H. M. Adeel Sharif, A.-J. Wang and N.-Q. Ren, Carbon nanotubes intercalated RGO electro-Fenton membrane for coenhanced permeability, rejection and catalytic oxidation of organic micropollutants, J. Membr. Sci., 2021, 623, 119069 CrossRef CAS.
- H. J. Zou, X. P. Li, Y. Q. Zhang, Z. N. Wang, B. Zhuo, P. Ti and Q. P. Yuan, Effects of different hot pressing processes and NFC/GO/CNT composite proportions on the performance of conductive membranes, Mater. Des., 2021, 198, 109334 CrossRef CAS.
- J. A. L. Willcox and H. J. Kim, Molecular dynamics study of water flow across multiple layers of pristine, oxidized, and mixed regions of graphene oxide, ACS Nano, 2017, 11, 2187–2193 CrossRef CAS PubMed.
- B. Chen, H. F. Jiang, X. Liu and X. J. Hu, Observation and analysis of water transport through graphene oxide interlamination, J. Phys. Chem. C, 2017, 121, 1321–1328 CrossRef CAS.
- X. Chen, M. Qiu, H. Ding, K. Fu and Y. Fan, A reduced graphene oxide nanofiltration membrane intercalated by well-dispersed carbon nanotubes for drinking water purification, Nanoscale, 2016, 8, 5696–5705 RSC.
- H. G. Zhang, X. Quan, S. Chen, X. F. Fan, G. L. Wei and H. T. Yu, Combined Effects of Surface Charge and Pore Size on Co-Enhanced Permeability and Ion Selectivity through RGO-OCNT Nanofiltration Membranes, Environ. Sci. Technol., 2018, 52, 4827–4834 CrossRef CAS PubMed.
- S. Yuan, Y. Li, R. S. Qiu, Y. Xia, C. Selomulya and X. W. Zhang, Minimising non-selective defects in ultrathin reduced graphene oxide membranes with graphene quantum dots for enhanced water and NaCl separation, Chin. J. Chem. Eng., 2022, 41, 278–285 CrossRef.
- W. H. Zhang, M. J. Yin, Q. Zhao, C. G. Jin, N. X. Wang, S. L. Ji, C. L. Ritt, M. Elimelech and Q. F. An, Graphene oxide membranes with stable porous structure for ultrafast water transport, Nat. Nanotechnol., 2021, 16, 337–343 CrossRef CAS PubMed.
- W. Zhang, H. Xu, F. Xie, X. Ma, B. Niu, M. Chen, H. Zhang, Y. Zhang and D. Long, General synthesis of ultrafine metal oxide/reduced graphene oxide nanocomposites for ultrahigh-flux nanofiltration membrane, Nat. Commun., 2022, 13, 471 CrossRef CAS PubMed.
- B. Pan and B. S. Xing, Adsorption mechanisms of organic chemicals on carbon nanotubes, Environ. Sci. Technol., 2008, 42, 9005–9013 CrossRef CAS PubMed.
- H. Liu, K. C. Zuo and C. D. Vecitis, Titanium dioxide-coated carbon nanotube network filter for rapid and effective arsenic sorption, Environ. Sci. Technol., 2014, 48, 13871–13879 CrossRef CAS PubMed.
- X. N. Li, S. Chen, X. Quan and Y. B. Zhang, Enhanced adsorption of PFOA and PFOS on multiwalled carbon nanotubes under electrochemical assistance, Environ. Sci. Technol., 2011, 45, 8498–8505 CrossRef CAS PubMed.
- G. L. Wei, X. Quan, S. Chen, X. F. Fan, H. T. Yu and H. M. Zhao, Voltage-gated transport of nanoparticles across free-standing all-carbon-nanotube-based hollow-fiber membranes, ACS Appl. Mater. Interfaces, 2015, 7, 14620–14627 CrossRef CAS PubMed.
- H. G. Zhang, X. Quan, X. F. Fan, G. Yi, S. Chen, H. T. Yu and Y. S. Chen, Improving ion rejection of conductive nanofiltration membrane through electrically enhanced surface charge density, Environ. Sci. Technol., 2018, 53, 868–877 CrossRef PubMed.
- G. Yi, S. Chen, X. Quan, G. L. Wei, X. F. Fan and H. T. Yu, Enhanced separation performance of carbon nanotube–polyvinyl alcohol composite membranes for emulsified oily wastewater treatment under electrical assistance, Sep. Purif. Technol., 2018, 197, 107–115 CrossRef CAS.
- Q. Y. Zhang and C. D. Vecitis, Conductive CNT-PVDF membrane for capacitive organic fouling reduction, J. Membr. Sci., 2014, 459, 143–156 CrossRef CAS.
- S. Wang, S. Liang, P. Liang, X. Y. Zhang, J. Y. Sun, S. J. Wu and X. Huang, In-situ combined dual-layer CNT/PVDF membrane for electrically-enhanced fouling resistance, J. Membr. Sci., 2015, 491, 37–44 CrossRef CAS.
- X. F. Fan, Y. M. Liu, X. Quan, H. M. Zhao, S. Chen, G. Yi and L. Du, High desalination permeability, wetting and fouling resistance on superhydrophobic carbon nanotube hollow fiber membrane under self-powered electrochemical assistance, J. Membr. Sci., 2016, 514, 501–509 CrossRef CAS.
- Y. Yang, S. Qiao, R. F. Jin, J. T. Zhou and X. Quan, Fouling control mechanisms in filtrating natural organic matters by electro-enhanced carbon
nanotubes hollow fiber membranes, J. Membr. Sci., 2018, 553, 54–62 CrossRef CAS.
- Y. Yang, S. Qiao, R. F. Jin, J. T. Zhou and X. Quan, Novel anaerobic electrochemical membrane bioreactor with a CNTs hollow fiber membrane cathode to mitigate membrane fouling and enhance energy recovery, Environ. Sci. Technol., 2019, 53, 1014–1021 CrossRef CAS PubMed.
- X. F. Fan, Y. M. Liu, X. Quan and S. Chen, Highly permeable thin-film composite forward osmosis membrane based on carbon nanotube hollow fiber scaffold with electrically enhanced fouling resistance, Environ. Sci. Technol., 2018, 52, 1444–1452 CrossRef CAS PubMed.
- S. Bhattacharjee and M. Elimelech, Surface element integration: a novel technique for evaluation of DLVO interaction between a particle and a flat plate, J. Colloid Interface Sci., 1997, 193, 273–285 CrossRef CAS PubMed.
- R. N. Zhang, Y. A. Liu, M. R. He, Y. L. Su, X. T. Zhao, M. Elimelech and Z. Y. Jiang, Antifouling membranes for sustainable water purification: Strategies and mechanisms, Chem. Soc. Rev., 2016, 45, 5888–5924 RSC.
- G. D. Gao and C. D. Vecitis, Electrochemical carbon nanotube filter oxidative performance as a function of surface chemistry, Environ. Sci. Technol., 2011, 45, 9726–9734 CrossRef CAS PubMed.
- C. D. Vecitis, M. H. Schnoor, M. S. Rahaman, J. D. Schiffman and M. Elimelech, Electrochemical multiwalled carbon nanotube filter for viral and bacterial removal and inactivation, Environ. Sci. Technol., 2011, 45, 3672–3679 CrossRef CAS PubMed.
- C. D. Vecitis, G. D. Gao and H. Liu, Electrochemical carbon nanotube filter for adsorption, desorption, and oxidation of aqueous dyes and anions, J. Phys. Chem. C, 2011, 115, 3621–3629 CrossRef CAS.
- G. D. Gao and C. D. Vecitis, Reactive depth and performance of an electrochemical carbon nanotube network as a function of mass transport, ACS Appl. Mater. Interfaces, 2012, 4, 6096–6103 CrossRef CAS PubMed.
- M. S. Rahaman, C. D. Vecitis and M. Elimelech, Electrochemical carbon-nanotube filter performance toward virus removal and inactivation in the presence of natural organic matter, Environ. Sci. Technol., 2012, 46, 1556–1564 CrossRef CAS PubMed.
- H. Liu and C. D. Vecitis, Reactive transport mechanism for organic oxidation during electrochemical filtration: Mass-transfer, physical adsorption, and electron-transfer, J. Phys. Chem. C, 2011, 116, 374–383 CrossRef.
- G. D. Gao and C. D. Vecitis, Electrocatalysis aqueous phenol with carbon nanotubes networks as anodes: Electrodes passivation and regeneration and prevention, Electrochim. Acta, 2013, 98, 131–138 CrossRef CAS.
- M. H. Schnoor and C. D. Vecitis, Quantitative examination of aqueous ferrocyanide oxidation in a carbon nanotube electrochemical filter: Effects of flow rate, ionic strength, and cathode material, J. Phys. Chem. C, 2013, 117, 2855–2867 CrossRef CAS.
- G. D. Gao, Q. Y. Zhang and C. D. Vecitis, CNT-PVDF composite flow-through electrode for single-pass sequential reduction-oxidation, J. Mater. Chem. A, 2014, 2, 6185–6190 RSC.
- Y. B. Liu, H. Liu, Z. Zhou, T. R. Wang, C. N. Ong and C. D. Vecitis, Degradation of the common aqueous antibiotic tetracycline using a carbon nanotube electrochemical filter, Environ. Sci. Technol., 2015, 49, 7974–7980 CrossRef CAS PubMed.
- Y. Yang, S. Qiao, R. F. Jin, J. T. Zhou and X. Quan, A Novel aerobic electrochemical membrane bioreactor with CNTs hollow fiber membrane by electrochemical oxidation to improve water quality and mitigate membrane fouling, Water Res., 2019, 151, 54–63 CrossRef CAS PubMed.
- G. L. Wei, X. Quan, X. F. Fan, S. Chen and Y. B. Zhang, Carbon-nanotube-based sandwich-like hollow fiber membranes for expanded Microcystin-LR removal applications, Chem. Eng. J., 2017, 319, 212–218 CrossRef CAS.
- C. Zhang, Y. H. Jiang, Y. L. Li, Z. X. Hu, L. Zhou and M. H. Zhou, Three-dimensional electrochemical process for wastewater treatment: A general review, Chem. Eng. J., 2013, 228, 455–467 CrossRef CAS.
- C. A. Martinez-Huitle and S. Ferro, Electrochemical oxidation of organic pollutants for the wastewater treatment: Direct and indirect processes, Chem. Soc. Rev., 2006, 35, 1324–1340 RSC.
- A. Vlyssides, E. M. Barampouti, S. Mai, D. Arapoglou and A. Kotronarou, Degradation of methylparathion in aqueous solution by electrochemical oxidation, Environ. Sci. Technol., 2004, 38, 6125–6131 CrossRef CAS PubMed.
- O. Simond, V. Schaller and C. Comninellis, Theoretical model for the anodic oxidation of organics on metal oxide electrodes, Electrochim. Acta, 1997, 42, 2009–2012 CrossRef CAS.
- M. H. Zhou, H. Sarkka and M. Sillanpaa, A comparative experimental study on methyl orange degradation by electrochemical oxidation on BDD and MMO electrodes, Sep. Purif. Technol., 2011, 78, 290–297 CrossRef CAS.
- S. Ohmori and T. Saito, Electrochemical durability of single-wall carbon nanotube electrode against anodic oxidation in water, Carbon, 2012, 50, 4932–4938 CrossRef CAS.
- M. Tominaga, Y. Yatsugi and N. Watanabe, Oxidative corrosion potential vs. pH diagram for single-walled carbon nanotubes, RSC Adv., 2014, 4, 27224–27227 RSC.
- X. Y. Lu and C. Zhao, Highly efficient and robust oxygen evolution catalysts achieved by anchoring nanocrystalline cobalt oxides onto mildly oxidized multiwalled carbon nanotubes, J. Mater. Chem. A, 2013, 1, 12053–12059 RSC.
- H. Liu, A. Vajpayee and C. D. Vecitis, Bismuth-doped tin oxide-coated carbon nanotube network: Improved anode stability and efficiency for flow-through organic electrooxidation, ACS Appl. Mater. Interfaces, 2013, 5, 10054–10066 CrossRef CAS PubMed.
- S. Y. Yang, C. D. Vecitis and H. Park, Electrocatalytic water treatment using carbon nanotube filters modified with metal oxides, Environ. Sci. Pollut. Res., 2019, 26, 1036–1043 CrossRef CAS PubMed.
- W. Y. Duan, A. Ronen, S. Walker and D. Jassby, Polyaniline-coated carbon nanotube ultrafiltration membranes: enhanced anodic stability for in situ cleaning and electro-oxidation processes, ACS Appl. Mater. Interfaces, 2016, 8, 22574–22584 CrossRef CAS PubMed.
- C. Cuevas, D. Kim, K. P. Katuri, P. Saikaly and S. P. Nunes, Electrochemically active polymeric hollow fibers based on poly (ether-b-amide)/carbon nanotubes, J. Membr. Sci., 2018, 545, 323–328 CrossRef CAS.
- Y. B. Liu, J. P. Xie, C. N. Ong, C. D. Vecitis and Z. Zhou, Electrochemical wastewater treatment with carbon nanotube filters coupled with in situ generated H2O2, Environ. Sci.: Water Res. Technol., 2015, 1, 769–778 RSC.
- G. D. Gao, Q. Y. Zhang, Z. W. Hao and C. D. Vecitis, Carbon nanotube membrane stack for flow-through sequential regenerative electro-Fenton, Environ. Sci. Technol., 2015, 49, 2375–2383 CrossRef CAS PubMed.
- X. H. Sun, J. Wu, Z. Q. Chen, X. Su and B. J. Hinds, Fouling characteristics and electrochemical recovery of carbon nanotube membranes, Adv. Funct. Mater., 2013, 23, 1500–1506 CrossRef CAS.
- B. S. Lalia, F. E. Ahmed, T. Shah, N. Hilal and R. Hashaikeh, Electrically conductive membranes based on carbon nanostructures for self-cleaning of biofouling, Desalination, 2015, 360, 8–12 CrossRef CAS.
- B. W. Xin and J. C. Hao, Reversibly switchable wettability, Chem. Soc. Rev., 2010, 39, 769–782 RSC.
- J. Lahann, S. Mitragotri, T. N. Tran, H. Kaido, J. Sundaram, I. S. Choi, S. Hoffer, G. A. Somorjai and R. Langer, A reversibly switching surface, Science, 2003, 299, 371–374 CrossRef CAS PubMed.
- L. B. Xu, W. Chen, A. Mulchandani and Y. S. Yan, Reversible conversion of conducting polymer films from superhydrophobic to superhydrophilic, Angew. Chem., Int. Ed., 2005, 44, 6009–6012 CrossRef CAS PubMed.
- Z. K. Wang, L. J. Ci, L. Chen, S. Nayak, P. M. Ajayan and N. Koratkar, Polarity-dependent electrochemically controlled transport of water through carbon nanotube membranes, Nano Lett., 2007, 7, 697–702 CrossRef CAS PubMed.
- S. L. Zhou, J. D. Sheng, Z. H. Yang and X. H. Zhang, Enhanced ion transport in densified CNT arrays, J. Mater. Chem. A, 2018, 6, 8763–8771 RSC.
- L. B. Zhu, J. W. Xu, Y. H. Xiu, Y. Y. Sun, D. W. Hess and C. P. Wong, Electrowetting of aligned carbon nanotube films, J. Phys. Chem. B, 2006, 110, 15945–15950 CrossRef CAS PubMed.
- G. Zhang, Z. Duan, Q. G. Wang, L. Li, W. Yao and C. H. Liu, Electrical potential induced switchable wettability of super-aligned carbon nanotube films, Appl. Surf. Sci., 2018, 427, 628–635 CrossRef CAS.
- B. A. Kakade, Chemical control of superhydrophobicity of carbon nanotube surfaces: droplet pinning and electrowetting behavior, Nanoscale, 2013, 5, 7011–7016 RSC.
- B. Kakade, R. Mehta, A. Durge, S. Kulkarni and V. Pillai, Electric field induced, superhydrophobic to superhydrophilic switching in multiwalled carbon nanotube papers, Nano Lett., 2008, 8, 2693–2696 CrossRef CAS PubMed.
- D. W. Lu, T. Zhang, L. Gutierrez, J. Ma and J. P. Croué, Influence of surface properties of filtration-layer metal oxide on ceramic membrane fouling during ultrafiltration of oil/water emulsion, Environ. Sci. Technol., 2016, 50, 4668–4674 CrossRef CAS PubMed.
- D. P. Liu, D. Li, D. Du, X. Z. Zhao, A. W. Qin, X. Li and C. J. He, Antifouling PVDF membrane with hydrophilic surface of terry pile-like structure, J. Membr. Sci., 2015, 493, 243–251 CrossRef CAS.
- N. Maximous, G. Nakhla and W. Wan, Comparative assessment of hydrophobic and hydrophilic membrane fouling in wastewater applications, J. Membr. Sci., 2009, 339, 93–99 CrossRef CAS.
- L. Du, X. Quan, X. F. Fan, S. Chen and H. T. Yu, Electro-responsive carbon membranes with reversible superhydrophobicity/superhydrophilicity switch for efficient oil/water separation, Sep. Purif. Technol., 2019, 210, 891–899 CrossRef CAS.
- M. Majumder, X. Zhan, R. Andrews and B. J. Hinds, Voltage gated carbon nanotube membranes, Langmuir, 2007, 23, 8624–8631 CrossRef CAS PubMed.
- C. E. Ren, M. Alhabeb, B. W. Byles, M. Zhao, B. Anasori, E. Pomerantseva, K. A. Mahmoud and Y. Gogotsi, Voltage-gated ions sieving through 2D MXene Ti3C2Tx membranes, ACS Appl. Nano Mater., 2018, 1, 3644–3652 CrossRef CAS.
- Y. Wang, R. Liang, T. Jia, X. Cao, Q. Wang, J. Cao, S. Li, Q. Shi, L. Isaacs and S. Sun, Voltage-gated membranes incorporating Cucurbit[n]uril molecular containers for molecular nanofiltration, J. Am. Chem. Soc., 2022, 144, 6483–6492 CrossRef CAS PubMed.
- K.-G. Zhou, K. S. Vasu, C. T. Cherian, M. Neek-Amal, J. C. Zhang, H. Ghorbanfekr-Kalashami, K. Huang, O. P. Marshall, V. G. Kravets, J. Abraham, Y. Su, A. N. Grigorenko, A. Pratt, A. K. Geim, F. M. Peeters, K. S. Novoselov and R. R. Nair, Electrically controlled water permeation through graphene oxide membranes, Nature, 2018, 559, 236–240 CrossRef CAS PubMed.
- M. Cheng, W. Zhang, W. Yuan, J. Xue, C. Li and S. Hou, Rational fabrication of MXene/graphene oxide membrane and its voltage-gated ion transport behavior, ACS Sustainable Chem. Eng., 2021, 9, 7206–7210 CrossRef CAS.
- L. Cantley, J. L. Swett, D. Lloyd, D. A. Cullen, K. Zhou, P. V. Bedworth, S. Heise, A. J. Rondinone, Z. Xu, S. Sinton and J. S. Bunch, Voltage gated inter-cation selective ion channels from graphene nanopores, Nanoscale, 2019, 11, 9856–9861 RSC.
- S. P. Surwade, S.-H. Chai, J.-P. Choi, X. Wang, J. S. Lee, I. V. Vlassiouk, S. M. Mahurin and S. Dai, Electrochemical control of ion transport through a mesoporous carbon membrane, Langmuir, 2014, 30, 3606–3611 CrossRef CAS PubMed.
- Y. Wang, H. Zhang, Y. Kang, Y. Zhu, G. P. Simon and H. Wang, Voltage-gated ion transport in two-dimensional sub-1 nm nanofluidic channels, ACS Nano, 2019, 13, 11793–11799 CrossRef CAS PubMed.
- J. Wu, K. Gerstandt, M. Majumder, X. Zhan and B. J. Hinds, Highly efficient electroosmotic flow through functionalized carbon nanotube membranes, Nanoscale, 2011, 3, 3321–3328 RSC.
- S. A. Miller, V. Y. Young and C. R. Martin, Electroosmotic Flow in Template-Prepared Carbon Nanotube Membranes, J. Am. Chem. Soc., 2001, 123, 12335–12342 CrossRef CAS PubMed.
- J. Wu, K. Gerstandt, H. B. Zhang, J. Liu and B. J. Hinds, Electrophoretically induced aqueous flow through single-walled carbon nanotube membranes, Nat. Nanotechnol., 2012, 7, 133–139 CrossRef CAS PubMed.
- L. Ramsay, M. M. Petersen, B. Hansen, J. Schullehner, P. van der Wens, D. Voutchkova and S. M. Kristiansen, Drinking Water Criteria for Arsenic in High-Income, Low-Dose Countries: The Effect of Legislation on Public Health, Environ. Sci. Technol., 2021, 55, 3483–3493 CrossRef CAS PubMed.
- Q. Ding, C. Li, H. Wang, C. Xu and H. Kuang, Electrochemical detection of heavy metal ions in water, Chem. Commun., 2021, 57, 7215–7231 RSC.
- L. A. Malik, A. Bashir, A. Qureashi and A. H. Pandith, Detection and removal of heavy metal ions: a review, Environ. Chem. Lett., 2019, 17, 1495–1521 CrossRef CAS.
- C. Thamaraiselvan, W. J. Lau and C. G. Dosoretz, Coupled electrochemical transformation and filtration of water pollutants by cathodic-carbon nanotube membranes, J. Environ. Chem. Eng., 2022, 10, 107670 CrossRef CAS.
- S. Ma, F. Yang, X. Chen, C. M. Khor, B. Jung, A. Iddya, G. Sant and D. Jassby, Removal of As(III) by Electrically Conducting Ultrafiltration Membranes, Water Res., 2021, 204, 117592 CrossRef CAS PubMed.
- Y. Liu, S. Yang, H. Jiang, B. Yang, X. Fang, C. Shen, J. Yang, W. Sand and F. Li, Sea urchin-like FeOOH functionalized electrochemical CNT filter for one-step arsenite decontamination, J. Hazard. Mater., 2021, 407, 124384 CrossRef CAS PubMed.
- L. Liu, Y. Xu, K. P. Wang, K. L. Li, L. L. Xu, J. B. Wang and J. Wang, Fabrication of a novel conductive ultrafiltration membrane and its application for electrochemical removal of hexavalent chromium, J. Membr. Sci., 2019, 584, 191–201 CrossRef CAS.
- Y. Ren, Y. Liu, F. Liu, F. Li, C. Shen and Z. Wu, Extremely efficient electro-Fenton-like Sb(III) detoxification using nanoscale Ti-Ce binary oxide: An effective design to boost catalytic activity via non-radical pathway, Chin. Chem. Lett., 2021, 32, 2519–2523 CrossRef CAS.
- Y. Liu, J. Zhang, F. Liu, C. Shen, F. Li, M. Huang, B. Yang, Z. Wang and W. Sand, Ultra-rapid detoxification of Sb(III) using a flow-through electro-Fenton system, Chemosphere, 2020, 245, 125604 CrossRef CAS PubMed.
- K. M. Kekre, A. Anvari, K. Kahn, Y. Yao and A. Ronen, Reactive electrically conducting membranes for phosphorus recovery from livestock wastewater effluents, J. Environ. Manage., 2021, 282, 111432 CrossRef CAS PubMed.
- B. Jung, C. Y. Kim, S. Jiao, U. Rao, A. V. Dudchenko, J. Tester and D. Jassby, Enhancing boron rejection on electrically conducting reverse osmosis membranes through local electrochemical pH modification, Desalination, 2020, 476, 114212 CrossRef CAS.
- F. Li, X. Peng, Y. Liu, J. Mei, L. Sun, C. Shen, C. Ma, M. Huang, Z. Wang and W. Sand, A chloride-radical-mediated electrochemical filtration system for rapid and effective transformation of ammonia to nitrogen, Chemosphere, 2019, 229, 383–391 CrossRef CAS PubMed.
- H. Zhang, X. Quan, S. Chen, H. Yu and J. Niu, Electrokinetic Enhancement of Water Flux and Ion Rejection through Graphene Oxide/Carbon Nanotube Membrane, Environ. Sci. Technol., 2020, 54, 15433–15441 CrossRef CAS PubMed.
- J. Sun, C. Hu, B. Wu, H. Liu and J. Qu, Improving ion rejection of graphene oxide conductive membranes by applying electric field, J. Membr. Sci., 2020, 604, 118077 CrossRef CAS.
- N. K. Khanzada, D. Jassby and A. K. An, Conductive reverse osmosis membrane for electrochemical chlorine reduction and sustainable brackish water treatment, Chem. Eng. J., 2022, 435, 134858 CrossRef CAS.
- C. Ng, I. T. Cousins, J. C. DeWitt, J. Glüge, G. Goldenman, D. Herzke, R. Lohmann, M. Miller, S. Patton, M. Scheringer, X. Trier and Z. Wang, Addressing Urgent Questions for PFAS in the 21st Century, Environ. Sci. Technol., 2021, 55, 12755–12765 CAS.
- M.-A. Pétré, D. P. Genereux, L. Koropeckyj-Cox, D. R. U. Knappe, S. Duboscq, T. E. Gilmore and Z. R. Hopkins, Per- and Polyfluoroalkyl Substance (PFAS) Transport from Groundwater to Streams near a PFAS Manufacturing Facility in North Carolina, USA, Environ. Sci. Technol., 2021, 55, 5848–5856 CrossRef PubMed.
- A. Previšić, M. Vilenica, N. Vučković, M. Petrović and M. Rožman, Aquatic Insects Transfer Pharmaceuticals and Endocrine Disruptors from Aquatic to Terrestrial Ecosystems, Environ. Sci. Technol., 2021, 55, 3736–3746 CrossRef PubMed.
- I. J. Neuwald, D. Hübner, H. L. Wiegand, V. Valkov, U. Borchers, K. Nödler, M. Scheurer, S. E. Hale, H. P. H. Arp and D. Zahn, Ultra-Short-Chain PFASs in the Sources of German Drinking Water: Prevalent, Overlooked, Difficult to Remove, and Unregulated, Environ. Sci. Technol., 2022, 56, 6380–6390 CrossRef CAS PubMed.
- A. Buffa and D. Mandler, Adsorption and detection of organic pollutants by fixed bed carbon nanotube electrochemical membrane, Chem. Eng. J., 2019, 359, 130–137 CrossRef CAS.
- W. Wang, C. Xie, L. Zhu, B. Shan, C. Liu and F. Cui, A novel 3-dimensional graphene-based membrane with superior water flux and electrocatalytic properties for organic pollutant degradation, J. Mater. Chem. A, 2019, 7, 172–187 RSC.
- A. J. Sutherland, M.-X. Ruiz-Caldas and C.-F. de Lannoy, Electro-catalytic microfiltration membranes electrochemically degrade azo dyes in solution, J. Membr. Sci., 2020, 611, 118335 CrossRef CAS.
- H. J. E. Yanez, Z. Wang, S. Lege, M. Obst, S. Roehler, C. J. Burkhardt and C. Zwiener, Application and characterization of electroactive membranes based on carbon nanotubes and zerovalent iron nanoparticles, Water Res., 2017, 108, 78–85 CrossRef PubMed.
- S. Almassi, Z. Li, W. Q. Xu, C. C. Pu, T. Zeng and B. P. Chaplin, Simultaneous adsorption and electrochemical reduction of N-Nitrosodimethylamine using carbon-Ti4O7 composite reactive electrochemical membranes, Environ. Sci. Technol., 2019, 53, 928–937 CrossRef CAS PubMed.
- J. J. Zheng, Z. W. Wang, J. X. Ma, S. P. Xu and Z. C. Wu, Development of an electrochemical ceramic membrane filtration system for efficient contaminant removal from waters, Environ. Sci. Technol., 2018, 52, 4117–4126 CrossRef CAS PubMed.
- S. F. Anis, B. S. Lalia, A. Lesimple, R. Hashaikeh and N. Hilal, Electrically conductive membranes for contemporaneous dye rejection and degradation, Chem. Eng. J., 2022, 428, 131184 CrossRef CAS.
- L. L. Xu, K. P. Wang, K. L. Li, S. Y. Zhao and J. Wang, Development and performance of stable PANI/MWNT conductive membrane for contaminants degradation and anti-fouling behavior, Sep. Purif. Technol., 2022, 282, 120112 CrossRef CAS.
- L. Yang, D. Xu, H. Yang, X. Luo and H. Liang, Structurally-controlled FeNi LDH/CNTs electro-Fenton membrane for in-situ electro-generation and activation of hydroxyl radicals toward organic micropollutant treatment, Chem. Eng. J., 2022, 432, 134436 CrossRef CAS.
- X. Yang, X. Li, Y. Hu, J. Cheng and Y. Chen, A High Flux Electrochemical Filtration System Based on Electrospun Carbon Nanofiber Membrane for Efficient Tetracycline Degradation, Water, 2022, 14, 910 CrossRef CAS.
- S. Yu, Y. Gao, R. Khan, P. Liang, X. Zhang and X. Huang, Electrospun PAN-based graphene/SnO2 carbon nanofibers as anodic electrocatalysis microfiltration membrane for sulfamethoxazole degradation, J. Membr. Sci., 2020, 614, 118368 CrossRef CAS.
- A. R. Bakr and M. S. Rahaman, Removal of bisphenol A by electrochemical carbon-nanotube filter: Influential factors and degradation pathway, Chemosphere, 2017, 185, 879–887 CrossRef CAS PubMed.
- A. R. Bakr and M. S. Rahaman, Electrochemical efficacy of a carboxylated multiwalled carbon nanotube filter for the removal of ibuprofen from aqueous solutions under acidic conditions, Chemosphere, 2016, 153, 508–520 CrossRef CAS PubMed.
- S. Yang, Y. Liu, C. Shen, F. Li, B. Yang, M. Huang, M. Yang, Z. Wang and W. Sand, Rapid decontamination of tetracycline hydrolysis product using electrochemical CNT filter: Mechanism, impacting factors and pathways, Chemosphere, 2020, 244, 125525 CrossRef CAS PubMed.
- D. Guo and Y. Liu, Singlet Oxygen-Mediated Electrochemical Filter for Selective and Rapid Degradation of Organic Compounds, Ind. Eng. Chem. Res., 2020, 59, 14180–14187 CrossRef CAS.
- M. Pan, J. Wang, G. Gao and J. W. Chew, Incorporation of single cobalt active sites onto N-doped graphene for superior conductive membranes in electrochemical filtration, J. Membr. Sci., 2020, 602, 117966 CrossRef CAS.
- H. Liu, J. Liu, Y. B. Liu, K. Bertoldi and C. D. Vecitis, Quantitative 2D electrooxidative carbon nanotube filter model: Insight into reactive sites, Carbon, 2014, 80, 651–664 CrossRef CAS.
- A. R. Bakr and M. S. Rahaman, Crossflow electrochemical filtration for elimination of ibuprofen and bisphenol a from pure and competing electrolytic solution conditions, J. Hazard. Mater., 2019, 365, 615–621 CrossRef CAS PubMed.
- G. D. Gao, M. L. Pan and C. D. Vecitis, Effect of the oxidation approach on carbon nanotube surface functional groups and electrooxidative filtration performance, J. Mater. Chem. A, 2015, 3, 7575–7582 RSC.
- C. Shi, S. Yu and C. Li, Fabrication of aligned carbon nanofiber doped with SnO2-Sb for efficient electrochemical removal of tetracycline, Chem. Eng. J., 2022, 441, 136052 CrossRef CAS.
- H.-J. Lee, N. Zhang, M. A. Ganzoury, Y. Wu and C.-F. de Lannoy, Simultaneous Dechlorination and Advanced Oxidation Using Electrically Conductive Carbon Nanotube Membranes, ACS Appl. Mater. Interfaces, 2021, 13, 34084–34092 CrossRef CAS PubMed.
- K. Lee, J. Lim, M. J. Lee, K. Ryu, H. Lee, J. Y. Kim, H. Ju, H.-S. Cho, B.-H. Kim, M. C. Hatzell, J. Kang and S. W. Lee, Structure-Controlled Graphene Electrocatalysts for High-Performance H2O2 Production, Energy Environ. Sci., 2022, 15, 2858–2866 RSC.
- X. Zhang, Z. Yao, J. Wang, W. Guo, X. Wu and Z. Jiang, High-capacity NCNT-encapsulated metal NP catalysts on carbonised loofah with dual-reaction centres over C–M bond bridges for Fenton-like degradation of antibiotics, Appl. Catal., B, 2022, 307, 121205 CrossRef CAS.
- Y. Wang, Y. Zhou, Y. Feng and X.-Y. Yu, Synergistic Electronic and Pore Structure Modulation in Open Carbon Nanocages Enabling Efficient Electrocatalytic Production of H2O2 in Acidic Medium, Adv. Funct. Mater., 2022, 32, 2110734 CrossRef CAS.
- P. Y. Liang, M. Rivallin, S. Cerneaux, S. Lacour, E. Petit and M. Cretin, Coupling cathodic electro-Fenton reaction to membrane filtration for AO7 dye degradation: A successful feasibility study, J. Membr. Sci., 2016, 510, 182–190 CrossRef CAS.
- L. Cui, H. Huang, P. Ding, S. Zhu, W. Jing and X. Gu, Cogeneration of H2O2 and OH via a novel Fe3O4/MWCNTs composite cathode in a dual-compartment electro-Fenton membrane reactor, Sep. Purif. Technol., 2020, 237, 116380 CrossRef CAS.
- D. Guo, Y. Liu, H. Ji, C.-C. Wang, B. Chen, C. Shen, F. Li, Y. Wang, P. Lu and W. Liu, Silicate-Enhanced Heterogeneous Flow-Through Electro-Fenton System Using Iron Oxides under Nanoconfinement, Environ. Sci. Technol., 2021, 55, 4045–4053 CrossRef CAS PubMed.
- Z. Li, C. Shen, Y. Liu, C. Ma, F. Li, B. Yang, M. Huang, Z. Wang, L. Dong and S. Wolfgang, Carbon nanotube filter functionalized with iron oxychloride for flow-through electro-Fenton, Appl. Catal., B, 2020, 260, 118204 CrossRef CAS.
- F. Liu, Y. Liu, Q. Yao, Y. Wang, X. Fang, C. Shen, F. Li, M. Huang, Z. Wang, W. Sand and J. Xie, Supported Atomically-Precise Gold Nanoclusters for Enhanced Flow-through Electro-Fenton, Environ. Sci. Technol., 2020, 54, 5913–5921 CrossRef CAS PubMed.
- L. T. X. Huong, L. F. Dumée, S. Lacour, M. Rivallin, Z. Yi, L. Kong, M. Bechelany and M. Cretin, Hybrid graphene-decorated metal hollow fibre membrane reactors for efficient electro-Fenton - Filtration co-processes, J. Membr. Sci., 2019, 587, 117182 CrossRef.
- X. Wang, H. Wang, F. Li, X. Hu, Z. Xie and T. Hua, Activation of peroxymonosulfate in an electrochemical filter by MnFe2O4-rGO electro-assisted catalytic membrane for the degradation of oxytetracycline, J. Environ. Chem. Eng., 2022, 10, 107008 CrossRef CAS.
- W. Zheng, Y. Liu, W. Liu, H. Ji, F. Li, C. Shen, X. Fang, X. Li and X. Duan, A novel electrocatalytic filtration system with carbon nanotube supported nanoscale zerovalent copper toward ultrafast oxidation of organic pollutants, Water Res., 2021, 194, 116961 CrossRef CAS PubMed.
- Q. Xu, Y. Liu, Y. Wang, Y. Song, C. Zhao and L. Han, Synergistic oxidation-filtration process of electroactive peroxydisulfate with a cathodic composite CNT-PPy/PVDF ultrafiltration membrane, Water Res., 2022, 210, 117971 CrossRef CAS PubMed.
- Q. Yang, M. Li, J. Wang and H. Huang, Synergistic electro-catalytic oxidation of ibuprofen in electro-peroxone system with flow-through carbon nanotube membrane cathode, Chem. Eng. J., 2022, 435, 135180 CrossRef CAS.
- D. Zhi, J. B. Wang, Y. Y. Zhou, Z. R. Luo, Y. Q. Sun, Z. H. Wan, L. Luo, D. C. W. Tsang and D. D. Dionysiou, Development of ozonation and reactive electrochemical membrane coupled process: Enhanced tetracycline mineralization and toxicity reduction, Chem. Eng. J., 2019, 383, 123149 CrossRef.
- Q. Yang, H. O. Huang, K. L. Li, Y. J. Wang, J. Wang and X. Y. Zhang, Ibuprofen removal from drinking water by electro-peroxone in carbon cloth filter, Chem. Eng. J., 2020, 415, 127618 CrossRef.
- F. R. Omi, M. R. Choudhury, N. Anwar, A. R. Bakr and M. S. Rahaman, Highly conductive ultrafiltration membrane via vacuum filtration assisted layer-by-layer deposition of functionalized carbon nanotubes, Ind. Eng. Chem. Res., 2017, 56, 8474–8484 CrossRef CAS.
- A. N. Zhu, H. K. Liu, F. Long, E. Z. Su and A. M. Klibanov, Inactivation of bacteria by electric current in the presence of carbon nanotubes embedded within a polymeric membrane, Appl. Biochem. Biotechnol., 2015, 175, 666–676 CrossRef CAS PubMed.
- L. Xie, Y. Shu, Y. Hu, J. Cheng and Y. Chen, SWNTs-PAN/TPU/PANI composite electrospun nanofiber membrane for point-of-use efficient electrochemical disinfection: New strategy of CNT disinfection, Chemosphere, 2020, 251, 126286 CrossRef CAS PubMed.
- K. C. Ho, Y. H. Teow, A. W. Mohammad, W. L. Ang and P. H. Lee, Development of graphene oxide (GO)/multi-walled carbon nanotubes (MWCNTs) nanocomposite conductive membranes for electrically enhanced fouling mitigation, J. Membr. Sci., 2018, 552, 189–201 CrossRef CAS.
- Q. Zhang, J. Wang and C. D. Vecitis, Fouling reduction and recovery during forward osmosis of wastewater using an electroactive CNT composite membrane, J. Membr. Sci., 2021, 620, 118803 CrossRef CAS.
- W. Yuan and A. L. Zydney, Humic acid fouling during ultrafiltration, Environ. Sci. Technol., 2000, 34, 5043–5050 CrossRef CAS.
- W. Yuan and A. L. Zydney, Humic acid fouling during microfiltration, J. Membr. Sci., 1999, 157, 1–12 CrossRef CAS.
- M. Cao, Y. Zhang, B. Zhang, Z. Liu, X. Ma and C. Chen, The preparation of a modified PVDF hollow fiber membrane by coating with multiwalled carbon nanotubes for high antifouling performance, RSC Adv., 2020, 10, 1848–1857 RSC.
- W. Y. Duan, A. Ronen, J. V. Leon, A. Dudchenko, S. Y. Yao, J. Corbala-Delgado, A. Yan, M. Matsumoto and D. Jassby, Treating anaerobic sequencing batch reactor effluent with electrically conducting ultrafiltration and nanofiltration membranes for fouling control, J. Membr. Sci., 2016, 504, 104–112 CrossRef CAS.
- F. Gao and C. E. Nebel, Electrically conductive diamond membrane for electrochemical separation processes, ACS Appl. Mater. Interfaces, 2016, 8, 18640–18646 CrossRef CAS PubMed.
- F. Sun, J. Yang, Q. Shen, M. Li, H. Du and D. Y. Xing, Conductive polyethersulfone membrane facilely prepared by simultaneous phase inversion method for enhanced anti-fouling and separation under low driven-pressure, J. Environ. Manage., 2021, 297, 113363 CrossRef CAS PubMed.
- Q. Liu, G. Qiu, Z. Zhou, J. Li, G. L. Amy, J. Xie and J. Y. Lee, An Effective Design of Electrically Conducting Thin-Film Composite (TFC) Membranes for Bio and Organic Fouling Control in Forward Osmosis (FO), Environ. Sci. Technol., 2016, 50, 10596–10605 CrossRef CAS PubMed.
- L. L. Xu, L. Liu, K. P. Wang, S. Y. Zhao, Q. Y. Liu, Y. Zhang and J. Wang, Development of a novel electrocoagulation membrane reactor with electrically conductive membranes as cathode to mitigate membrane fouling, J. Membr. Sci., 2021, 618, 118713 CrossRef CAS.
- J. Kim, E.-T. Yun, L. Tijing, H. K. Shon and S. Hong, Mitigation of fouling and wetting in membrane distillation by electrical repulsion using a multi-layered single-wall carbon nanotube/polyvinylidene fluoride membrane, J. Membr. Sci., 2022, 653, 120519 CrossRef CAS.
- Y. Dong, L. Ma, C. Y. Tang, F. Yang, X. Quan, D. Jassby, M. J. Zaworotko and M. D. Guiver, Stable Superhydrophobic Ceramic-Based Carbon Nanotube Composite Desalination Membranes, Nano Lett., 2018, 18, 5514–5521 CrossRef CAS PubMed.
- Y. Si, C. Sun, D. Li, F. Yang, C. Y. Tang, X. Quan, Y. Dong and M. D. Guiver, Flexible Superhydrophobic Metal-Based Carbon Nanotube Membrane for Electrochemically Enhanced Water Treatment, Environ. Sci. Technol., 2020, 54, 9074–9082 CrossRef CAS PubMed.
- L. Jiang, L. Chen and L. Zhu, In-situ electric-enhanced membrane distillation for simultaneous flux-increasing and anti-wetting, J. Membr. Sci., 2021, 630, 119305 CrossRef CAS.
- M. Han, Y. Wang, J. Yao, C. Liu, J. W. Chew, Y. Wang, Y. Dong and L. Han, Electrically conductive hydrophobic membrane cathode for membrane distillation with super anti-oil-fouling capability: Performance and mechanism, Desalination, 2021, 516, 115199 CrossRef CAS.
- Y. Shi, Q. Zheng, L. Ding, F. Yang, W. Jin, C. Y. Tang and Y. Dong, Electro-Enhanced Separation of Microsized Oil-in-Water Emulsions via Metallic Membranes: Performance and Mechanistic Insights, Environ. Sci. Technol., 2022, 56, 4518–4530 CrossRef CAS PubMed.
- X. Chen, J. Gao, Y. Song, Y. Gong, M. Qi and R. Hao, Fabrication of a High Water Flux Conductive MWCNTs/PVC Composite Membrane with Effective Electrically Enhanced Antifouling Behavior, Coatings, 2021, 11, 1548 CrossRef CAS.
- S. F. Anis, B. S. Lalia, M. Khair, R. Hashaikeh and N. Hilal, Electro-ceramic self-cleaning membranes for biofouling control and prevention in water treatment, Chem. Eng. J., 2021, 415, 128395 CrossRef CAS.
- L. J. Jiang, L. Chen and L. Zhu, Electrically conductive membranes for anti-biofouling in membrane distillation with two novel operation modes: Capacitor mode and resistor mode, Water Res., 2019, 161, 297–307 CrossRef CAS PubMed.
- A. Ronen, W. Y. Duan, I. Wheeldon, S. Walker and D. Jassby, Microbial attachment inhibition through low-voltage electrochemical reactions on electrically conducting membranes, Environ. Sci. Technol., 2015, 49, 12741–12750 CrossRef CAS PubMed.
- M. A. Halali and C.-F. de Lannoy, Quantifying the Impact of Electrically Conductive Membrane-Generated Hydrogen Peroxide and Extreme pH on the Viability of Escherichia coli Biofilms, Ind. Eng. Chem. Res., 2022, 61, 660–671 CrossRef CAS.
- Q. Y. Zhang, P. Arribas, E. M. Remillard, G. M. Carmen, M. Khayet and C. D. Vecitis, Interlaced CNT electrodes for bacterial fouling reduction of microfiltration membranes, Environ. Sci. Technol., 2017, 51, 9176–9183 CrossRef CAS PubMed.
- C. Thamaraiselvan, A. Ronen, S. Lerman, M. Balaish, Y. Ein-Eli and C. G. Dosoretz, Low voltage electric potential as a driving force to hinder biofouling in self-supporting carbon nanotube membranes, Water Res., 2018, 129, 143–153 CrossRef CAS PubMed.
- M. N. Mangal, S. G. Salinas-Rodriguez, J. Dusseldorp, A. J. B. Kemperman, J. C. Schippers, M. D. Kennedy and W. G. J. van der Meer, Effectiveness of antiscalants in preventing calcium phosphate scaling in reverse osmosis applications, J. Membr. Sci., 2021, 623, 119090 CrossRef CAS.
- B. Cao, A. Ansari, X. Yi, D. F. Rodrigues and Y. Hu, Gypsum scale formation on graphene oxide modified reverse osmosis membrane, J. Membr. Sci., 2018, 552, 132–143 CrossRef CAS.
- T. Tong, S. Zhao, C. Boo, S. M. Hashmi and M. Elimelech, Relating Silica Scaling in Reverse Osmosis to Membrane Surface Properties, Environ. Sci. Technol., 2017, 51, 4396–4406 CrossRef CAS PubMed.
- U. Rao, A. Iddya, B. Jung, C. M. Khor, Z. Hendren, C. Turchi, T. Cath, E. M. V. Hoek, G. Z. Ramon and D. Jassby, Mineral Scale Prevention on Electrically Conducting Membrane Distillation Membranes Using Induced Electrophoretic Mixing, Environ. Sci. Technol., 2020, 54, 3678–3690 CrossRef CAS PubMed.
- T. Virtanen, S.-P. Reinikainen, M. Kögler, M. Mänttäri, T. Viitala and M. Kallioinen, Real-time fouling monitoring with Raman spectroscopy, J. Membr. Sci., 2017, 525, 312–319 CrossRef CAS.
- S. Park, S. Kim, J. Park and K. H. Cho, Real-time monitoring the spatial distribution of organic fouling using fluorescence imaging technique, J. Membr. Sci., 2020, 597, 117778 CrossRef CAS.
- Z. Cui, X. Wang, H. Ngo and G. Zhu, In-situ monitoring of membrane fouling migration and compression mechanism with improved ultraviolet technique in membrane bioreactors, Bioresour. Technol., 2022, 347, 126684 CrossRef CAS PubMed.
- X.-S. Yuan, Z.-Y. Guo, H.-Z. Geng, D. S. Rhen, L. Wang, X.-T. Yuan and J. Li, Enhanced performance of conductive polysulfone/MWCNT/PANI ultrafiltration membrane in an online fouling monitoring application, J. Membr. Sci., 2019, 575, 160–169 CrossRef CAS.
- N. Zhang, M. A. Halali and C. Lannoy, Detection of fouling on electrically conductive membranes by electrical impedance spectroscopy, Sep. Purif. Technol., 2020, 242, 116823 CrossRef CAS.
- X. Wang, M. Sun, Y. Zhao, C. Wang, W. Ma, M. S. Wong and M. Elimelech, In Situ Electrochemical Generation of Reactive Chlorine Species for Efficient Ultrafiltration Membrane Self-Cleaning, Environ. Sci. Technol., 2020, 54, 6997–7007 CrossRef CAS PubMed.
- J. H. Lee, E.-T. Yun, S.-Y. Ham, H.-S. Kim, P.-F. Sun and H.-D. Park, Electrically conductive carbon nanotube/graphene composite membrane for self-cleaning of biofouling via bubble generation, Desalination, 2022, 535, 115841 CrossRef CAS.
- G. L. Wei, J. Dong, J. Bai, Y. S. Zhao and Y. Li, Structurally Stable, Anti-fouling and Easily Renewable Reduced Graphene Oxide Membrane with Carbon Nanotube Protective Layer, Environ. Sci. Technol., 2019, 53, 11896–11903 CrossRef CAS PubMed.
- G. L. Wei, Y. S. Zhao, J. Dong, M. Gao and C. Li, Electrochemical cleaning of fouled laminar graphene membranes, Environ. Sci. Technol. Lett., 2020, 7, 773–778 CrossRef CAS.
- L. Tang, A. Iddya, X. B. Zhu, A. V. Dudchenko, W. Y. Duan, C. Turchi, J. Vanneste, T. Y. Cath and D. Jassby, Enhanced flux and electrochemical cleaning of silicate scaling on carbon nanotube-coated membrane distillation membranes treating geothermal brines, ACS Appl. Mater. Interfaces, 2017, 9, 38594–38605 CrossRef CAS PubMed.
- R. Hashaikeh, B. S. Lalia, V. Kochkodan and N. Hilal, A novel in situ membrane cleaning method using periodic electrolysis, J. Membr. Sci., 2014, 471, 149–154 CrossRef CAS.
- C. Cuevas, D. Kim, K. P. Katuri, P. Saikaly and S. P. Nunes, Electrochemically active polymeric hollow fibers based on poly(ether-b-amide)/carbon nanotubes, J. Membr. Sci., 2018, 545, 323–328 CrossRef CAS.
- Y. K. Wang, W. W. Li, G. P. Sheng, B. J. Shi and H. Q. Yu, In-situ utilization of generated electricity in an electrochemical membrane bioreactor to mitigate membrane fouling, Water Res., 2013, 47, 5794–5800 CrossRef CAS PubMed.
- K. Zhang, Y. Yang, S. Qiao, J. Zhou and X. Quan, Alternating current-enhanced carbon nanotubes hollow fiber membranes for membrane fouling control in novel membrane bioreactors, Chemosphere, 2021, 277, 130240 CrossRef CAS PubMed.
- Y. Yang, S. Qiao, M. Zheng, Q. Han, R. Wang, J. Zhou and X. Quan, Polyaniline derived carbon membrane and its in-situ membrane fouling mitigation performance in MBR based on metal-free electro-Fenton, Water Res., 2022, 219, 118564 CrossRef CAS PubMed.
- Y. Yang, K. Zhang, S. Qiao, J. Zhou and X. Quan, Performance of Alternating-Current-Enhanced Anaerobic Membrane Bioreactor: Membrane Fouling, Wastewater Treatment, and CH4 Production, ACS Sustainable Chem. Eng., 2021, 9, 15973–15982 CrossRef CAS.
- Y. Yang, S. Qiao, J. Zhou and X. Quan, Mitigating Membrane Fouling Based on In Situ ˙OH Generation in a Novel Electro-Fenton Membrane Bioreactor, Environ. Sci. Technol., 2020, 54, 7669–7676 CrossRef CAS PubMed.
- Y. Yang, M. Zheng, S. Qiao, J. Zhou, Z. Bi and X. Quan, Electro-Fenton improving fouling mitigation and microalgae harvesting performance in a novel membrane photobioreactor, Water Res., 2022, 210, 117955 CrossRef CAS PubMed.
- K. C. Zuo, H. Liu, Q. Y. Zhang, P. Liang, C. D. Vecitis and X. Huang, Enhanced performance of nitrogen-doped carbon nanotube membrane-based filtration cathode microbial fuel cell, Electrochim. Acta, 2016, 211, 199–206 CrossRef CAS.
Footnote |
† These authors contributed equally to this work. |
|
This journal is © The Royal Society of Chemistry 2023 |
Click here to see how this site uses Cookies. View our privacy policy here.