Per- and polyfluoroalkyl substances (PFAS) in white-tailed sea eagle eggs from Sweden: temporal trends (1969–2021), spatial variations, fluorine mass balance, and suspect screening†
Received
10th April 2023
, Accepted 31st July 2023
First published on 2nd August 2023
Abstract
Temporal and spatial trends of 15 per- and polyfluoroalkyl substances (PFAS) were determined in white-tailed sea eagle (WTSE) eggs (Haliaeetus albicilla) from two inland and two coastal regions of Sweden between 1969 and 2021. PFAS concentrations generally increased from ∼1969 to ∼1990s–2010 (depending on target and site) and thereafter plateaued or declined, with perfluorooctane sulfonamide (FOSA) and perfluorooctane sulfonate (PFOS) declining faster than most perfluoroalkyl carboxylic acids (PFCAs). The net result was a shift in the PFAS profile from PFOS-dominant in 1969–2010 to an increased prevalence of PFCAs over the last decade. Further, during the entire period higher PFAS concentrations were generally observed in coastal populations, possibly due to differences in diet and/or proximity to more densely populated areas. Fluorine mass balance determination in pooled samples from three of the regions (2019–2021) indicated that target PFAS accounted for the vast majority (i.e. 81–100%) of extractable organic fluorine (EOF). Nevertheless, high resolution mass-spectrometry-based suspect screening identified 55 suspects (31 at a confidence level [CL] of 1–3 and 24 at a CL of 4–5), of which 43 were substances not included in the targeted analysis. Semi-quantification of CL ≤ 2 suspects increased the identified EOF to >90% in coastal samples. In addition to showing the impact of PFAS regulation and phase-out initiatives, this study demonstrates that most extractable organofluorine in WTSE eggs is made up of known (legacy) PFAS, albeit with low levels of novel substances.
Environmental significance
The historical susceptibility of white-tailed sea eagle (WTSE) populations to effects from environmental contaminants highlights the importance of chemical exposure assessment in this species. Per- and polyfluoroalkyl substances (PFAS), which are known to accumulate in WTSE eggs, are of particular concern. Since only a handful of the several thousand known PFAS are routinely monitored, estimates of WTSE exposure to PFAS may be underestimated. The present work utilized a suite of state-of-the-art analytical techniques to investigate PFAS temporal and spatial trends in WTSE eggs. In addition to showing a decline in PFAS concentrations in eggs from throughout Sweden in more recent years (likely in response to phase-out and regulatory initiatives), we find that legacy substances account for majority of the extractable organic fluorine, despite the observation of a wide range of novel PFAS. Collectively, this work demonstrates that total PFAS exposure in WTSEs is well characterized by monitoring legacy PFAS, and that overall, exposure appears to be declining.
|
Introduction
Per- and polyfluoroalkyl substances (PFAS) are a large and diverse class of anthropogenic compounds that, with few exceptions, are defined as having at least one perfluorinated methylene (i.e. CF2) or methyl (i.e. CF3) group.1 The unique combination of lipophobicity and hydrophobicity imparted by perfluoroalkyl chains,2,3 together with the notable strength of the C–F bond,4 make PFAS desirable for use in a variety of household and industrial applications.5,6 However, PFAS are also environmentally persistent, or degrade to environmentally persistent and mobile end products (e.g. perfluoroalkyl acids; PFAAs),7 which has contributed to their global distribution in the environment, including both humans and wildlife.8–10
International efforts to reduce PFAS emissions through regulation have ramped up over the past two decades since the phase-out of perfluorooctane sulfonyl fluoride-based chemistries by the 3 M Co in 2002.11,12 Perfluorooctanesulfonic acid (PFOS) was added to the United Nations Stockholm Convention on Persistent Organic Pollutants in 2009, followed by perfluorooctanoate (PFOA) in 2019 and perfluorohexanesulfonate (PFHxS) in 2022. Inclusion of C9–C14 perfluoroalkyl carboxylates (PFCAs) in the Stockholm Convention is currently under consideration.13 In addition, a broad restriction proposal on PFAS prepared by authorities in Sweden, the Netherlands, Germany, Denmark and Norway was submitted in 2023, for consideration by the European Chemicals Agency (ECHA).14
Temporal trends in humans and biota offer a means of evaluating the success of regulatory and phase-out initiatives. Analysis of PFAS in human serum from Sweden (sampled 1996–2017) revealed declining trends for all target PFAS, beginning around the year 2000 for PFOS, and ∼2004–2009 for long-chain PFCAs.15 Declining trends have also been reported in human milk from Sweden (sampled 1972–2016), but with an earlier onset of decline for PFOS (1988) and only a few significantly declining trends for long chain PFCAs (starting ∼2000–2011).16 However, in Baltic Sea wildlife, declining trends are less commonly observed.17 A number of factors could contribute to this delayed response, including ongoing discharge of PFAS from rivers which feed into the Baltic Sea, poor water exchange with the North Sea,8,18 or simply a lag time between the phase out of PFAS-containing consumer products and reduction in wildlife exposure. Alternatively, temporal trends in wildlife may simply not extend far enough to detect a statistically significant decline. For example, PFAS time series in liver of Baltic herring and cod only extend to 2013/2014 and show increasing trends for all PFAS except perfluoroctane sulfonamide (FOSA).19,20 Likewise, long chain PFCAs measured in two freshwater species from Sweden (perch and Arctic char; 1980–2018) displayed significantly increasing concentrations of several long chain PFCAs, with a declining trend (perch; starting 1980) or no trend (char) observed for PFOS.21 Elsewhere in Europe, northern gannet eggs (United Kingdom; 1977–2014) displayed declining perfluoroalkyl sulfonic acids (PFSAs) and PFOA concentrations starting in the mid-1990s but no evidence of a decline for other long chain PFCAs.22 Collectively, it remains unclear whether all PFCAs and PFSAs are declining in European wildlife in response to international regulation of PFAS.
In addition to the inconclusive trends reported for numerous PFAS in wildlife, most exposure studies have focused on a small fraction of the >4700 PFAS estimated by the OECD.1 Recent surveys in human and environmental samples have shown that a significant fraction of the extractable organic fluorine (EOF) is unidentified,23,24 and may even be increasing.15,25,26 Accurate characterization of exposure is particularly important for high trophic level organisms, which tend to receive a large dose of contaminants via their diet and, by extension, are the most at risk of contaminant-related health effects.27,28 White-tailed sea eagles (WTSE) (Haliaeetus albicilla) are an example of an apex predator that has been impacted historically by contaminant exposure. In the early 1960s, breeding of WTSE deteriorated in the Baltic Sea region due to polychlorinated biphenyl (PCB) and dichlorodiphenyltrichloroethane (DDT) exposure, to the point of endangerment.29 While the population has since recovered, there are ongoing concerns surrounding the vulnerability of WTSE populations from exposure to more contemporary contaminants such as PFAS.30
The most recent survey of PFAS in WTSE eggs in Sweden from 1966–2010 found no evidence of declining concentrations.20 The present work builds upon this earlier study by updating the temporal and spatial trends for PFAS in WTSE eggs with samples from 2015–2021. To assess the extent to which exposure is characterized by the 15 targeted PFAS, pooled samples from the most recent years (2019–2021) were subjected to a fluorine mass balance (FMB). Thereafter, high resolution mass spectrometry-based suspect screening together with semi-quantification was used to close the fluorine mass balance. Together, this work provides a comprehensive and up-to-date picture of PFAS exposure and trends in WTSE eggs from Sweden.
Materials and methods
Sample collection
Annual surveys of WTSEs, including reproduction using non-viable eggs, have been carried out in Sweden since the 1960s.29 WTSEs have a high life expectancy and typically reside within their nesting home range throughout their life.30 The home range can vary in size due to the environment but typically has a radius of <15 km in the Baltic region.31 These factors make them ideal sentinel species for studying changes in contaminant exposure within a local/regional environment over time. Eggs analyzed in the present work were collected during late spring/early summer, 1–2 months after failure to hatch. Contents of each egg were homogenized and stored either individually (time trend analysis) or as pools (fluorine mass balance/suspect screening) in the Environmental Specimen Bank (ESB) at the Swedish Museum of Natural History. Temporal trends of PFAAs and FOSA in 83 of these samples (1960s–2010) were reported previously in Faxneld et al. (2016).20 In the present work we analyzed an additional 75 samples collected more recently (2015–2021; Table S1†) and used the combined dataset (152 samples total, after outlier removal; 1969–2021) to re-evaluate spatio-temporal trends. Collectively, the entire dataset covers four regions of Sweden: Northern Inland (Lapland), Southern Inland, Gulf of Bothnia, and Baltic Proper (Fig. 1). In addition, a snapshot of fluorine mass balance and suspect screening for three pooled samples from the most recent years (2019–2021) is included for the Baltic Proper, Gulf of Bothnia and Northern Inland (Table S2†).
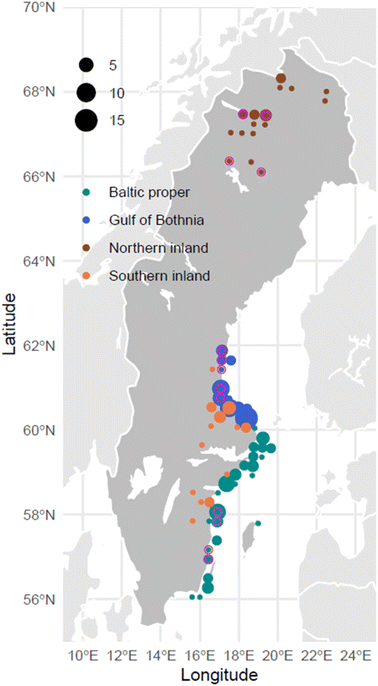 |
| Fig. 1 Map of WTSE egg sampling locations (1969–2021). Pink circles indicate individuals which were included in regional pooled samples (2019–2021 only; Southern Inland not included), which were subjected to fluorine mass balance determination and suspect screening. Circle size represent the number of samples collected from each sampling location across all years of sampling. | |
Standards and reagents
A total of 15 PFAS were targeted in the present study, which included 10 perfluoroalkyl carboxylic acids (PFCAs; C6–15), 4 perfluoroalkyl sulfonic acids (PFSAs; C4,6,8,10) and FOSA. All native and isotopically-labelled standards were purchased from Wellington Labs (Guelph, Canada) and are listed in Table S3.† Certified reference material (CRM) BCR-461 (Fluorine in Clay), was purchased from Sigma Aldrich. Remaining standards and reagents are provided in the ESI.†
Targeted analysis of individuals (1969–2021)
Approximately 1 g of individual egg samples (n = 1 per individual) were extracted using a modified version of a protein precipitation method described by Powley et al.32 (see ESI† for details). Internal standards (ISs; 1 ng each; Table S3†) and recovery standards (13C8-PFOA and 13C8-PFOS) were added before and after extraction, respectively, and all extracts were stored at −20 °C until the day of analysis. Instrumental analysis was carried out on a Waters Acquity UPLC coupled to a Waters Xevo triple quadrupole mass spectrometer. Details of these methods have been previously published15,23 and are described in the ESI (text and Tables S3 and S4†). Analytes without a corresponding, exactly matched isotopically labelled standard were semi-quantified using the IS with the closest retention time (Table S3†). In cases where branched isomers were observed, concentrations were estimated using the calibration curve for the corresponding linear isomer. The linear and branched isomers were summed to produce an overall concentration for the selected analyte. Method quantification limits (MQLs) are displayed in Table S1† and were determined using concentration of lowest calibration standard available.
Fluorine mass balance and suspect screening in pooled samples (2019–2021)
The FMB workflow is depicted in Fig. S1.† Briefly, pooled egg homogenate (n = 3 per sampling region) was extracted in the same manner as individuals, but without adding IS prior to extraction. The resulting extract was split into two separate portions: one portion was fortified with 4 mM ammonium acetate and ISs (1 ng each; Table S3†) and analyzed by UPLC-high resolution mass spectrometry using a combined target/suspect screening method (details below), while the second portion was analyzed for EOF by combustion ion chromatography (CIC).23 CIC-based total fluorine (TF) analysis was also performed directly on egg homogenates (200 mg). Further details of CIC instrumental parameters are provided in the ESI (text and Table S5†).
The combined target/suspect screening method applied to pooled samples was based on previous studies,15,23 details of which are provided in the ESI (text and Tables S4 and S6†). Briefly, extracts were injected onto a Dionex Ultimate 3000 Ultrahigh performance liquid chromatography (Thermo Scientific) coupled to a Q Exactive HF Orbitrap mass spectrometer (Thermo Scientific). Measurements were carried out in full scan (200–1200 m/z) data dependent MS2 acquisition mode. The inclusion list consisted of 173 PFAS previously determined in marine mammals from the peer-reviewed literature (Table S7†). Thermo-Fisher TraceFinder™ software was used for data processing and analysis of these 173 suspects, with acquired MS2 data assessed manually against spectra from the aforementioned literature.23,33–35 Each substance was then assigned a confidence level (CL; 1–5) according to the Schymanski scale (see details in the ESI†),36 with analytes with a CL ≤ 2 undergoing semi-quantification using the nearest eluting homologue with an available authentic standard calibration curve.
Quality control
Targeted analysis and suspect screening.
Quality control procedures for individual samples collected prior to 2015 are reported elsewhere.20 For individual samples collected from 2015 to 2021, accuracy and precision were assessed via replicate spike/recovery experiments involving chicken egg homogenate (1 g; n = 13) fortified with 10–40 ng of a native standards (C6–C14 PFCAs, C4,6,8,10 PFSAs and FOSA). These samples were analyzed together with unfortified samples. Accuracy was reasonable for most targets (76–88%; stdev 3.3–14%; Table S8†), with the exception of perfluorotridecanoate (PFTrDA), PFTeDA, and perfluorodecane sulfonate (PFDS) which displayed lower and more variable recoveries (40–63%; stdev 23–28%; Table S8†). The same procedure was used for fluorine mass balance experiments (10 ng individual PFAS, n = 3), with the exception that internal standards were only added after extraction and to a portion of the extract intended for targeted PFAS analysis. Percent recoveries of individual target PFAS were again reasonable (58–114%; stdev 3.4–12%; Table S9†). To validate the suspect screening method, the spiked egg homogenate was analyzed by HRMS. We first confirmed that the instrument would successfully trigger an MS2 experiment when spiked substances were matched to the inclusion list. Secondly, we visually evaluated the quality of acquired MS2 spectra in standards spiked into the egg homogenate to those prepared in solvent. Finally, method blanks (n = 3) that were processed together with real samples were examined for the presence of any suspects. In the case of detection in the method blanks, an average of the peak area was subtracted from each individual replicate in each regional pooled sample.
EOF and TF analysis.
The portion of extract not fortified with IS was subjected to CIC analysis, which revealed an EOF recovery of 55 ± 8%, which was slightly lower than the recoveries obtained for individual PFAS on a fluorine-equivalent basis. Inorganic fluorine recovery tests were also done through spiking of sodium fluoride (250 ng; n = 3) and displayed concentrations below quantification limits, showing that the extraction procedure eliminated inorganic fluorine present in the samples. Throughout the EOF instrumental analysis, standards of PFOS were analyzed regularly to monitor combustion efficiency and instrumental drift. Average recoveries were 95 ± 9%, indicating acceptable accuracy and precision. Replicates of certified reference material (CRM) BCR-461 (fluorine in clay) were also analyzed to evaluate accuracy and precision of TF measurements and displayed good agreement (92 ± 1%), with reference values. Finally, procedural blanks analyzed alongside both individual (2019–21 only) and pooled samples, displayed concentrations below the LOQ for all targets.
Statistical analysis
Eggs were in varying states of desiccation upon sampling. To account for varying water content, desiccation indices (DIs) were calculated using the formula DI = (W − S)/V where W = weight (g) of the egg after sampling, S = dry shell weight (g) and V = internal volume (ml) of the egg.37 DIs start at 1.00 (freshly laid egg) and decrease with increasing extent of desiccation (Table S1†). Thereafter, measured PFAS concentrations were multiplied by the DI in order to approximate the original fresh weight concentration (which is commonly used for reporting PFAS in bird eggs).20,38–40 This procedure has been previously applied and validated for use in WTSE eggs.20,29,37 In some samples collected after 2000 where DIs were unavailable an average DI from 2000–2010 was used (0.800 ± 0.115), while samples prior to 2000 were removed when no DI was available (due to higher variability in the DI between samples).
Statistical analyses were conducted in R (v. 4.0.3).41 We tested potential outliers using Grubb's test and based on the results excluded four observations prior to the data analysis. The final dataset contained 152 samples collected between 1969 and 2021 (Fig. 1). For determination of sum concentrations and relative distribution of PFAS, values below LOQ were replaced with 0. For evaluating time series change points (CP), trends for individual PFAS, and yearly averages, concentrations below LOQ were substituted with LOQ/√2 (Table S1†). CP analysis for PFAS time series was carried out by fitting two-piece linear regression splines to yearly average log-concentrations, with the CP represented by the unknown join point estimated by least squares.42,43 A P-value of <0.05 was considered statistically significant. In comparison to e.g. Nyberg et al.,16 who fits separate log-linear regression before and after the CP, this has the advantage of forcing the trend-lines to meet at the CP year. In order to test the null hypothesis (that there is no structural change in the time series) we used the supF test with a p value approximated by parametric bootstrap.44,45 The full fitting process is implemented as an R package, lmcp, freely available at https://github.com/mskoldSU/lmcp. To investigate the presence of temporal trends, we used log-linear regression on the whole time series, on the period from the CP to the last year of the time series (CPyear–2021), and on the last 15 years (2007–2021). The slope of the log-linear regression represents the yearly average percent increase or decrease of the time series.
Results and discussion
PFAS concentrations and profiles
Across the entire study period (1969–2021), sum target PFAS concentrations (∑15PFAS; DI corrected) were highest in the Baltic Proper (avg. 304 ng g−1; range: 11.2–950 ng g−1), followed by Gulf of Bothnia (Avg. 146 ng g−1; range: 7.50–372 ng g−1), Southern Inland (Avg. 121 ng g−1; range: 38.8–312 ng g−1), and Northern Inland (Avg. 55.9 ng g−1; range: 13.6–155 ng g−1). The higher concentrations in the coastal and southern populations are likely associated with diet (coastal/marine-based diets can be expected to contain higher levels of PFAS compared to inland/terrestrial), and proximity to more densely populated areas in Sweden (as seen for surface water and fish46,47), and can also be attributed to the sedentary nature of the territorial adults.48
PFOS generally dominated the PFAS profile across all regions, accounting for as much as 98% of the ∑15PFAS concentration in the earliest years (1969–79) but displayed a decrease in the relative contribution in more recent years (Fig. 2). In Northern Inland, for example, PFOS accounted for <40% of ∑15PFAS concentrations from 2015–2021 (Fig. 2). This decrease is caused by a more rapid decline of PFOS concentrations starting in the 1990s compared to PFCAs (discussed further in the section on temporal trends). Similar observations were made in gull eggs from Western Europe,49 where a shift in profile from PFOS-dominant to PFCA-dominant was attributed to simultaneous phase-out of PFOS and ongoing production of long chain PFCAs over the last 20 years.50 The major PFCA homologues across all regions over most of the studied period were typically perfluorononanoate (PFNA), perfluoroundecanoate (PFUnDA), and perfluorotetradecanoate (PFTeDA), with the exception of a few cases where perfluorodecanoate (PFDA) exceeded the concentrations of PFNA. The prevalence of odd-chain length homologues (i.e. PFNA, PFUnDA, perfluorotridecanoate [PFTrDA]) over even chain-length homologues (PFOA, PFDA, PFTeDA) was noted previously across all 4 regions20 and could be due to atmospheric oxidation of even chain length precursors (e.g. x
:
2 FTOHs) to approximately equal proportions of even and odd-chain length PFCAs, followed by bioaccumulation of the odd chain homologues (related to preferential binding of long chain PFCAs to egg yolk proteins).9,51,52 A predominance of long chain length PFCAs has also been noted in bird eggs from the UK,53 Indian Ocean,54 Western Canada,53 Norway,39 and the Canadian Arctic.55
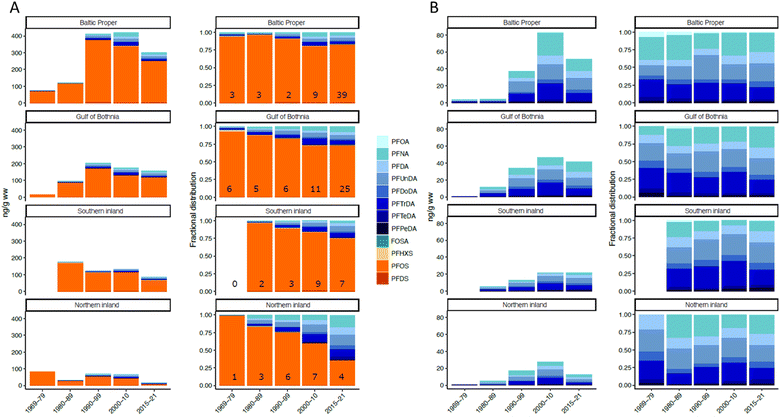 |
| Fig. 2 Absolute and relative concentrations for major targeted PFAS (A) and PFCAs only (B), from the four sampling regions within Sweden across 1969–2021 sampling periods. Profiles are split approximately by decade. Numbers stated inside bars in panel A represent the number of individuals within the stated year range. Concentrations for targets not included here (i.e. PFHxA, PFHpA and PFBS) due to low detection frequencies (<17%) are provided in Table S1.† | |
PFCA temporal trends
While statistically significant increasing trends in ∑PFCA concentrations (i.e. ∑C8–C15 PFCAs) occurred at all four locations when considering the entire time period (i.e., 1969–2021, Fig. 3), statistically significant CPs were also observed in 2003 (Baltic Proper), 1992 (Gulf of Bothnia) and 2008 (Northern Inland), followed by either non-statistically significant or significantly declining trends for ∑PFCAs. The exception was for Southern Inland, which did not display a statistically significant change point for ∑PFCA concentrations. In general, ∑PFCA trends are representative of trends for all individual PFCAs detected in the present work (Table 1), albeit with some variability in the year and statistical significance of the change point between locations (note that time trends of C6 and C7 PFCAs were not investigated due to <17% detection frequency). The range of change points reported here (i.e. 1992–2009) overlaps with change points for individual PFCAs observed in human milk (1984–2011)16 and serum (2000–2009)15 from Sweden, as well as the US phase out of long chain PFCAs and their precursors, which began in 2006 and aimed to be completed by 2015.50 Despite being a North American initiative, the US phaseout was adopted by several major global manufacturers outside the US,56 and can reasonably be assumed to have initiated a reduction of PFCA emissions in Europe. Formal regulation of PFCAs in Europe did not start until 2012, when C11–C14 PFCAs (and then PFOA in 2013) were included in the candidate list of substances of very high concern under REACH.56 Most recently (2023), a broad restriction proposal for the entire PFAS class has been submitted for consideration by the European Chemicals Agency (ECHA).14
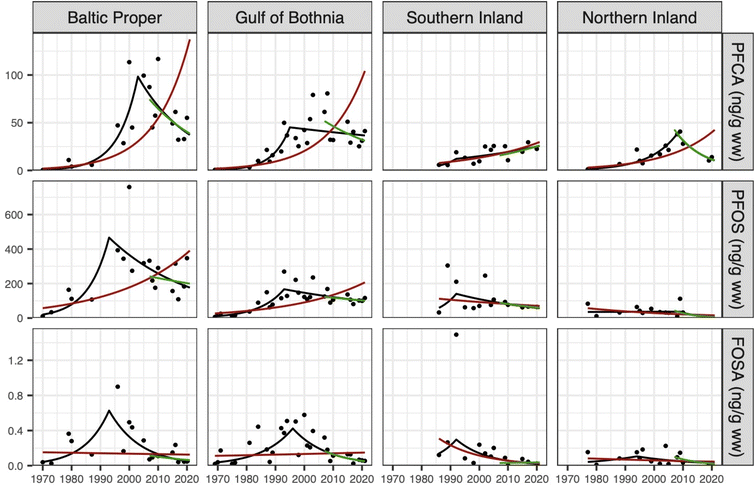 |
| Fig. 3 Change-point graphs for the summed concentration of PFCAs, PFOS and FOSA across the four sampling regions. Each dot represents a yearly geometric average. Change-point trends are provided in black, while log-linear trends for the whole period and last 15 years are shown in red and green, respectively. Statistical significance is provided in Table 1. | |
Table 1 Table displaying PFAS change points and trends (% per year) over various time periods. “∑PFCAs” is the sum of individual PFCAs shown below. PFHxA, PFHpA, PFBS were excluded from analysis due to <17% detection frequencies. Asterisk denotes significant change-point/trend with p-value <0.05 (*), <0.01 (**), < 0.001 (***)
PFAS |
Area |
CP year |
Trend CP–2019 (%) |
Trend all (%) |
Trend last 15 years (%) |
∑PFCAs |
Baltic Proper |
2003* |
−5.2* |
8.7*** |
−4.6 |
Gulf of Bothnia |
1995*** |
−0.8 |
8.1*** |
−3.6 |
Southern Inland |
1992 |
2.6 |
3.9* |
3.7 |
Northern Inland |
2008*** |
−9.6* |
6.2* |
−9.6* |
PFOA |
Baltic Proper |
2000 |
−4.1 |
2.8* |
−0.9 |
Gulf of Bothnia |
1989 |
0.1 |
2.7*** |
−2.5 |
Southern Inland |
1992 |
−1 |
−0.6 |
−0.3 |
Northern Inland |
2009* |
−9.2*** |
−1.4 |
−9.4 |
PFNA |
Baltic Proper |
1992* |
−1 |
9.5*** |
−3.4 |
Gulf of Bothnia |
1993* |
1.2 |
9.3*** |
0.7 |
Southern Inland |
2014 |
24.2 |
2.7 |
12.3 |
Northern Inland |
1992*** |
−0.4 |
6.1* |
−3.4 |
PFDA |
Baltic Proper |
2003* |
−3.2 |
10.7*** |
−2.6 |
Gulf of Bothnia |
1993*** |
1.3 |
8.8*** |
−1.1 |
Southern Inland |
1992 |
4.2* |
4.8* |
6.8 |
Northern Inland |
2008*** |
−7.9* |
6.3*** |
−8.1* |
PFUnDA |
Baltic Proper |
1996* |
−1 |
10.3*** |
−3 |
Gulf of Bothnia |
1995*** |
−1.9 |
8.2*** |
−4.2 |
Southern Inland |
1992 |
2.5 |
4.3* |
3.6 |
Northern Inland |
2008*** |
−11* |
6.1* |
−10.7* |
PFDoDA |
Baltic proper |
2003* |
−1.6 |
9.8*** |
−1.4 |
Gulf of Bothnia |
1994*** |
0.3 |
7.8*** |
−3.3 |
Southern Inland |
1992 |
3.4 |
5.1* |
4.4 |
Northern Inland |
2009* |
−9.7* |
6.8*** |
−11* |
PFTrDA |
Baltic Proper |
2003*** |
−7.4* |
7.7*** |
−9.5* |
Gulf of Bothnia |
2003* |
−8.2*** |
7.9*** |
−8.8* |
Southern Inland |
2005* |
−4.2 |
3.2 |
−2.7 |
Northern Inland |
2009* |
−16.5*** |
7.4* |
−16.5* |
PFTeDA |
Baltic Proper |
2010* |
−12* |
6.7*** |
−7.2* |
Gulf of Bothnia |
1994* |
0.1 |
7.5*** |
−4.9 |
Southern Inland |
2005 |
−0.2 |
4.5* |
1.4 |
Northern Inland |
2010 |
−10.7* |
8.3*** |
−11.9* |
PFPeDA |
Baltic Proper |
2012*** |
−28.7*** |
7.5*** |
−13* |
Gulf of Bothnia |
2005*** |
−9.5* |
7.8*** |
−9.7 |
Southern Inland |
2015 |
−21.4 |
6* |
−1.4 |
Northern Inland |
2009* |
−11.9* |
9.3*** |
−12.6* |
PFHxS |
Baltic Proper |
1993* |
−4.7* |
3* |
−7.3* |
Gulf of Bothnia |
1998* |
−1.9 |
2.4* |
−3.1 |
Southern Inland |
1992 |
−3.3 |
−1.3 |
−0.1 |
Northern Inland |
2009* |
−24.3*** |
−4 |
−24.4* |
PFOS |
Baltic Proper |
1993 |
−3.4* |
3.8* |
−1.3 |
Gulf of Bothnia |
1993* |
−1.9* |
4*** |
−1.4 |
Southern Inland |
1992 |
−3.1 |
−1.3 |
−2.9 |
Northern Inland |
2009 |
−15.1* |
−2.9 |
−13.5 |
PFDS |
Baltic Proper |
1997* |
−9.9*** |
8.3* |
−9.8 |
Gulf of Bothnia |
1995* |
−6.9* |
5.6* |
−8.4* |
Southern Inland |
2006* |
−14* |
−1.2 |
−13.4* |
Northern Inland |
2010 |
−11.9 |
0.4 |
−11.4 |
FOSA |
Baltic Proper |
1993* |
−8.8*** |
−0.4 |
−4.2 |
Gulf of Bothnia |
1996* |
−8.1*** |
0.6 |
−8.2 |
Southern Inland |
1992 |
−8.9* |
−6.8* |
1.6 |
Northern Inland |
1994 |
−4.8 |
−1.4 |
−10.5 |
Declining PFCA concentrations in bird eggs are reported infrequently in the peer-reviewed literature, likely because prior studies have not extended far enough in time to observe such trends. For example, a recent study in eggs of the Northern gannet from two colonies in the UK observed a peak in PFOA concentrations around ∼1998–2002 but no evidence of a decline in C9–C11 and C13 PFCAs from 1988–1992 until 2014 (the last year of sampling).22 Two other studies involving bird eggs from the Canadian west coast and Great Lakes region (∼1990 to ∼2011) also reported increasing ∑PFCA concentrations but limited evidence of declining trends.39,53
PFSA and FOSA temporal trends
Across the entire time period (i.e. 1969–2021), PFOS displayed statistically significant increasing trends for the Baltic Proper and Gulf of Bothnia, but non-statistically significant declining trends for Northern and Southern Inland. Significant CPs for PFOS were only found in the Gulf of Bothnia (1993). However, this aligned well with the non-significant CPs for the Baltic Proper (1993) and Southern Inland (1992; Table 1). Similar CPs were observed for FOSA (1992–1996; all 4 locations), PFHxS (1992–1998; 3 locations) and PFDS (1995 and 1997; 2 locations), of which the Baltic Proper and Gulf of Bothnia were consistently significant. Time trends of perfluorobutane sulfonate (PFBS) were not investigated due to low detection frequency (<2%). While these CPs occur nearly a decade earlier than the voluntary phase-out of perfluorooctane sulfonyl fluoride-based products by the 3 M Co in 2000, they align well with prior trends of PFOS and FOSA in human milk from Stockholm, Sweden (CPs of 1988 and 1984, respectively),16 PFOS in bird eggs from two colonies in the UK (CPs of 1994 and 1998),22 and two in Norway (leveling off observed ∼1993),39 and with trends of a major PFOS-precursor (the perfluorooctane sulfonamide ethanol-based phosphate diester; SAmPAP diester) in sediment cores from Tyrifjorden, Norway (peak observed in the early 1980s).57 The observed decline in PFOS concentrations in a wide range of species and locations globally in the late 80s–90s was previously suggested to be linked to a shift away from SAmPAP-based formulations by the 3 M Co., prior to the phase-out of all perfluorooctane sulfonyl fluoride-based products in 2000.58 After the CP year, all regions except Southern Inland showed significantly decreasing trends (1.9–15.1% per year, Table 1). The slopes are similar (Fig. 3) but non-significant when considering only the last 15 years (1.3–13.5% per year). This suggests that PFOS is being removed from the system as an effect of the phase-out but also highlights the need for long time series to quantify the removal due to high inter-annual variability in observations.
Fluorine mass balance of pooled samples
Concentrations for target PFAS, EOF, TF on a fluorine equivalent basis are provided in Tables S10 and S11.† TF and EOF concentrations were highest in the Baltic Proper (402 ± 57.2 and 213 ± 9.12 ng F g−1, respectively), followed by the Gulf of Bothnia (301 ± 66.6 ng F g−1 and 116 ± 11.1 ng F g−1, respectively), and Northern Inland (32.7 ± 4.38 and 11.3 ± 6.35 ng F g−1, respectively), consistent with ∑15PFAS concentrations (Fig. 4; panel A). Similar observations have been made for other POPs (DDT and its metabolites, PCB and mercury) and appear to align with population density, which is highest in the Baltic Proper, and much lower in northern regions.29 EOF mass balances for the Northern Inland and Baltic Proper regions were closed (p > 0.05; 1-sided t-test), with ≥95% of the EOF accounted for by target PFAS. In comparison, the fluorine mass balance in the Gulf of Bothnia region was slightly open (p < 0.05; 1-sided t-test), with 81% of EOF attributed to target PFAS. Across all sampling regions, EOF accounted for 34–53% of the TF concentrations, leaving a large fraction of the TF unidentified. The identity of this fraction is unclear but may include non-extractable organic- and/or inorganic fluorinated substances.
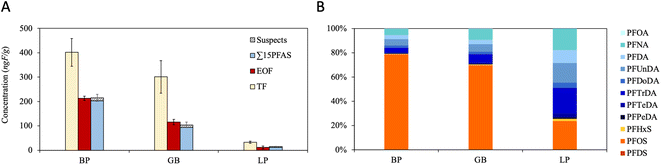 |
| Fig. 4 (A) FMB detailing total fluorine (TF), extractable organofluorine (EOF), targeted PFAS (∑15PFAS) and suspect concentrations (ng F g−1) in pooled samples. PFHxA, PFHpA, and FOSA were not detected across all three regions and are therefore not shown. Error bars represent standard deviation of replicate analysis (n = 3). (B) Normalised PFAS profiles of pooled samples. BP = Baltic Proper; GB = Gulf of Bothnia; LP = Northern Inland. | |
EOF concentrations in WTSE eggs from the present study (11–213 ng F g−1) are lower than those observed previously for common guillemots in Sweden (419–649 ng F g−1), but similar to those of black guillemot, common guillemot and Northern fulmar from several Nordic regions (Greenland, Iceland, and Faroe Islands; <40–287 ng F g−1).59 They also fall within the range of EOF concentrations reported in bird eggs from a wide range of species from Norway (glaucous gull, common eider, black-legged kittiwake, European common gull, European shag; ∼40–400 ng F g−1).60 The percentage of EOF accounted for by known PFAS reported here (81–99%) also overlapped with prior observations in Swedish common guillemots eggs (67–87% identified) and black guillemot and Northern fulmar from the Faroe islands (58–102% identified),59 but are considerably higher than EOF mass balances in black guillemot eggs from Greenland (9% identified), common guillemot eggs from Iceland (33% identified), and eggs from various bird species from Norway (6–60% identified).60 Geography, species, and year of sampling can all reasonably be assumed to influence observed EOF concentrations, and by extension, the fluorine mass balance. It was also previously shown that extraction procedure can influence EOF concentrations.61 While the present study used an ACN extraction procedure, others have employed a MeOH extraction procedure with EnviCarb clean-up,60 or an ion-pairing method.59
Finally, while EOF concentrations in WTSE eggs from the Baltic Proper were close to average concentrations in liver of WTSEs from Norway (221 ng F g−1), the percentage of EOF accounted for by known PFAS in liver was much lower (17% identified).60 While we cannot rule out the influence of geography, year of sampling, and extraction procedure on this result, another possibility is that the unidentified EOF observed in liver is not as readily transferred to the eggs as target PFAS.62 This hypothesis remains tentative and requires further investigation in the future.
Suspect screening of pooled samples
In addition to the 15 PFAS included in the targeted analysis, 40 additional PFAS were detected by suspect screening, resulting in a total of 55 PFAS from 15 classes observed in WTSE eggs (Table 2). The following 6 classes were identified at ≤CL 2, and were semi-quantified if they were not previously measured/included in the target analysis (Table S12†): (1) PFCAs, (2) PFSAs, (3) n
:
3 fluorotelomer carboxylic acids (n
:
3 FTCAs), (4) n
:
2 fluorotelomer sulfonic acids (n
:
2 FTSAs), (5) perfluoroalkyl sulfonamides (FASAs), and (6) cyclic/unsaturated PFSAs. The remaining 9 classes were identified with lower confidence (CL 3–5) and have therefore not been semi-quantified. This includes: (7) perfluorinated N-heterocycles, (8) single-hydrogenated PFCAs (H-PFCAs), (9) single-hydrogenated PFSAs (H-PFSAs), (10) ether PFCAs (PFECAs), (11) ether PFSAs, (12) multiple ether PFSAs, (13) Cl-substituted PFCAs, (14) cyclic/unsaturated ether PFSAs, and finally, (15) an unknown class lacking structural information, which was previously identified in biota.23 A detailed explanation of the procedure for identifying all substances is provided below (summarized in Table 2), and their contribution to the overall F mass balance following semi-quantification (for CL 1 and 2 substances) is shown in Fig. 4.
Table 2 Summary of all 15 PFAS classes and 55 individual substances detected by suspect screening along with information supporting their assignment. Heat map indicates relative abundance for each substance across the three regions from highest (dark red) to non-detect (white). Bold font indicates analyte with highest abundance relative to others in the same homologous series. N/F indicates MS2 was triggered with no matching fragments. * = tentative structures. RT = retention time; CL = confidence level; BP = Baltic Proper; GB = Gulf of Bothnia; LP = Northern Inland
PFCAs and PFSAs.
In addition to the 14 PFAAs included in the targeted analysis, perfluoroheptane sulfonate (PFHpS) and perfluorononane sulfonate (PFNS) were identified through suspect screening, with the former confirmed using an authentic standard (CL 1). While the signal for PFNS was sufficient to trigger an MS2 scan, product ion peak areas were very low (<20
000 cps). Nevertheless, its elution between PFOS and PFDS, and exact mass within 1.5 ppm of theoretical provided considerable confidence in this assignment (CL = 2b). All PFAAs displayed the highest abundance in the Baltic Proper, followed by Gulf of Bothnia and Northern Inland (Table 2).
n
:
3 FTCAs.
A total of five n
:
3 FTCAs ([CnF2n−5O2H4]−, 10 ≤ n ≤ 14) were detected through suspect screening, one of which (7
:
3 FTCA; CL 1) was confirmed with an authentic standard. 8
:
3 and 9
:
3 FTCAs (CL 2a) were identified using a combination of exact mass, matching diagnostic MS2 fragments to literature,23,33,34 and increasing relative retention time with chain length. The remaining two homologues (10
:
3 and 11
:
3 FTCA) displayed exact masses within 3 ppm of theoretical and relative retention times identical to our prior measurements in marine mammals23 (where MS2 data were collected); nevertheless, due to a lack of fragmentation data, a lower confidence was assigned to these homologues (CL 2b). Sum n
:
3 FTCA concentrations, determined semi-quantitatively using the 7
:
3 FTCA standard, accounted for 79 and 43% of the unidentified EOF for the Baltic Proper and Gulf of Bothnia regions, respectively. Unlike most other PFAS identified through suspect screening, n
:
3 FTCAs frequently occurred at higher concentrations in the Gulf of Bothnia than in the other regions (Table 2). Despite 8
:
3 FTCA appearing to be the dominant congener (followed closely by 7
:
3 FTCA), this result should be interpreted cautiously due to potential errors arising from semi-quantification. FTCAs, in particular the 7
:
3 homologue, were previously reported in liver of marine mammals from the Northern Hemisphere, and bird eggs from Svalbard.23,33,62,63 While intentional production of n
:
3 FTCAs is unclear, these substances are known to be among the major stable transformation products of fluorotelomer alcohols, which are high production volume chemicals produced globally.64,65
n
:
2 FTSAs.
Three FTSAs (6
:
2, 8
:
2 and 10
:
2) were detected and confirmed with authentic standards (CL = 1). Sum FTSA concentrations accounted for up to 10% of the unidentified EOF across all regions. n
:
2 FTSAs have been previously detected in marine mammals in the Northern Hemisphere23 and in the South China Sea,66 as well as in European fauna at various trophic levels.67 FTSAs and their derivatives are known to be used in AFFF,68 and have been reported at particularly high concentrations in groundwater impacted by fire-fighting activities.69
FASAs.
FASAs ([CnF2n+1HNO2S]−, n = 4, 5, 6, 8) occurred at very low concentrations in WTSE eggs, resulting in a lack of product ion data, and also hampering attempts at semi-quantifying homologues. Most FASA congeners were identified at CL 2b, based on exact mass and retention time data (both relative retention times but also similarities to our previous analyses where MS2 data were collected23). FOSA, which was below detection limits when measured by targeted analysis, was detectable during suspect screening and could be confirmed using an authentic standard (CL 1). FASAs are relatively stable intermediates in the degradation of N-alkyl substituted perfluoroalkyl sulfonamides and sulfonamido alcohols to PFSAs.58 They are widely reported in global wildlife, and usually dominated by the C8 homologue (FOSA).23,33,66,70–72
Cyclic/unsaturated PFSAs.
Among the cyclic/unsaturated PFSA class ([CnF2n−1SO3]−, n = 8–12) only a single target (perfluoroethylcyclohexane sulfonate; PFECHS) was confirmed at CL 1. Others within the homologous series were ascribed a CL of 2b based on exact mass and retention time (including comparisons to prior datasets23). Semi quantification of cyclic/unsaturated PFSAs with a standard of PFECHS revealed low concentrations (0.008–3.001 ng F g−1), dominated by PFECHS (∼3 ng F g−1). This cyclic PFSA has been reported previously in polar bear and ringed seal samples from the Arctic,9,73 as well as in Baltic biota and sea water samples.74,75 Time trends of PFECHS in human serum indicate that concentrations have been declining since the year 2000.15
Perfluorinated N-heterocycles.
A single homologue was identified in this class ([CnF2n−3N2O]−, n = 17), and while product ion data were available, only a single diagnostic fragment could be matched to data from the literature.34 This substance was therefore assigned a CL of 3 and was not quantified. Reports of perfluorinated N-heterocycles are rare; to the best of our knowledge this class of PFAS has only been reported once previously, in fish from Tangxun Lake, China.76 Homologues ranging from C9–C18 (plus isomers) were detected in that work, among which C12 was dominant. Sources of these substances remain unclear, but it was noted that similar structures have been used in the pharmaceutical industry.76
H-PFCAs/H-PFSAs.
Both H-PFCAs ([CnF2n−2HO2]−; n = 5–9) and H-PFSAs ([CnHF2nO3S]−; n = 8 and 10) were detected, among which C10 and C8 were the dominant homologues, respectively, based on peak areas. Owing to a lack of fragmentation data; the position of the H-atom on the fluorinated chain of these classes could not be deduced; consequently, a CL of 4 was assigned to all H-PFCAs and H-PFSAs. Surprisingly, H-PFCAs occurred readily in Northern Inland, despite the low target PFAS concentrations and EOF in this region. Hydrogen-substituted PFAAs have been previously reported in surface water,77,78 sediment,17 human blood79 and biota,33 but the dominant congener has varied depending on matrix (e.g. H-PFDA and H-PFDoDA were dominant in blood,79 while H-PFHxA was dominant in sediment17).
Ether PFCAs/PFSAs.
Ether PFCAs and PFSAs are structurally similar to PFAAs but contain an ether linkage within the perfluorinated chain (i.e. [CnF2n−1O3]− for ether PFCAs and [CnF2n+1SO4]− for ether PFSAs). Only a single ether PFCA was detected in the present work (n = 9), while 4 ether PFSA homologues were measured (n = 4–7), among which C8 was the dominant congener. Due to a lack of fragmentation data, the position of the ether-linkage or possible branching could not be confirmed; consequenetly, a CL of 4 was assigned to all ether PFAAs. As with the n
:
3 FTCAs, the monoether PFSAs appear to be present in considerable amounts within the Gulf of Bothnia pool. A growing number of studies have reported ether-based PFAAs in the environment, including in fish and marine mammals,23,33,76,80 possibly due to the increasing use of ether-based PFAAs by industry.81
Multiple ether PFSAs.
The structure for multiple ether PFSAs involves an additional ether functional group substituted for a fluorinated carbon atom from the ether PFSAs ([CnF2n+1O5S]−, n = 9). The observation of a single congener, and no accompanying MS2 data means that this assignment remains highly tentative (CL = 4). As with monoether-PFCAs/PFSAs, the position of the ether linkages and of possible branching in the carbon chain are also unknown. Nevertheless, this class of PFAS is known to be produced as a by-product of fluoropolymer manufacturing and has been detected downstream from fluoropolymer manufacturing sites.82
Cl-substituted PFCAs.
Cl-substituted PFCAs ([CnF2n−2O2Cl]−, n = 8–12) are structurally analogous to H-PFCAs with the substituted hydrogen atom replaced with chlorine. Despite the detection of 5 congeners, no MS2 data were collected, thus all assignments were given a CL of 4. Nevertheless, this class of PFAS has been previously reported in industrial wastewater,78,83 fish,76 and human serum in China.84
Cyclic/unsaturated ether PFSAs.
For the cyclic/unsaturated ether PFSAs (CnF2n−1SO4]−, n = 8), there was insufficient data available to determine a final structure of this suspect. While only a single homologue with no M2 data, there is prior evidence for detection of this substance in both polar bear serum, as well the liver of a variety of marine mammals,23 albeit in low amounts.
Unknown class.
The final class detected through the suspect screening ([CnF2n−9H9NO4S]−, n = 12–16) was previously reported in marine mammals,23 but to the best of our knowledge has not yet been identified. While diagnostic fragments were available for almost all of the homologues in this series, a suitable structure could not be proposed, and consequently, a CL of 5 was ascribed to the entire class.
Implications for the health of WTSEs
The susceptibility of WTSEs to contaminant-induced population crashes in the past highlights the importance of accurately characterizing chemical exposure in this species.85 In the present work, the highest ∑15PFAS concentrations observed in WTSE eggs (950 ng g−1) are just below the predicted no effect concentration for PFOS in egg yolk (1000 ng g−1).40 Nevertheless, decreased hatchability in chicken eggs was previously reported at doses as low as 100 ng PFOS per g egg,86 which is below the average ∑15PFAS concentrations observed at three of the four sites in the present work. This is highly concerning, in particular considering the wide range of additional/novel PFAS that were detectable in WTSE eggs, few of which have been characterized for health effects.87,88 Moreover, we cannot rule out that the considerable gap between EOF and TF concentrations observed at all 3 locations is made up (at least in part) by non-extractable organofluorine substances. Systematic investigations into the effects of extraction procedure on EOF are clearly needed, along with effects assessment for a wider suite of PFAS.
Conflicts of interest
There are no conflicts of interest to declare.
Acknowledgements
This work was supported through funding from the Swedish Environmental Protection Agency, (project number NV-06943-21) and the Swedish Research Council Formas (Grant numbers 2018-00801 and 2018-00423). ML and JPB acknowledge support from the European Union's Horizon 2020 research and innovation programme under Marie Skłodowska-Curie Action Grant Agreement 860665 (PERFORCE3). Dr Peter Hellström and Elisabeth Nyberg (Swedish Museum of Natural History) are thanked for helpful discussions.
References
- Z. Wang, A. M. Buser, I. T. Cousins, S. Demattio, W. Drost, O. Johansson, K. Ohno, G. Patlewicz, A. M. Richard, G. W. Walker, G. S. White and E. Leinala, A New OECD Definition for Per- and Polyfluoroalkyl Substances, Environ. Sci. Technol., 2021, 55(23), 15575–15578, DOI:10.1021/acs.est.1c06896.
- J. Glüge, M. Scheringer, I. T. Cousins, J. C. DeWitt, G. Goldenman, D. Herzke, R. Lohmann, C. A. Ng, X. Trier and Z. Wang, An Overview of the Uses of Per- and Polyfluoroalkyl Substances (PFAS), Environ. Sci.: Processes Impacts, 2020, 22(12), 2345–2373, 10.1039/D0EM00291G.
- B. C. Crone, T. F. Speth, D. G. Wahman, S. J. Smith, G. Abulikemu, E. J. Kleiner and J. G. Pressman, Occurrence of Per- and Polyfluoroalkyl Substances (PFAS) in Source Water and Their Treatment in Drinking Water, Crit. Rev. Environ. Sci. Technol., 2019, 49(24), 2359–2396, DOI:10.1080/10643389.2019.1614848.
- I. T. Cousins, J. C. DeWitt, J. Glüge, G. Goldenman, D. Herzke, R. Lohmann, C. A. Ng, M. Scheringer and Z. Wang, The High Persistence of PFAS Is Sufficient for Their Management as a Chemical Class, Environ. Sci.: Processes Impacts, 2020, 22(12), 2307–2312, 10.1039/D0EM00355G.
- T. Ruan and G. Jiang, Analytical Methodology for Identification of Novel Per- and Polyfluoroalkyl Substances in the Environment, TrAC, Trends Anal. Chem., 2017, 95, 122–131, DOI:10.1016/j.trac.2017.07.024.
- Q. Kang, F. Gao, X. Zhang, L. Wang, J. Liu, M. Fu, S. Zhang, Y. Wan, H. Shen and J. Hu, Nontargeted Identification of Per- and Polyfluoroalkyl Substances in Human Follicular Fluid and Their Blood-Follicle Transfer, Environ. Int., 2020, 139, 105686, DOI:10.1016/j.envint.2020.105686.
- Z. Wang, J. C. DeWitt, C. P. Higgins and I. T. Cousins, A Never-Ending Story of Per- and Polyfluoroalkyl Substances (PFASs)?, Environ. Sci. Technol., 2017, 51(5), 2508–2518, DOI:10.1021/acs.est.6b04806.
- X. C. Hu, D. Q. Andrews, A. B. Lindstrom, T. A. Bruton, L. A. Schaider, P. Grandjean, R. Lohmann, C. C. Carignan, A. Blum, S. A. Balan, C. P. Higgins and E. M. Sunderland, Detection of Poly- and Perfluoroalkyl Substances (PFASs) in U.S. Drinking Water Linked to Industrial Sites, Military Fire Training Areas, and Wastewater Treatment Plants, Environ. Sci. Technol. Lett., 2016, 3(10), 344–350, DOI:10.1021/acs.estlett.6b00260.
- D. Muir, R. Bossi, P. Carlsson, M. Evans, A. De Silva, C. Halsall, C. Rauert, D. Herzke, H. Hung, R. Letcher, F. Rigét and A. Roos, Levels and Trends of Poly- and Perfluoroalkyl Substances in the Arctic Environment – An Update, Emerging Contam., 2019, 5, 240–271, DOI:10.1016/j.emcon.2019.06.002.
- J. M. Graber, C. Alexander, R. J. Laumbach, K. Black, P. O. Strickland, P. G. Georgopoulos, E. G. Marshall, D. G. Shendell, D. Alderson, Z. Mi, M. Mascari and C. P. Weisel, Per- and Polyfluoroalkyl Substances (PFAS) Blood Levels after Contamination of a Community Water Supply and Comparison with 2013-14 NHANES, J. Exposure Sci. Environ. Epidemiol., 2019, 29(2), 172–182, DOI:10.1038/s41370-018-0096-z.
- T. Stahl, D. Mattern and H. Brunn, Toxicology of Perfluorinated Compounds, Environ. Sci. Eur., 2011, 23(1), 38, DOI:10.1186/2190-4715-23-38.
- E. M. Sunderland, X. C. Hu, C. Dassuncao, A. K. Tokranov, C. C. Wagner and J. G. Allen, A Review of the Pathways of Human Exposure to Poly- and Perfluoroalkyl Substances (PFASs) and Present Understanding of Health Effects, J. Exposure Sci. Environ. Epidemiol., 2019, 29(2), 131–147, DOI:10.1038/s41370-018-0094-1.
- W. Xu, X. Wang and Z. Cai, Analytical Chemistry of the Persistent Organic Pollutants Identified in the Stockholm Convention: A Review, Anal. Chim. Acta, 2013, 790, 1–13, DOI:10.1016/j.aca.2013.04.026.
- All news - ECHA, https://echa.europa.eu/sv/-/echa-publishes-pfas-restriction-proposal, accessed 2023-06-24.
- L. T. Miaz, M. M. Plassmann, I. Gyllenhammar, A. Bignert, O. Sandblom, S. Lignell, A. Glynn and J. P. Benskin, Temporal Trends of Suspect- and Target-per/Polyfluoroalkyl Substances (PFAS), Extractable Organic Fluorine (EOF) and Total Fluorine (TF) in Pooled Serum from First-Time Mothers in Uppsala, Sweden, 1996–2017, Environ. Sci.: Processes Impacts, 2020, 22(4), 1071–1083, 10.1039/C9EM00502A.
- E. Nyberg, R. Awad, A. Bignert, C. Ek, G. Sallsten and J. P. Benskin, Inter-Individual, Inter-City, and Temporal Trends of per- and Polyfluoroalkyl Substances in Human Milk from Swedish Mothers between 1972 and 2016, Environ. Sci.: Processes Impacts, 2018, 20(8), 1136–1147, 10.1039/C8EM00174J.
- X. Song, R. Vestergren, Y. Shi, J. Huang and Y. Cai, Emissions, Transport, and Fate of Emerging Per- and Polyfluoroalkyl Substances from One of the Major Fluoropolymer Manufacturing Facilities in China, Environ. Sci. Technol., 2018, 52(17), 9694–9703, DOI:10.1021/acs.est.7b06657.
- B. Gustafsson, Interaction between Baltic Sea and North Sea, Dtsch. Hydrogr. Z., 1997, 49(2), 165–183, DOI:10.1007/BF02764031.
- L. Schultes, O. Sandblom, K. Broeg, A. Bignert and J. P. Benskin, Temporal Trends (1981–2013) of Per- and Polyfluoroalkyl Substances and Total Fluorine in Baltic Cod (Gadus Morhua), Environ. Toxicol. Chem., 2020, 39(2), 300–309, DOI:10.1002/etc.4615.
- S. Faxneld, U. Berger, B. Helander, S. Danielsson, A. Miller, E. Nyberg, J.-O. Persson and A. Bignert, Temporal Trends and Geographical Differences of Perfluoroalkyl Acids in Baltic Sea Herring and White-Tailed Sea Eagle Eggs in Sweden, Environ. Sci. Technol., 2016, 50(23), 13070–13079, DOI:10.1021/acs.est.6b03230.
-
A. L. Soerensen and S. Faxneld, Per- and Polyfluoroalkyl Substances (PFAS) within the Swedish Monitoring Programme for Contaminants in Marine Biota, Swedish Museum of Natural History, Stockholm, Sweden, 2023 Search PubMed.
- M. G. Pereira, S. Lacorte, L. A. Walker and R. F. Shore, Contrasting Long Term Temporal Trends in Perfluoroalkyl Substances (PFAS) in Eggs of the Northern Gannet (Morus Bassanus) from Two UK Colonies, Sci. Total Environ., 2021, 754, 141900, DOI:10.1016/j.scitotenv.2020.141900.
- K. M. Spaan, C. van Noordenburg, M. M. Plassmann, L. Schultes, S. Shaw, M. Berger, M. P. Heide-Jørgensen, A. Rosing-Asvid, S. M. Granquist, R. Dietz, C. Sonne, F. Rigét, A. Roos and J. P. Benskin, Fluorine Mass Balance and Suspect Screening in Marine Mammals from the Northern Hemisphere, Environ. Sci. Technol., 2020, 54(7), 4046–4058, DOI:10.1021/acs.est.9b06773.
- L. Schultes, C. van Noordenburg, K. M. Spaan, M. M. Plassmann, M. Simon, A. Roos and J. P. Benskin, High Concentrations of Unidentified Extractable Organofluorine Observed in Blubber from a Greenland Killer Whale (Orcinus Orca), Environ. Sci. Technol. Lett., 2020, 7(12), 909–915, DOI:10.1021/acs.estlett.0c00661.
- R. Aro, U. Eriksson, A. Kärrman and L. W. Y. Yeung, Organofluorine Mass Balance Analysis of Whole Blood Samples in Relation to Gender and Age, Environ. Sci. Technol., 2021, 55(19), 13142–13151, DOI:10.1021/acs.est.1c04031.
- L. W. Y. Yeung, S. A. Mabury, L. W. Y. Yeung and S. A. Mabury, Are Humans Exposed to Increasing Amounts of Unidentified Organofluorine?, Environ. Chem., 2015, 13(1), 102–110, DOI:10.1071/EN15041.
- M. Haukås, U. Berger, H. Hop, B. Gulliksen and G. W. Gabrielsen, Bioaccumulation of Per- and Polyfluorinated Alkyl Substances (PFAS) in Selected Species from the Barents Sea Food Web, Environ. Pollut., 2007, 148(1), 360–371, DOI:10.1016/j.envpol.2006.09.021.
- K. Borgå, A. T. Fisk, P. F. Hoekstra and D. C. G. Muir, Biological and Chemical Factors of Importance in the Bioaccumulation and Trophic Transfer of Persistent Organochlorine Contaminants in Arctic Marine Food Webs, Environ. Toxicol. Chem., 2004, 23(10), 2367–2385, DOI:10.1897/03-518.
- B. Helander, M. Olsson and L. Reutergårdh, Residue Levels of Organochlorine and Mercury Compounds in Unhatched Eggs and the Relationships to Breeding Success in White-Tailed Sea Eagles Haliaeetus Albicilla in Sweden, Holarctic Ecol., 1982, 5(4), 349–366 CAS.
-
B. Helander and T. Stjernberg, White-Tailed Eagle (Haliaeetus Albicilla), in Birds of the World, Cornell Lab of Ornithology, 2002, DOI:10.2173/bow.whteag.01.
- C. Ekblad, H. Tikkanen, S. Sulkava and T. Laaksonen, Diet and Breeding Habitat Preferences of White-Tailed Eagles in a Northern Inland Environment, Polar Biol., 2020, 43(12), 2071–2084, DOI:10.1007/s00300-020-02769-1.
- C. R. Powley, S. W. George, T. W. Ryan and R. C. Buck, Matrix Effect-Free Analytical Methods for Determination of Perfluorinated Carboxylic Acids in Environmental Matrixes, Anal. Chem., 2005, 77(19), 6353–6358, DOI:10.1021/ac0508090.
- H. Barrett, X. Du, M. Houde, S. Lair, J. Verreault and H. Peng, Suspect and Nontarget Screening Revealed Class-Specific Temporal Trends (2000–2017) of Poly- and Perfluoroalkyl Substances in St. Lawrence Beluga Whales, Environ. Sci. Technol., 2021, 55(3), 1659–1671, DOI:10.1021/acs.est.0c05957.
- Y. Liu, L. A. D'Agostino, G. Qu, G. Jiang and J. W. Martin, High-Resolution Mass Spectrometry (HRMS) Methods for Nontarget Discovery and Characterization of Poly- and per-Fluoroalkyl Substances (PFASs) in Environmental and Human Samples, TrAC, Trends Anal. Chem., 2019, 121, 115420, DOI:10.1016/j.trac.2019.02.021.
- A. Wang, D. P. Abrahamsson, T. Jiang, M. Wang, R. Morello-Frosch, J.-S. Park, M. Sirota and T. Woodruff, J. Suspect Screening, Prioritization, and Confirmation of Environmental Chemicals in Maternal-Newborn Pairs from San Francisco, Environ. Sci. Technol., 2021, 55(8), 5037–5049, DOI:10.1021/acs.est.0c05984.
- E. L. Schymanski, J. Jeon, R. Gulde, K. Fenner, M. Ruff, H. P. Singer and J. Hollender, Identifying Small Molecules via High Resolution Mass Spectrometry: Communicating Confidence, Environ. Sci. Technol., 2014, 48(4), 2097–2098, DOI:10.1021/es5002105.
- B. Helander, A. Olsson, A. Bignert, L. Asplund and K. Litzén, The Role of DDE, PCB, Coplanar PCB and Eggshell Parameters for Reproduction in the White-Tailed Sea Eagle (Haliaeetus Albicilla) in Sweden, Ambio, 2002, 31(5), 386–403, DOI:10.1579/0044-7447-31.5.386.
- K. E. Holmström and U. Berger, Tissue Distribution of Perfluorinated Surfactants in Common Guillemot (Uria Aalge) from the Baltic Sea, Environ. Sci. Technol., 2008, 42(16), 5879–5884, DOI:10.1021/es800529h.
-
V. Jonathan, B. Urs and G. W. Gabrielsen, Trends of Perfluorinated Alkyl Substances in Herring Gull Eggs from Two Coastal Colonies in Northern Norway: 1983−2003, ACS Publications, DOI:10.1021/es070723j.
- J. L. Newsted, P. D. Jones, K. Coady and J. P. Giesy, Avian Toxicity Reference Values for Perfluorooctane Sulfonate, Environ. Sci. Technol., 2005, 39(23), 9357–9362, DOI:10.1021/es050989v.
- R Core Team – European Environment Agency. R: A language and environment for statistical computing, R Foundation for Statistical Computing, Vienna, Austria, https://www.R-project.org, accessed 2022-11-05.
- D. J. Hudson, Fitting Segmented Curves Whose Join Points Have to Be Estimated, J. Am. Stat. Assoc., 1966, 61(316), 1097–1129, DOI:10.1080/01621459.1966.10482198.
- R. Chappell, Fitting Bent Lines to Data, with Applications to Allometry, J. Theor. Biol., 1989, 138(2), 235–256, DOI:10.1016/S0022-5193(89)80141-9.
- D. W. K. Andrews and W. Ploberger, Optimal Tests When a Nuisance Parameter Is Present Only Under the Alternative, Econometrica, 1994, 62(6), 1383–1414, DOI:10.2307/2951753.
- D. W. K. Andrews, Tests for Parameter Instability and Structural Change With Unknown Change Point, Econometrica, 1993, 61(4), 821–856, DOI:10.2307/2951764.
- M. A. Nguyen, K. Wiberg, E. Ribeli, S. Josefsson, M. Futter, J. Gustavsson and L. Ahrens, Spatial Distribution and Source Tracing of Per- and Polyfluoroalkyl Substances (PFASs) in Surface Water in Northern Europe, Environ. Pollut., 2017, 220, 1438–1446, DOI:10.1016/j.envpol.2016.10.089.
-
A. L. Soerensen and S. FaxneldPer- and Polyfluoroalkyl Substances (PFAS) within the Swedish Monitoring Programme for Contaminants in Marine Biota, 2023 Search PubMed.
- A. Bignert and B. O. Helander, Monitoring of Contaminants and Their Effects on the Common Guillemot and the White-Tailed Sea Eagle, J. Ornithol., 2015, 156(1), 173–185, DOI:10.1007/s10336-015-1240-3.
- P. Colomer-Vidal, A. Bertolero, C. Alcaraz, E. Garreta-Lara, F. J. Santos and S. Lacorte, Distribution and Ten-Year Temporal Trends (2009–2018) of Perfluoroalkyl Substances in Gull Eggs from Spanish Breeding Colonies, Environ. Pollut., 2022, 293, 118555, DOI:10.1016/j.envpol.2021.118555.
- M. Land, C. A. de Wit, A. Bignert, I. T. Cousins, D. Herzke, J. H. Johansson and J. W. Martin, What Is the Effect of Phasing out Long-Chain per- and Polyfluoroalkyl Substances on the Concentrations of Perfluoroalkyl Acids and Their Precursors in the Environment? A Systematic Review, Environ. Evid., 2018, 7(1), 4, DOI:10.1186/s13750-017-0114-y.
- C. M. Butt, D. C. G. Muir and S. A. Mabury, Biotransformation Pathways of Fluorotelomer-Based Polyfluoroalkyl Substances: A Review, Environ. Toxicol. Chem., 2014, 33(2), 243–267, DOI:10.1002/etc.2407.
- W. A. Gebbink and R. J. Letcher, Comparative Tissue and Body Compartment Accumulation and Maternal Transfer to Eggs of Perfluoroalkyl Sulfonates and Carboxylates in Great Lakes Herring Gulls, Environ. Pollut., 2012, 162, 40–47, DOI:10.1016/j.envpol.2011.10.011.
- A. Miller, J. E. Elliott, K. H. Elliott, S. Lee and F. Cyr, Temporal Trends of Perfluoroalkyl Substances (PFAS) in Eggs of Coastal and Offshore Birds: Increasing PFAS Levels Associated with Offshore Bird Species Breeding on the Pacific Coast of Canada and Wintering near Asia, Environ. Toxicol. Chem., 2015, 34(8), 1799–1808, DOI:10.1002/etc.2992.
- V. van der Schyff, N. S. C. Kwet Yive, A. Polder, N. C. Cole and H. Bouwman, Perfluoroalkyl Substances (PFAS) in Tern Eggs from St. Brandon's Atoll, Indian Ocean, Mar. Pollut. Bull., 2020, 154, 111061, DOI:10.1016/j.marpolbul.2020.111061.
- B. M. Braune and R. J. Letcher, Perfluorinated Sulfonate and Carboxylate Compounds in Eggs of Seabirds Breeding in the Canadian Arctic: Temporal Trends (1975–2011) and Interspecies Comparison, Environ. Sci. Technol., 2013, 47(1), 616–624, DOI:10.1021/es303733d.
- Assessment, U. E. N. C. for E, Working towards a global emission inventory of PFASS: focus on PFCAS - status quo and the way forward, https://hero.epa.gov/hero/index.cfm/reference/details/reference_id/4774679, accessed 2023-06-28.
- H. A. Langberg, H. P. H. Arp, G. D. Breedveld, G. A. Slinde, Å. Høiseter, H. M. Grønning, M. Jartun, T. Rundberget, B. M. Jenssen and S. E. Hale, Paper Product Production Identified as the Main Source of Per- and Polyfluoroalkyl Substances (PFAS) in a Norwegian Lake: Source and Historic Emission Tracking, Environ. Pollut., 2021, 273, 116259, DOI:10.1016/j.envpol.2020.116259.
- J. W. Martin, B. J. Asher, S. Beesoon, J. P. Benskin and M. S. Ross, PFOS or PreFOS? Are Perfluorooctane Sulfonate Precursors (PreFOS) Important Determinants of Human and Environmental Perfluorooctane Sulfonate (PFOS) Exposure?, J. Environ. Monit., 2010, 12(11), 1979–2004, 10.1039/c0em00295j.
-
A. Kärrman; T. Wang; R. Kallenborn; A. M. Langseter; S. M. Grønhovd; E. M. Ræder; J. L. Lyche; L. Yeung; F. Chen; U. Eriksson; R. Aro and F. Fredriksson, PFASs in the Nordic Environment : Screening of Poly- and Perfluoroalkyl Substances (PFASs) and Extractable Organic Fluorine (EOF) in the Nordic Environment, Nordisk Ministerråd, 2019 Search PubMed.
- D. Herzke, V. Nikiforov, L. W. Y. Yeung, B. Moe, H. Routti, T. Nygård, G. W. Gabrielsen and L. Hanssen, Targeted PFAS Analyses and Extractable Organofluorine – Enhancing Our Understanding of the Presence of Unknown PFAS in Norwegian Wildlife, Environ. Int., 2023, 171, 107640, DOI:10.1016/j.envint.2022.107640.
- A.-M. Kaiser, R. Aro, A. Kärrman, S. Weiss, C. Hartmann, M. Uhl, M. Forsthuber, C. Gundacker and L. W. Y. Yeung, Comparison of Extraction Methods for Per- and Polyfluoroalkyl Substances (PFAS) in Human Serum and Placenta Samples—Insights into Extractable Organic Fluorine (EOF), Anal. Bioanal. Chem., 2021, 413(3), 865–876, DOI:10.1007/s00216-020-03041-5.
- W. Jouanneau, D.-J. Léandri-Breton, A. Corbeau, D. Herzke, B. Moe, V. A. Nikiforov, G. W. Gabrielsen and O. Chastel, A Bad Start in Life? Maternal Transfer of Legacy and Emerging Poly- and Perfluoroalkyl Substances to Eggs in an Arctic Seabird, Environ. Sci. Technol., 2022, 56(10), 6091–6102, DOI:10.1021/acs.est.1c03773.
- K. Lee, J. J. Alava, P. Cottrell, L. Cottrell, R. Grace, I. Zysk and S. Raverty, Emerging Contaminants and New POPs (PFAS and HBCDD) in Endangered Southern Resident and Bigg's (Transient) Killer Whales (Orcinus Orca): In Utero Maternal Transfer and Pollution Management Implications, Environ. Sci. Technol., 2023, 57(1), 360–374, DOI:10.1021/acs.est.2c04126.
- H. Nilsson, A. Kärrman, A. Rotander, B. van Bavel, G. Lindström and H. Westberg, Biotransformation of Fluorotelomer Compound to Perfluorocarboxylates in Humans, Environ. Int., 2013, 51, 8–12, DOI:10.1016/j.envint.2012.09.001.
- M. C. Huang, V. G. Robinson, S. Waidyanatha, A. L. Dzierlenga, M. J. DeVito, M. A. Eifrid, S. T. Gibbs and C. R. Blystone, Toxicokinetics of 8:2 Fluorotelomer Alcohol (8:2-FTOH) in Male and Female Hsd:Sprague Dawley SD Rats after Intravenous and Gavage Administration, Toxicol. Rep., 2019, 6, 924–932, DOI:10.1016/j.toxrep.2019.08.009.
- Q. Wang, Y. Ruan, L. Jin, X. Zhang, J. Li, Y. He, S. Wei, J. C. W. Lam and P. K. S. Lam, Target, Nontarget, and Suspect Screening and Temporal Trends of Per- and Polyfluoroalkyl Substances in Marine Mammals from the South China Sea, Environ. Sci. Technol., 2021, 55(2), 1045–1056, DOI:10.1021/acs.est.0c06685.
- A. Androulakakis, N. Alygizakis, G. Gkotsis, M.-C. Nika, V. Nikolopoulou, E. Bizani, E. Chadwick, A. Cincinelli, D. Claßen, S. Danielsson, R. W. R. J. Dekker, G. Duke, N. Glowacka, H. A. H. Jansman, O. Krone, T. Martellini, P. Movalli, S. Persson, A. Roos, E. O'Rourke, U. Siebert, G. Treu, N. W. van den Brink, L. A. Walker, R. Deaville, J. Slobodnik and N. S. Thomaidis, Determination of 56 Per- and Polyfluoroalkyl Substances in Top Predators and Their Prey from Northern Europe by LC-MS/MS, Chemosphere, 2022, 287, 131775, DOI:10.1016/j.chemosphere.2021.131775.
- K. A. Barzen-Hanson, S. C. Roberts, S. Choyke, K. Oetjen, A. McAlees, N. Riddell, R. McCrindle, P. L. Ferguson, C. P. Higgins and J. A. Field, Discovery of 40 Classes of Per- and Polyfluoroalkyl Substances in Historical Aqueous Film-Forming Foams (AFFFs) and AFFF-Impacted Groundwater, Environ. Sci. Technol., 2017, 51(4), 2047–2057, DOI:10.1021/acs.est.6b05843.
- C. Baduel, J. F. Mueller, A. Rotander, J. Corfield and M.-J. Gomez-Ramos, Discovery of Novel Per- and Polyfluoroalkyl Substances (PFASs) at a Fire Fighting Training Ground and Preliminary Investigation of Their Fate and Mobility, Chemosphere, 2017, 185, 1030–1038, DOI:10.1016/j.chemosphere.2017.06.096.
- W. A. Gebbink, R. Bossi, F. F. Rigét, A. Rosing-Asvid, C. Sonne and R. Dietz, Observation of Emerging Per- and Polyfluoroalkyl Substances (PFASs) in Greenland Marine Mammals, Chemosphere, 2016, 144, 2384–2391, DOI:10.1016/j.chemosphere.2015.10.116.
- C. Dassuncao, X. C. Hu, X. Zhang, R. Bossi, M. Dam, B. Mikkelsen and E. M. Sunderland, Temporal Shifts in Poly- and Perfluoroalkyl Substances (PFASs) in North Atlantic Pilot Whales Indicate Large Contribution of Atmospheric Precursors, Environ. Sci. Technol., 2017, 51(8), 4512–4521, DOI:10.1021/acs.est.7b00293.
- A. O. De Silva, J. M. Armitage, T. A. Bruton, C. Dassuncao, W. Heiger-Bernays, X. C. Hu, A. Kärrman, B. Kelly, C. Ng, A. Robuck, M. Sun, T. F. Webster and E. M. Sunderland, PFAS Exposure Pathways for Humans and Wildlife: A Synthesis of Current Knowledge and Key Gaps in Understanding, Environ. Toxicol. Chem., 2021, 40(3), 631–657, DOI:10.1002/etc.4935.
- R. J. Letcher, A. D. Morris, M. Dyck, E. Sverko, E. J. Reiner, D. A. D. Blair, S. G. Chu and L. Shen, Legacy and New Halogenated Persistent Organic Pollutants in Polar Bears from a Contamination Hotspot in the Arctic, Hudson Bay Canada, Sci. Total Environ., 2018, 610–611, 121–136, DOI:10.1016/j.scitotenv.2017.08.035.
- H. Joerss, C. Apel and R. Ebinghaus, Emerging Per- and Polyfluoroalkyl Substances (PFASs) in Surface Water and Sediment of the North and Baltic Seas, Sci. Total Environ., 2019, 686, 360–369, DOI:10.1016/j.scitotenv.2019.05.363.
- C. A. de Wit, R. Bossi, R. Dietz, A. Dreyer, S. Faxneld, S. E. Garbus, P. Hellström, J. Koschorreck, N. Lohmann, A. Roos, U. Sellström, C. Sonne, G. Treu, K. Vorkamp, B. Yuan and I. Eulaers, Organohalogen Compounds of Emerging Concern in Baltic Sea Biota: Levels, Biomagnification Potential and Comparisons with Legacy Contaminants, Environ. Int., 2020, 144, 106037, DOI:10.1016/j.envint.2020.106037.
- Y. Liu, M. Qian, X. Ma, L. Zhu and J. W. Martin, Nontarget Mass Spectrometry Reveals New Perfluoroalkyl Substances in Fish from the Yangtze River and Tangxun Lake, China, Environ. Sci. Technol., 2018, 52(10), 5830–5840, DOI:10.1021/acs.est.8b00779.
- M. Awchi, W. A. Gebbink, B. J. A. Berendsen, J. P. Benskin and S. P. J. van Leeuwen, Development, Validation, and Application of a New Method for the Quantitative Determination of Monohydrogen-Substituted Perfluoroalkyl Carboxylic Acids (H–PFCAs) in Surface Water, Chemosphere, 2022, 287, 132143, DOI:10.1016/j.chemosphere.2021.132143.
- Y. Liu, A. D. S. Pereira and J. W. Martin, Discovery of C5–C17 Poly- and Perfluoroalkyl Substances in Water by In-Line SPE-HPLC-Orbitrap with In-Source Fragmentation Flagging, Anal. Chem., 2015, 87(8), 4260–4268, DOI:10.1021/acs.analchem.5b00039.
- Y. Li, N. Yu, L. Du, W. Shi, H. Yu, M. Song and S. Wei, Transplacental Transfer of Per- and Polyfluoroalkyl Substances Identified in Paired Maternal and Cord Sera Using Suspect and Nontarget Screening, Environ. Sci. Technol., 2020, 54(6), 3407–3416, DOI:10.1021/acs.est.9b06505.
- Y. Liu, E. S. Richardson, A. E. Derocher, N. J. Lunn, H.-J. Lehmler, X. Li, Y. Zhang, J. Y. Cui, L. Cheng and J. W. Martin, Hundreds of Unrecognized Halogenated Contaminants Discovered in Polar Bear Serum, Angew. Chem., Int. Ed., 2018, 57(50), 16401–16406, DOI:10.1002/anie.201809906.
- W. A. Gebbink, L. van Asseldonk and S. P. J. van Leeuwen, Presence of Emerging Per- and Polyfluoroalkyl Substances (PFASs) in River and Drinking Water near a Fluorochemical Production Plant in the Netherlands, Environ. Sci. Technol., 2017, 51(19), 11057–11065, DOI:10.1021/acs.est.7b02488.
- C. Zhang, Z. R. Hopkins, J. McCord, M. J. Strynar and D. R. U. Knappe, Fate of Per- and Polyfluoroalkyl Ether Acids in the Total Oxidizable Precursor Assay and Implications for the Analysis of Impacted Water, Environ. Sci. Technol. Lett., 2019, 6(11), 662–668, DOI:10.1021/acs.estlett.9b00525.
- Y. Wang, N. Yu, X. Zhu, H. Guo, J. Jiang, X. Wang, W. Shi, J. Wu, H. Yu and S. Wei, Suspect and Nontarget Screening of Per- and Polyfluoroalkyl Substances in Wastewater from a Fluorochemical Manufacturing Park, Environ. Sci. Technol., 2018, 52(19), 11007–11016, DOI:10.1021/acs.est.8b03030.
- L. Li, N. Yu, X. Wang, W. Shi, H. Liu, X. Zhang, L. Yang, B. Pan, H. Yu and S. Wei, Comprehensive Exposure Studies of Per- and Polyfluoroalkyl Substances in the General Population: Target, Nontarget Screening, and Toxicity Prediction, Environ. Sci. Technol., 2022, 56(20), 14617–14626, DOI:10.1021/acs.est.2c03345.
- B. Helander, A. Bignert and L. Asplund, Using Raptors as Environmental Sentinels: Monitoring the White-Tailed Sea Eagle Haliaeetus Albicilla in Sweden, AMBIO J. Hum. Environ., 2008, 37(6), 425–431, DOI:10.1579/0044-7447(2008)37[425:URAESM]2.0.CO;2.
- E. D. Molina, R. Balander, S. D. Fitzgerald, J. P. Giesy, K. Kannan, R. Mitchell and S. J. Bursian, Effects of Air Cell Injection of Perfluorooctane Sulfonate before Incubation on Development of the White Leghorn Chicken (Gallus Domesticus) Embryo, Environ. Toxicol. Chem., 2006, 25(1), 227–232, DOI:10.1897/04-414R.1.
- S. Gaballah, A. Swank, J. R. Sobus, X. M. Howey, J. Schmid, T. Catron, J. McCord, E. Hines, M. Strynar and T. Tal, Evaluation of Developmental Toxicity, Developmental Neurotoxicity, and Tissue Dose in Zebrafish Exposed to GenX and Other PFAS, Environ. Health Perspect., 2020, 128(4), 47005, DOI:10.1289/EHP5843.
- N. Sheng, R. Cui, J. Wang, Y. Guo, J. Wang and J. Dai, Cytotoxicity of Novel Fluorinated Alternatives to Long-Chain Perfluoroalkyl Substances to Human Liver Cell Line and Their Binding Capacity to Human Liver Fatty Acid Binding Protein, Arch. Toxicol., 2018, 92(1), 359–369, DOI:10.1007/s00204-017-2055-1.
|
This journal is © The Royal Society of Chemistry 2023 |