Ecotoxicological effect of enrofloxacin on Spirulina platensis and the corresponding detoxification mechanism†
Received
5th July 2022
, Accepted 19th November 2022
First published on 24th November 2022
Abstract
Enrofloxacin is a widely used antibiotic targeting DNA gyrase and has become the commonly detected micropollutant in aquatic environments. Thus, the potential toxicity of enrofloxacin to Spirulina platensis which is a kind of prokaryote similar to Gram-negative bacteria has been hypothesized. However, little is known about the toxicity and degradation mechanism of enrofloxacin during the growth process of Spirulina platensis. Herein, the biomass accumulation of Spirulina platensis was stimulated to 115% of the control group by 0.1 mg L−1 enrofloxacin (10th day), which could be removed probably through the metabolism. Further increasing the enrofloxacin level to 5.0 mg L−1 almost inhibited the growth and remediation ability of Spirulina platensis for 35 days. Environmental stress also caused the variations of photosynthetic pigments (chlorophyll a and carotenoids) and primary biocomponents (proteins, lipids, and carbohydrates), reflecting the adaptation of Spirulina platensis for handling the negative effects of enrofloxacin. The detoxification mechanism was studied by identifying the degradation products of enrofloxacin, suggesting the occurrence of dealkylation and oxidation reactions primarily at the piperazine group. The decreased antimicrobial activity was confirmed by the reduced binding affinity of degradation products with enzymes. The obtained results could help us understand the role of enrofloxacin in the growth of Spirulina platensis, thus providing great support for employing Spirulina platensis in risk assessment and hazard reduction.
Environmental significance
Antibiotics including enrofloxacin and other fluoroquinolones have gained increasing attention as emerging environmental pollutants, thus requiring a sustainable and ecological approach without massive consumption of energy and chemicals for not only evaluating and reducing their hazards but also, more importantly, for producing valuable resources. Herein, Spirulina platensis showed specific sensitivity to enrofloxacin, along with the obvious variations of its biomass, photosynthetic pigments, and primary biocomponents. Removal of enrofloxacin was also observed in the culture of growing Spirulina platensis, displaying the reduced antimicrobial potencies of the corresponding degradation products. All these results could lay a foundation for further use of Spirulina platensis in evaluating ecological risks and reducing environmental hazards.
|
Introduction
Enrofloxacin is a broad-spectrum antimicrobial agent belonging to the fluoroquinolone drug class that has been widely employed in human therapy and livestock production.1 Owing to its extensive usage, enrofloxacin is inevitably released into aquatic environments and is thus frequently detected in water and wastewater.2 It was reported that 4.24 μg L−1 of enrofloxacin was detected in river water, and a surprisingly high enrofloxacin concentration up to 0.9 mg L−1 was detected in wastewater effluents.3,4 Previous studies have demonstrated that exposure to enrofloxacin may have adverse effects on non-target species and even induce the development of antibiotic resistant bacteria/genes.5,6 Consequently, it is of great importance to develop a reliable and effective mean for evaluating and reducing ecological risks in aquatic environments.7
Microalgae are a group of photoautotrophic microorganisms and have attracted much attention for their increasing applications in energy and environmental fields.8 As primary producers, microalgae play a fundamental role in the food chains of aquatic ecosystems and have been used to assess ecotoxicity due to their high sensitivity to xenobiotic pollutants.9,10 It is found that microalgae could grow well in wastewater and promote the transformation of nitrogen and phosphorus pollutants into biomass resources.11,12 More importantly, some recent studies have shown that microalgae may offer a certain degree of remediation capability towards emerging pollutants.13 Hence, it is hypothesized that microalgae-based techniques have the potential to become a promising solution for both risk assessment and pollution remediation.14
Among various microalgal species, Spirulina platensis is one of the most important prokaryotic microalgae belonging to cyanobacteria and has been used by humans for a long time.15 This is due to the fact that Spirulina platensis has high nutritional value, containing protein up to approximately 60% of its dry weight and other essential ingredients such as amino acids, vitamins, minerals, and unsaturated fatty acids.16 In recent years, Spirulina platensis was also found to be remarkably sensitive to aquatic pollutants including pesticides, antibiotics, and endocrine disruptors.17,18 However, there is little information concerning the ecotoxicological effect of fluoroquinolone antibiotics (e.g. enrofloxacin) on Spirulina platensis, which might be more sensitive than other algae owing to their prokaryotic characteristics.19
In this work, the toxicity of enrofloxacin on Spirulina platensis was evaluated at different contamination levels. The inhibition and protection phenomena were studied by determining the growth of Spirulina platensis and the removal of enrofloxacin. The corresponding mechanisms were studied by examining the variations of photosynthetic pigments (chlorophyll a and carotenoids) and primary biocomponents (proteins, lipids, and carbohydrates). Moreover, the detoxification process was investigated by identifying the degradation products and evaluating their antimicrobial activities. All these results would provide an in-depth insight into the role of enrofloxacin in the growth of Spirulina platensis, thus paving the way for applying Spirulina platensis in risk assessment and hazard reduction.
Materials and methods
Chemicals and materials
Enrofloxacin (>98.0% purity) was obtained from Sigma-Aldrich Corporation (St. Louis, MO, USA). Solvents in HPLC grade including methanol, acetonitrile, and chloroform were purchased from Thermo Fisher Scientific (Waltham, MA, USA). All other chemicals were of analytical grade and provided by Sinopharm Chemical Reagent Co., Ltd. (Shanghai, China). The studied microalgal strain, Spirulina platensis FACHB-314, was supplied by Institute of Hydrobiology, Chinese Academy of Sciences (Wuhan, China), and was maintained in a sterilized medium under artificial climate conditions (25 °C, white fluorescent light illumination of 1000 lux, and light/dark cycle of 12 h:12 h).
Microalgae inoculum and cultivation
The inoculum of Spirulina platensis was cultivated in a 250 mL Erlenmeyer flask containing 100 mL sterilized medium under the same artificial climate conditions as described above. The cultures used modified Zarrouk's medium, which consisted of 13.61 g L−1 NaHCO3, 4.03 g L−1 Na2CO3, 0.5 g L−1 K2HPO4, 2.5 g L−1 NaNO3, 1.0 g L−1 K2SO4, 1.0 g L−1 NaCl, 0.2 g L−1 MgSO4·7H2O, 0.04 g L−1 CaCl2·2H2O, 0.01 g L−1 FeSO4·7H2O and 1.0 mL L−1 A5 solution (2.86 g L−1 H3BO3, 1.86 g L−1 MnCl2·4H2O, 0.22 g L−1 ZnSO4·7H2O, 0.39 g L−1 Na2MoO4·2H2O, 0.08 g L−1 CuSO4·5H2O, 0.05 g L−1 Co(NO3)2·6H2O). The initial inoculum ratio (Vinoculum/Vmedium) was 2.5%, approximately equaling to a biomass concentration of 0.127 ± 0.009 g L−1. During the experiment, the flasks were covered with perforated two-layered films to avoid contamination/evaporation and shaken by hand twice everyday to prevent aggregation. The growth inhibition of Spirulina platensis was investigated by adding enrofloxacin into the medium. Samples were taken from the algal culture at the predetermined time points and analyzed immediately.
Analytical methods
Microalgal growth was determined by the dry cell weight (DCW, mg L−1) through the relationship between DCW and OD560 (optical density at 560 nm) as follows: | DCW = 675.6 × OD560 + 32.0(R2 = 0.997) | (1) |
OD560 was measured by using a Metash UV-9000S UV-Vis spectrophotometer, while the DCW was calculated as the weight gain of a 0.45 μm filter paper by filtering 10 mL microalgal suspension and drying it at 105 °C for 2 h.20
The residual level of enrofloxacin in the medium was determined using a HPLC system (Alliance e2695, Waters, USA) after filtration through a 0.22 μm membrane. Isocratic separation of the 10 μL sample was carried out on a C18 column (250 × 4.6 mm, 5 μm) at a temperature of 35 °C and detection wavelength of 278 nm. The mobile phase was 0.1% formic acid aqueous solution/acetonitrile (80
:
20 v/v) at a flow rate of 1 mL min−1.
The degradation products were analyzed as described in a previous study,21 by using a liquid chromatograph-tandem mass spectrometer (1290-6430, Agilent, USA) after purification and concentration by solid phase extraction.22 In brief, PEP-2 cartridges (200 mg/6 mL, Agela, China) were activated using 3 mL methanol and 3 mL water. The filtered solution (10 mL) sampled from the culture medium was loaded onto the cartridge and washed with 3 mL water to reduce the interference. The cartridge was then dried under vacuum and consecutively washed with 3 mL methanol five times. The collected elute was evaporated under a nitrogen stream to 1 mL and 10 μL injected sample solution was separated on a C18 column (150 mm × 2.1 mm, 5 μm) with the mobile phase containing A (0.1% formic acid in water) and B (methanol). The gradient elution was as follows: 0 min, 95% A and 5% B; 0–20 min, a linear gradient to 0% A and 100% B; 20–23 min, 0% A and 100% B; 23–24 min, a linear gradient to 95% A and 5% B; 24–30 min, 95% A and 5% B. Positive electrospray ionization was operated with 4.0 kV capillary voltage. Mass spectra were recorded in full scan mode over the range of m/z 50–650. The fragmentation patterns were obtained by analyzing the selected target ions at a collision energy of 20 eV.
The photosynthetic pigments of chlorophyll a and carotenoids were determined based on the established method.23 Samples were centrifuged at 10
000 rpm for 10 min and washed with water three times. By discarding the supernatant, the obtained residues were mixed with methanol and incubated at 4 °C in the dark for 24 h. After centrifugation, the supernatant was analyzed spectrophotometrically at wavelengths of 665, 652 and 470 nm and computationally using the following formula:
| [Chlorophyll-a] = 16.72 × A665 − 9.16 × A652 | (2) |
| [Carotenoids] = (1000 × A470 − 1.63 × [chlorophyll-a])/221 | (3) |
The primary biocomponents including lipids, proteins and carbohydrates were determined according to previous studies. The amount of lipid was measured gravimetrically after extraction with a chloroform/methanol (1/1, v/v) solution.24 The content of protein was analyzed by the Kjeldahl method using a factor of 6.25 for conversion of nitrogen into protein.25 The carbohydrate content was measured based on the phenol–sulfuric acid colorimetric method by examining absorbance at 490 nm and using glucose as the standard.26
The experiments were performed in triplicate (n = 3), and the data were displayed as mean values with their standard deviations (SD). One-way analysis of variance (ANOVA) with an LSD post hoc test was employed to analyze the significant differences between the control group and different treatments on Spirulina platensis.
Results and discussion
The effect of enrofloxacin on the growth of Spirulina platensis
Fig. 1 shows the biomass growth of Spirulina platensis that were treated with different concentrations of enrofloxacin for 35 days. It is found in Fig. 1a that Spirulina platensis could grow well in a culture medium containing 0.0–1.0 mg L−1 of enrofloxacin. The stimulating effect was observed by adding a low level of enrofloxacin (e.g., 0.1 mg L−1) into the culture, showing a 14.6% biomass increment (P < 0.05) in comparison to the control experiment (0.0 mg L−1) on the 10th day. Additionally, the biomass of Spirulina platensis was lower than that observed in the control experiment when initially exposed to 1.0 mg L−1 enrofloxacin, but the growth recovered on the 35th day. A similar effect has been recently reported in the culture of Spirulina platensis via the introduction of relatively low levels of estrone and carbamazepine.18,20 This may be called hormesis, as likely attributed to the stress response of various kinds of microalgae.27 However, increasing the enrofloxacin concentration up to 10.0–100.0 mg L−1 would cause a significant and irreversible reduction of biomass as can be seen in Fig. 1b (P < 0.01). This implied complete inhibition and excessive toxicity toward Spirulina platensis. A recent investigation has reported that 1.0 mg L−1 enrofloxacin could already present significant inhibition on Chrysosporum ovalisporum (cyanobacterium), while 50.0 mg L−1 enrofloxacin could only slightly influence the growth of Chlorella vulgaris (green algae).28 This appears to be attributed to the fact that enrofloxacin is a broad-spectrum antibiotic with specific activity against bacteria by targeting DNA gyrase.29 Therefore, the sensitivity of Spirulina platensis (another cyanobacterium) to enrofloxacin is presumably similar to that of Chrysosporum ovalisporum, possibly due to the disturbance of its cell division.
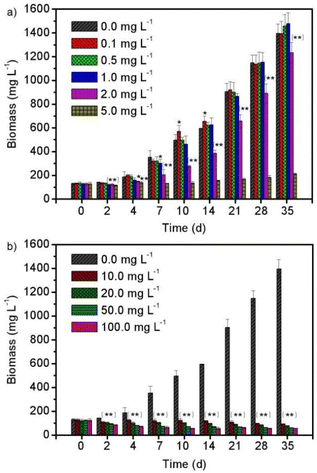 |
| Fig. 1 The biomass variations of Spirulina platensis exposed to (a) 0.0–5.0 mg L−1 and (b) 0.0–100.0 mg L−1 of enrofloxacin within a 35 day cultivation process. The statistically significant differences as compared to the control group were represented by * if P < 0.05 and ** if P < 0.01, respectively. | |
The effect of enrofloxacin on the chlorophyll a and carotenoid contents
Photosynthesis is a vital physiological process for algal growth, and is very sensitive to environmental stress including exposure to xenobiotic pollutants.30 Pigments such as chlorophyll a and carotenoids are widely believed to play crucial role in the photosynthetic system, and are also recognized to be potential biomarkers for stress monitoring.8,31 Therefore, the contents of photosynthetic pigments (chlorophyll a and carotenoids) in Spirulina platensis were studied under various concentrations of enrofloxacin. As can be seen in Fig. 2a, the content of chlorophyll a increased significantly via treating Spirulina platensis with 1.0–5.0 mg L−1 enrofloxacin (P < 0.05). The massive accumulation of chlorophyll a should be a protective mechanism activated by the toxicant (i.e., enrofloxacin) as shown in previous studies.14,30 Carotenoids are a group of photosynthetic pigments, which contribute to light harvesting over a different range of wavelengths from chlorophyll.30 The variation of carotenoid content in Fig. 2b coincides well with that of chlorophyll a, showing a gradual increment under 1.0–5.0 mg L−1 of enrofloxacin treatment. This might be attributed to the stress response of microalgae by promoting the synthesis of carotenoids to scavenge the excess reactive oxygen species and thus to prevent cellular damage.14,16 In other words, carotenoids can act as antioxidants to protect the photosynthetic system and maintain an intact cell membrane, which should be important in growth and detoxification.14,32 Comparatively, the effect of enrofloxacin on photosynthetic pigments of cyanobacterium was more significant than that of other antibiotics such as carbamazepine, sulfadiazine, and sulfamethazine.20,28 The result implied that the toxicity of enrofloxacin to Spirulina platensis might include not only lipid peroxidation but also the adaptation of the photosynthetic system for converting energy to biomass and biocomponents.16,31
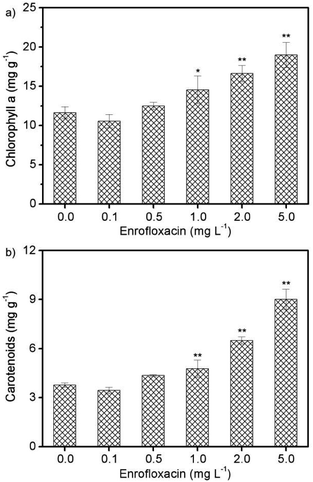 |
| Fig. 2 The contents of (a) chlorophyll a and (b) carotenoids in Spirulina platensis after 10 days exposure to 0.0–5.0 mg L−1 enrofloxacin. The statistically significant differences as compared to the control group were represented by * if P < 0.05 and ** if P < 0.01, respectively. | |
The effect of enrofloxacin on protein, lipid, and carbohydrate contents
In response to environmental stress, microalgae could adjust their physiological activities for adaptation, resulting in significant changes of primary biocomponents including proteins, lipids, and carbohydrates.8Spirulina platensis is typically rich in protein accounting for more than 60% of its biomass content, but is commonly poor in lipids (6–13%).33 On the basis of these characteristics, the protein content in Spirulina platensis was supposed to be sensitive to xenobiotic pollutants, and was thus investigated after exposure to different concentrations of enrofloxacin as displayed in Fig. 3a. It is found that 0.1–1.0 mg L−1 enrofloxacin could significantly improve the protein content from 56.6% to 61.1–67.3% (P < 0.05), which may be attributed to the increased enzymes and pigments for defending against environmental stress.15 However, 5.0 mg L−1 enrofloxacin would cause the obvious reduction of protein content to 49.1% (P < 0.01), implying the possible defolding and defunctions of protein.8,16 In contrast to proteins, the lipid content in Spirulina platensis reached the highest value of 12.1% by treating with 0.1 mg L−1 enrofloxacin, and then exhibited a significant downward trend to 6.2% by treating with 0.5–5.0 mg L−1 enrofloxacin in Fig. 3b (P < 0.01). This is in agreement with the results of algal growth, reflecting the potential role of lipid in adaptation, possibly through absorbing the organic pollutants to limit their bioavailability.34 Interestingly, the carbohydrate content in Spirulina platensis decreased to 22.7% by treating with 0.1 mg L−1 of enrofloxacin (P < 0.05), and then increased significantly to 30.2% by treating with 2.0 mg L−1 enrofloxacin in Fig. 3c (P < 0.01). It is clear to infer from the results that 5.0 mg L−1 enrofloxacin had an obviously toxic effect on Spirulina platensis, leading to a comprehensive decline of its biocomponents (proteins, lipids, and carbohydrates). Under the stimulation of low levels of enrofloxacin, both proteins and lipids were regulated preferentially to cope with the negative effects of enrofloxacin, thus correspondingly reducing the accumulation of carbohydrate in the algal cells. With the increment of the enrofloxacin concentration, Spirulina platensis is becoming inclined to produce more carbohydrates, which could act as a barrier to prevent the entry of pollutants into algal cells.8 This may be the reason for the negligible reduction of the enrofloxacin concentration after 10 days of cultivation by using 0.5–2.0 mg L−1 enrofloxacin in Fig. 4. In other words, Spirulina platensis seems to be capable of regulating primary biocomponents to trigger defense mechanisms such as exclusion and metabolism.
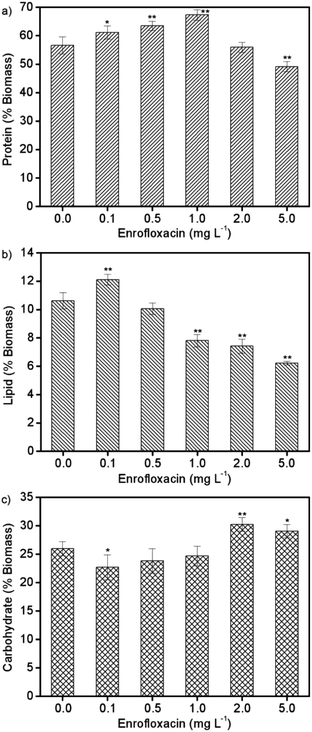 |
| Fig. 3 The contents of (a) protein, (b) lipid, and (c) carbohydrate in Spirulina platensis after 10 days of exposure to 0.0–5.0 mg L−1 enrofloxacin. The statistically significant differences as compared to the control group were represented by * if P < 0.05 and ** if P < 0.01, respectively. | |
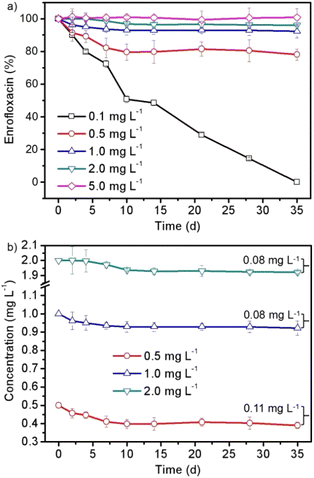 |
| Fig. 4 The (a) changed amount and (b) decreased concentration of enrofloxacin in the 35 day cultivation process of Spirulina platensis treated with 0.1–5.0 mg L−1 enrofloxacin. | |
The removal of enrofloxacin from the Spirulina platensis culture
In order to further discuss the interaction between Spirulina platensis and enrofloxacin, the changes in the enrofloxacin contents as a function of time were investigated as shown in Fig. 4. A preliminary study has been carried out to confirm the negligible abiotic degradation of enrofloxacin under the same culture conditions without inoculation of Spirulina platensis as can be seen in Fig. S1,† which coincides well with the result of a previous study.14Fig. 4a shows that 0.1 mg L−1 enrofloxacin could be completely removed in the 35 day cultivation process. The amount of enrofloxacin was then decreased by 22.0%, 7.8%, and 3.9% when increasing the enrofloxacin concentration to 0.5, 1.0, and 2.0 mg L−1, respectively, whereas no significant change was observed when applying 5.0 mg L−1 of enrofloxacin. This result is in agreement with previous studies that the removal efficiency by microalgae generally decreased with increasing the concentration of xenobiotic pollutants, most likely because of the increased toxic effect.18,20 Particularly in this work, the changes of enrofloxacin amount in Fig. 4a corresponded well with the growth inhibition of Spirulina platensis in Fig. 1. In other words, the removal of enrofloxacin could be only observed in the culture of growing Spirulina platensis (i.e. treated with 0.1–2.0 mg L−1 enrofloxacin). This may be due to the fact that Spirulina platensis is one kind of mixotrophic species and has the ability to assimilate organic compounds.17 Similar results have also been found in culturing Chlorella and Microcystis accompanied with the stimulating effects on their biomass growth, which are identical to our findings in Fig. 1.13 Consequently, the removal of enrofloxacin by Spirulina platensis was proposed to be a metabolic reaction, which is identical to the degradation of carbamazepine and estrone by microalgal species.18,20 Nevertheless, the decreased concentration of enrofloxacin was found to be restricted at 0.08–0.11 mg L−1 as displayed in Fig. 4b. The result seemed to indicate the limited ability of Spirulina platensis to remove enrofloxacin. It once again suggested the toxic effect of xenobiotic pollutants on the physiological state of Spirulina platensis. The adaptation stage of Spirulina platensis could be clearly observed in the presence of 2.0 mg L−1 enrofloxacin, showing an almost negligible reduction of the enrofloxacin concentration in the first 4 days. Moreover, the stability of 0.5–2.0 mg L−1 enrofloxacin after 10 days of cultivation is different from that observed for 0.1 mg L−1 enrofloxacin (Fig. 4), implying the already occurred physiological changes of Spirulina platensis, albeit its growth was still vigorous under these conditions (Fig. 1).
The products of enrofloxacin in the Spirulina platensis culture
To further study the metabolic process, the degradation products of enrofloxacin were examined after 10 days cultivation of Spirulina platensis, resulting in the identification of P332, P346, P291, and P334 in Fig. S2.† As shown in Fig. S3,† the molecular ion of enrofloxacin at m/z 360 corresponded well with the molecular weight of its protonated form. The fragment ions at m/z 342 and 316 appeared due to the loss of fluoro or carboxyl groups from the molecular ion, while the fragment ion at m/z 245 resulted from the breakage of piperazine ring of the fragment ion at m/z 316 via losing the ethyl group and C2H4N moiety. Based on these structural characteristics, P332 was proposed to be ciprofloxacin (Fig. 5a), which was commonly found as the dealkylated product of enrofloxacin.35 The molecular ion at m/z 332 and the fragment ions at m/z 314 and 288 could be recognized as transformation of the ions at m/z 360, 342 and 316 of enrofloxacin by reducing the mass weight of 28 daltons (ethyl group). The fragment ion at m/z 245 was assigned to be the product of the fragment ion at m/z 288 by losing the C2H5N moiety, confirming the absence of the ethyl group in P332 (ciprofloxacin). In Fig. 5b, P346 was proposed to be the carbonyl substituted ciprofloxacin with the molecular ion at m/z 346, corresponding to the oxidized product of ciprofloxacin with the mass gain of 14 daltons.36 This was supported by the detection of the fragment ion at m/z 284, which was dissociated from the molecular ion at m/z 346 by losing both fluoro and carboxyl groups. According to the “nitrogen rule”, P291 should have two nitrogen atoms in its structure (Fig. 5c), and was thus proposed to be the oxidized product of P346.35,37 The loss of the fluoro group from the molecular ion could produce the fragment ion at m/z 273, whose further fragmentation generated the fragment ion at m/z 243 by losing the hydroxyl group and oxygen atom. Fig. 5d displays the fragmentation pattern (334
:
316
:
290) of P334 involving the loss of fluoro or carboxyl groups, which were identical to that of enrofloxacin. P334 was then proposed to be the dealkylated product of enrofloxacin and could be also oxidized to yield P291.38 The fragment ion at m/z 245 was considered as the product of the fragment ion at m/z 290 by further breaking the C–N bond. It is then deduced that the metabolism of enrofloxacin by Spirulina platensis should primarily occur at the piperazine substituent through dealkylation and oxidation. Although the identified products preserved the intact quinolone structure, the destruction of the piperazine ring has been considered to be sufficient to eliminate the antibacterial activity of enrofloxacin.38 On the basis of the identified products, the possible degradation pathways of enrofloxacin could be proposed schematically in Fig. S4,† showing the initial dealkylation from the piperazine group to produce P332 and P334, followed by the subsequent oxidation to give P346 and finally P291. This biodegradation might be related to the intracellular cytochrome P450 enzyme family (e.g., demethylase and hydroxylase) and the extracellular biofilm digestion system (e.g., excreted extracellular polymeric substances and enzymes).13,39
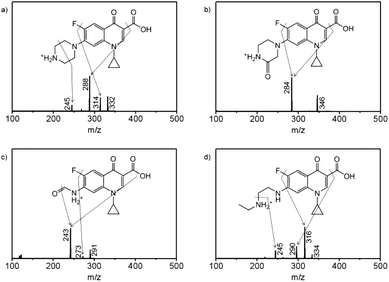 |
| Fig. 5 The tandem mass spectra by fragmenting molecular ions at (a) m/z 332, (b) m/z 346, (c) m/z 291, and (d) m/z 334 and the corresponding structural interpretations for the proposed products including (a) P332, (b) P346, (c) P291, and (d) P334. | |
The detoxification mechanism and environmental significance
In comparison with previous studies, it seems reasonable to suggest that Spirulina platensis should be very sensitive to enrofloxacin. For instance, Spirulina platensis could maintain its growth even when exposed to 50 mg L−1 of carbamazepine or estrone, but would be completely inhibited by treating with 5 mg L−1 of enrofloxacin.18,20 Furthermore, the high toxicity of enrofloxacin to Spirulina platensis cannot be observed for other microalgal species such as Scenedesmus, Chlamydomonas, Chlorella, Ourococcus, and Micractinium.14 Consequently, enrofloxacin should have specific activity against Spirulina platensis, which is a type of prokaryote that is similar to Gram-negative bacteria.40 Note that the role of enrofloxacin is the fixation of the enzyme–DNA complex by primarily targeting the A subunit of DNA gyrase.41 It is thus assumed that the adaptation mechanism of enrofloxacin by Spirulina platensis might be related to the reduced binding capacity between degradation products and the enzyme. The docking simulation was then implemented as described in Text S1.† As shown in Fig. 6a, enrofloxacin could be successfully docked into the DNA gyrase A active site, displaying the same binding mode as moxifloxacin in the original co-crystallized structure.42 The crucial interactions were found to be primarily the hydrogen bonds between the keto-carboxyl groups of enrofloxacin and glutamic acid–serine residues of gyrase via the water-ion bridge in Fig. 6b. This is exactly the key action of fluoroquinolone antibiotics on DNA gyrase, so as to prevent the cleavage and reunion of DNA strands.41 Fig. S5† shows the docking results of DNA gyrase with the above-mentioned degradation products, whose interactions are identical to those of enrofloxacin in Fig. 6b. It is thus reasonable to evaluate the docking score (i.e., binding affinity) of each determined complex in Fig. 6c, exhibiting the lower energy values of degradation products (except for the intermediate P346) than enrofloxacin. The result means reduced antimicrobial potency accompanying the metabolic process, illustrating the detoxification mechanism of enrofloxacin by Spirulina platensis, and is also important in dealing with the environmental risk from enrofloxacin contamination. As one of the fluoroquinolone antibiotics, which is the only DNA gyrase inhibitor approved clinically, enrofloxacin has and will continue to be used extensively.43 Hence, the cultivation of Spirulina platensis may have to face its ubiquitous existence due to the inevitable leakage of enrofloxacin into the water resource.2 Fortunately, the growth and metabolism of Spirulina platensis were found to be not only insensitive to a low level (0.1 mg L−1) of enrofloxacin (commonly ng to μg L−1 in aqueous environments) but also capable of eliminating this pollutant and its antimicrobial activity.6 Consequently, culturing Spirulina platensis is suggested to be a very promising solution to make use of solar energy for simultaneous hazard reduction (e.g. enrofloxacin) and resource harvest (e.g. biomass). Based on the current findings, these advantages are also expected to be further strengthened by emerging technologies such as genetic engineering.39
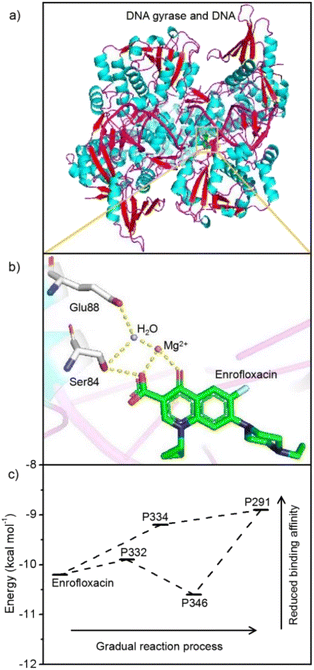 |
| Fig. 6 Schematic diagrams of (a) the complex structure of enrofloxacin with DNA gyrase and (b) the critical interactions (the hydrogen bonds displayed as dashed lines) of enrofloxacin with DNA gyrase via the H2O–Mg2+ bridge (carbon atoms are colored green in enrofloxacin while colored white in residues Ser84 and Glu88, nitrogen atoms are colored blue, and oxygen atoms are colored red) and the (c) corresponding docking scores of enrofloxacin and its degradation products. | |
Conclusions
In summary, we investigated the ecotoxicological effect of enrofloxacin on Spirulina platensis and the corresponding detoxification mechanism. It is found that the biomass accumulation of Spirulina platensis was inhibited by 5.0 mg L−1 enrofloxacin but was stimulated by 0.1 mg L−1 enrofloxacin. Under this environmental stress, photosynthetic pigments and primary biocomponents showed obvious variations, which should be adaptation for defending against the pollutant. The removal of 0.1 mg L−1 enrofloxacin was observed in the cultivation process, implying the remediation ability of growing microalgae. The metabolic process was further studied by investigating the enrofloxacin degradation, showing primarily the dealkylation and oxidation of the piperazine group. The detoxification mechanism was attributed to the reduced antimicrobial activity probably being accompanied by the gradual metabolism of enrofloxacin by Spirulina platensis. All these results are considered as great support for further use of Spirulina platensis for evaluating ecological risks and reducing environmental hazards.
Author contributions
Xiaohua Jiang: conceptualisation, methodology, investigation, analysis, drafting, and revising; Dabin Wang: conceptualisation, analysis, and revising; Weiran Wu: methodology, and analysis; Fengmin Li: conceptualisation and revising.
Conflicts of interest
The authors declare that they have no known competing financial interests or personal relationships that could have appeared to influence the work reported in this paper.
Acknowledgements
This work was supported by the National Key R&D Program of China (2018YFC0406304), the Central Public-Interest Scientific Institution Basal Research Fund (1610232019005), and the Agricultural Science and Technology Innovation Program (ASTIPTRIC06).
References
- Y. Y. Zhang, L. F. Wang, H. Zhuang, X. X. Li, X. J. Gao, Z. H. An, X. D. Liu, H. Yang, W. Z. Wei and X. J. Zhang, Excessive use of enrofloxacin leads to growth inhibition of juvenile giant freshwater prawn Macrobrachium rosenbergii, Ecotoxicol. Environ. Saf., 2019, 169, 344–352, DOI:10.1016/j.ecoenv.2018.11.042.
- J. Du, H. X. Zhao, Y. Wang, H. J. Xie, M. H. Zhu and J. W. Chen, Presence and environmental risk assessment of selected antibiotics in coastal water adjacent to mariculture areas in the Bohai Sea, Ecotoxicol. Environ. Saf., 2019, 177, 117–123, DOI:10.1016/j.ecoenv.2019.03.075.
- R. C. Wei, F. Ge, M. Chen and R. Wang, Occurrence of ciprofloxacin, enrofloxacin, and florfenicol in animal wastewater and water resources, J. Environ. Qual., 2012, 41, 1481–1486, DOI:10.2134/jeq2012.0014.
- D. G. J. Larsson, C. de Pedro and N. Paxeus, Effluent from drug manufactures contains extremely high levels of pharmaceuticals, J. Hazard. Mater., 2007, 148, 751–755, DOI:10.1016/j.jhazmat.2007.07.008.
- V. K. Sharma, N. Johnson, L. Cizmas, T. J. McDonald and H. Kim, A review of the influence of treatment strategies on antibiotic resistant bacteria and antibiotic resistance genes, Chemosphere, 2016, 150, 702–714, DOI:10.1016/j.chemosphere.2015.12.084.
- Y. X. Zhang, H. Y. Chen, L. J. Jing and Y. G. Teng, Ecotoxicological risk assessment and source apportionment of antibiotics in the waters and sediments of a peri-urban river, Sci. Total Environ., 2020, 731, 139128, DOI:10.1016/j.scitotenv.2020.139128.
- H. Xu, J. de Koning and Y. Geng, Reliability and efficiency of an advanced tertiary treatment process for wastewater reclamation, J. Water Reuse Desalin., 2019, 9, 385–395, DOI:10.2166/wrd.2019.007.
- V. Shashirekha, M. R. Sridharan and M. Swamy, Biochemical response of cyanobacterial species to trivalent chromium stress, Algal Res., 2015, 12, 421–430, DOI:10.1016/j.algal.2015.10.003.
- M. B. Kurade, J. R. Kim, S. P. Govindwar and B. H. Jeon, Insights into microalgae mediated biodegradation of diazinon by Chlorella vulgaris: microalgal tolerance to xenobiotic pollutants and metabolism, Algal Res., 2016, 20, 126–134, DOI:10.1016/j.algal.2016.10.003.
- D. A. Song, H. Y. Cheng, X. H. Jiang, H. Q. Sun, F. Y. Kong, R. N. Liang, Z. M. Qiang, H. J. Liu and J. H. Qu, Oxidative removal of quinclorac by permanganate through a rate-limiting [3 + 2] cycloaddition reaction, Environ. Sci.: Processes Impacts, 2018, 20, 790–797, 10.1039/C8EM00024G.
- G. Forlani, V. Prearo, D. Wieczorek, P. Kafarski and J. Lipok, Phosphonate degradation by Spirulina strains: cyanobacterial biofilters for the removal of anticorrosive polyphosphonates from wastewater, Enzyme Microb. Technol., 2011, 48, 299–305, DOI:10.1016/j.enzmictec.2010.12.005.
- W. J. Hui, E. M. David and J. Y. Huang, Using C. vulgaris assisted microbial desalination cell as a green technology in landfill leachate pre-treatment: a factor-performance relation study, J. Water Reuse Desalin., 2020, 10, 1–16, DOI:10.2166/wrd.2019.073.
- K. Miazek and B. Brozek-Pluska, Effect of PHRs and PCPs on microalgal growth, metabolism and microalgae-based bioremediation processes: a review, Int. J. Mol. Sci., 2019, 20, 2492, DOI:10.3390/ijms20102492.
- J. Q. Xiong, M. B. Kurade and B. H. Jeon, Ecotoxicological effects of enrofloxacin and its removal by monoculture of microalgal species and their consortium, Environ. Pollut., 2017, 226, 486–493, DOI:10.1016/j.envpol.2017.04.044.
- E. F. Shabana, M. A. Gabr, H. R. Moussa, E. A. El-Shaer and M. M. S. Ismaiel, Biochemical composition and antioxidant activities of Arthrospira (Spirulina) platensis in response to gamma irradiation, Food Chem., 2017, 214, 550–555, DOI:10.1016/j.foodchem.2016.07.109.
- M. M. S. Ismaiel, M. D. Piercey-Normore and C. Rampitsch, Proteomic analyses of the cyanobacterium Arthrospira (Spirulina) platensis under iron and salinity stress, Environ. Exp. Bot., 2018, 147, 63–74, DOI:10.1016/j.envexpbot.2017.11.013.
- M. Kurashvili, T. Varazi, G. Khatisashvili, G. Gigolashvili, G. Adamia, M. Pruidze, G. Gordeziani, L. Chokheli, S. Japharashvili and N. Khuskivadze, Blue-green alga Spirulina as a tool against water pollution by 1,1'-(2,2,2-trichloroethane-1,1-diyl)bis(4-chlorobenzene) (DDT), Ann. Agric. Sci., 2018, 16, 405–409, DOI:10.1016/j.aasci.2018.07.005.
- N. Sami and T. Fatma, Studies on estrone biodegradation potential of cyanobacterial species, Biocatal. Agric. Biotechnol., 2019, 17, 576–582, DOI:10.1016/j.bcab.2019.01.022.
- E. van der Grinten, M. G. Pikkemaat, E. van den Brandhof, G. J. Stroomberg and M. H. S. Kraak, Comparing the sensitivity of algal, cyanobacterial and bacterial bioassays to different groups of antibiotics, Chemosphere, 2010, 80, 1–6, DOI:10.1016/j.chemosphere.2010.04.011.
- Q. F. Wang, W. B. Liu, X. T. Li, R. Wang and J. Zhai, Carbamazepine toxicity and its co-metabolic removal by the cyanobacteria Spirulina platensis, Sci. Total Environ., 2020, 706, 135686, DOI:10.1016/j.scitotenv.2019.135686.
- P. H. Shao, Z. J. Ren, J. Y. Tian, S. S. Gao, X. B. Luo, W. X. Shi, B. Y. Yan, J. Li and F. Y. Cui, Silica hydrogel-mediated dissolution-recrystallization strategy for synthesis of ultrathin α-Fe2O3 nanosheets with highly exposed (110) facets: a superior photocatalyst for degradation of bisphenol S, Chem. Eng. J., 2017, 323, 64–73, DOI:10.1016/j.cej.2017.04.069.
- X. H. Jiang, D. A. Song, D. B. Wang, R. M. Zhang, Q. N. Fang, H. Q. Sun and F. Y. Kong, Eliminating imidacloprid and its toxicity by permanganate via highly selective partial oxidation, Ecotoxicol. Environ. Saf., 2020, 191, 110234, DOI:10.1016/j.ecoenv.2020.110234.
- H. K. Lichtenthaler, Chlorophylls and carotenoids: pigments of photosynthetic biomembranes, Methods Enzymol., 1987, 148, 350–382, DOI:10.1016/0076-6879(87)48036-1.
- E. G. Bligh and W. J. Dyer, A rapid method of total lipid extraction and purification, Can. J. Biochem. Physiol., 1959, 37, 911–917, DOI:10.1139/o59-099.
- T. Y. Zhang, X. X. Wang, Y. H. Wu, J. H. Wang, V. M. Deantes-Espinosa, L. L. Zhuang, H. Y. Hu and G. X. Wu, Using straw hydrolysate to cultivate chlorella pyrenoidosa for high-value biomass production and the nitrogen regulation for biomass composition, Bioresour. Technol., 2017, 244, 1254–1260, DOI:10.1016/j.biortech.2017.05.095.
- M. Dubois, K. A. Gilles, J. K. Hamilton, P. A. Rebers and F. Smith, Colorimetric method for determination of sugars and related substances, Anal. Chem., 1956, 28, 350–356, DOI:10.1021/ac60111a017.
- W. W. Yang, Z. P. Tang, F. Q. Zhou, W. H. Zhang and L. R. Song, Toxicity studies of tetracycline on Microcystis aeruginosa and Selenastrum capricornutum, Environ. Toxicol. Pharmacol., 2013, 35, 320–324, DOI:10.1016/j.etap.2013.01.006.
- S. Chen, W. Zhang, J. Y. Li, M. Z. Yuan, J. H. Zhang, F. Xu, H. T. Xu, X. Y. Zheng and L. Q. Wang, Ecotoxicological effects of sulfonamides and fluoroquinolones and their removal by a green alga (Chlorella vulgaris) and a cyanobacterium (Chrysosporum ovalisporum), Environ. Pollut., 2020, 263, 114554, DOI:10.1016/j.envpol.2020.114554.
- S. Carusso, A. B. Juárez, J. Moretton and A. Magdaleno, Effects of three veterinary antibiotics and their binary mixtures on two green alga species, Chemosphere, 2018, 194, 821–827, DOI:10.1016/j.chemosphere.2017.12.047.
- P. Tsiaka, V. Tsarpali, I. Ntaikou, M. N. Kostopoulou, G. Lyberatos and S. Dailianis, Carbamazepine-mediated pro-oxidant effects on the unicellular marine algal species Dunaliella tertiolecta and the hemocytes of mussel Mytilus galloprovincialis, Ecotoxicology, 2013, 22, 1208–1220, DOI:10.1007/s10646-013-1108-3.
- X. Y. Liu, Y. Hong and W. P. Gu, Influence of light quality on Chlorella growth, photosynthetic pigments and high-valued products accumulation in coastal saline-alkali leachate, J. Water Reuse Desalin., 2021, 11, 301–311, DOI:10.2166/wrd.2021.088.
- C. Paliwal, I. Pancha, T. Ghosh, R. K. Maurya, K. Chokshi, S. V. V. Bharadwaj, S. Ram and S. Mishra, Bioresour. Technol., 2015, 197, 363–368, DOI:10.1016/j.biortech.2015.08.122.
- Z. Cohen and A. Vonshak, Fatty acid composition of Spirulina and spirulina-like cyanobacteria in relation to their chemotaxonomy, Phytochemistry, 1991, 30, 205–206, DOI:10.1016/0031-9422(91)84125-C.
- V. Asselborn, C. Fernandez, Y. Zalocar and E. R. Parodi, Effects of chlorpyrifos on the growth and ultrastructure of green algae, Ankistrodesmus gracilis, Ecotoxicol. Environ. Saf., 2015, 120, 334–341, DOI:10.1016/j.ecoenv.2015.06.015.
- A. Junza, J. Saurina, D. Barrón and C. Minguillón, Metabolic profile modifications in milk after enrofloxacin administration studied by liquid chromatography coupled with high resolution mass spectrometry, J. Chromatogr. A, 2016, 1460, 92–99, DOI:10.1016/j.chroma.2016.07.016.
- J. F. Carneiro, J. M. Aquino, B. F. Silva, A. J. Silva and R. C. Rocha-Filho, Comparing the electrochemical degradation of the fluoroquinolone antibiotics norfloxacin and ciprofloxacin using distinct electrolytes and a bdd anode: evolution of main oxidation byproducts and toxicity, J. Environ. Chem. Eng., 2020, 8, 104433, DOI:10.1016/j.jece.2020.104433.
- D. A. Song, W. A. Jefferson, H. Y. Cheng, X. H. Jiang, H. Q. Z. M. Qiang, H. He, H. J. Liu and J. H. Qu, Acidic permanganate oxidation of sulfamethoxazole by stepwise electron-proton transfer, Chemosphere, 2019, 222, 71–82, DOI:10.1016/j.chemosphere.2019.01.113.
- Y. P. Xu, S. Y. Liu, F. Guo and B. Zhang, Evaluation of the oxidation of enrofloxacin by permanganate and the antimicrobial activity of the products, Chemosphere, 2016, 144, 113–121, DOI:10.1016/j.chemosphere.2015.07.083.
- J. Q. Xiong, M. B. Kurade and B. H. Jeon, Can microalgae remove pharmaceutical contaminants from water, Trends Biotechnol., 2018, 36, 30–44, DOI:10.1016/j.tibtech.2017.09.003.
- P. Välitalo, A. Kruglova, A. Mikola and R. Vahala, Toxicological impacts of antibiotics on aquatic micro-organisms: a mini-review, Int. J. Hyg. Environ. Health, 2017, 220, 558–569, DOI:10.1016/j.ijheh.2017.02.003.
- T. R. Blower, B. H. Williamson, R. J. Kerns and J. M. Berger, Crystal structure and stability of gyrase-fluoroquinolone cleaved complexes from Mycobacterium tuberculosis, Proc. Natl. Acad. Sci. U. S. A., 2016, 113, 1706–1713, DOI:10.1073/pnas.1525047113.
- P. F. Chan, V. Srikannathasan, J. Huang, H. Cui, A. P. Fosberry, M. Gu, M. M. Hann, M. Hibbs, P. Homes, K. Ingraham, J. Pizzollo, C. Shen, A. J. Shillings, C. E. Spitzfaden, R. Tanner, A. J. Theobald, R. A. Stavenger, B. D. Bax and M. N. Gwynn, Structural basis of DNA gyrase inhibition by antibacterial QPT-1, anticancer drug etoposide and moxifloxacin, Nat. Commun., 2015, 6, 10048, DOI:10.1038/ncomms10048.
- M. Brvar, A. Perdih, M. Renko, G. Anderluh, D. Turk and T. Solmajer, Structure-based discovery of substituted 4,5'-bithiazoles as novel DNA gyrase inhibitors, J. Med. Chem., 2012, 55, 6413–6426, DOI:10.1021/jm300395d.
|
This journal is © The Royal Society of Chemistry 2023 |