Likely substantial underestimation of reported methane emissions from United Kingdom upstream oil and gas activities†
Received
22nd September 2022
, Accepted 27th October 2022
First published on 22nd December 2022
Abstract
The United Kingdom (UK) government's National Atmospheric Emissions Inventory (NAEI) is used to provide UK greenhouse gas emission data to the United Nations Framework Convention on Climate Change. The NAEI bottom-up approach estimated 2019 methane (CH4) emissions from the extraction and transport of oil and natural gas (O&G) from offshore sources to onshore terminals at 52 Gg CH4, corresponding to the loss of 0.14% of gas production. Here we investigate the approaches used by the NAEI and find it substantially underestimates leakage. We suggest alternative integrated approaches that combine direct measurements, management practices, and the effects of environmental conditions to estimate fugitive emissions. We estimate the total UK CH4 emissions from flaring, combustion, processing, venting, and O&G transfer to be 289 Gg CH4 (0.72% of production), five times larger than the NAEI estimate. We suggest NAEI underestimates emissions because of outdated/incorrect emission factors, incomplete activity data, and incomplete data on vented emissions. Similarly, CH4 emissions from upstream O&G in other countries may be underestimated, with regional regulatory differences resulting in venting often being a substantially underestimated emission source. While bottom-up methods can be used to understand the relative size of emissions from the extraction and transport of O&G, they are inherently biased low as they only include emissions from processes and activities designated as emission sources and for which emission factors exist. Emission factors remain a large source of uncertainty as many used to generate the NAEI inventory are taken from industrial studies or unpublished research that have not been independently validated. To improve the NAEI estimate, widespread and frequent direct measurements are needed to supplement and improve bottom-up emission estimates generated with existing emission factors and activity levels.
Broader context
Accurate quantification of methane emissions is important as it is a strong greenhouse gas and contributes to production of tropospheric ozone, which is damaging to human health, ecosystems and agriculture. In general, greenhouse gas inventories are generated using activity data and emission factors. The UK government currently estimates 0.13% of the methane extracted from offshore activities is lost before it reaches land. These emission estimates are reported to the Intergovernmental Panel on Climate Change (IPCC), the United Nations Economic Commission for Europe (UNECE) and the United Nations Framework Convention on Climate Change and used to better understand the drivers of climate change. Recent studies suggest that emission factors currently used for inventory development may not accurately represent emissions over the full range of actual environmental conditions and management practices and that some emitting activities are not reported. Hence, emissions may be much higher than currently reported. Here we reassess methane lost from extraction and transport of natural gas in the UK and globally. We critically evaluate current methods, addressing their shortcomings, and provide methodologies and recalculations which indicate five times more methane is leaked from upstream oil and gas processes in the UK than is indicated in the NAEI.
|
1. Introduction
In 2019, natural gas (NG) supplied 41% of the United Kingdom's (UK's) total energy demands,1 with 50% coming from UK offshore oil and gas (O&G) operations and 50% imported either using pipelines or liquified NG, including imports from Norway (30%), Qatar (9%), USA (3%), Russia (3%) and the Netherlands (2%).2 Many recent studies have shown that methane (CH4) is lost during extraction and transport of O&G.3–6 Leakage of CH4 is important as it is a strong greenhouse (GHG) gas (GWP20 = 84; GWP100 = 28), contributes to production of tropospheric ozone which is damaging to human health, ecosystems and agriculture,7,8 and is identified as a key target gas for reduction to meet climate goals.9,10 The UK is a participant in the Global Methane Pledge in which participating countries commit to reducing global CH4 emissions by at least 30% from 2020 levels by 2030. Under this pledge the UK has committed to “…working to continuously improve the accuracy, transparency, consistency, comparability and completeness of [their] national greenhouse gas inventory reporting under the UNFCC and Paris Agreement”.11 Here we investigate the UK National Atmospheric Emissions Inventory (NAEI) methodology and CH4 leakage from upstream UK O&G production and transmission processes which primarily occur during offshore extraction and transport.
Currently, the UK government estimates 52 Gg year−1 (0.13%) of the 22 Tg year−1 CH4 produced offshore by the UK is lost before it reaches land.2 Emissions are estimated by each O&G operator for flaring, venting and offshore oil loading activities using activity data, typically the net energy production from each facility and emission factors (EFs). The emission estimates are then reported to the UK NAEI.12 As part of the Paris Agreement,13 the UK publishes the NAEI to report anthropogenic GHG emissions to the Intergovernmental Panel on Climate Change (IPCC), the United Nations Economic Commission for Europe (UNECE) and the United Nations Framework Convention on Climate Change.14
In 2019, 99.8% of UK O&G was produced offshore using 323 installations run by 29 different operators (Fig. 1). Of these, 257 were production platforms, where O&G was transported to the mainland by pipeline, and 66 were floating production storage and offloading (FPSO) installations.15 FPSOs extract O&G, process and store oil until it can be transported by tanker, and transfer gas to the UK by pipeline. The UK only extracts 0.2% of produced O&G onshore, primarily from a single site at Wytch Farm in Dorset.2
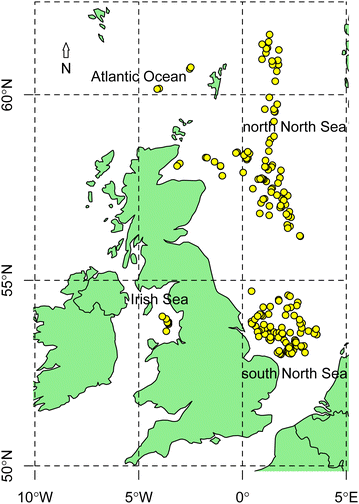 |
| Fig. 1 Locations of all UK offshore oil/gas operations (yellow circles). Source: North Sea Transition Authority, 2021. | |
Typically, CH4 emissions from upstream O&G operations reported to the IPCC or the UNECE are derived from bottom-up methods which use EFs for specific processes multiplied by those processes’ activity levels.12,15,16 However, recent studies suggest that EFs currently used for inventory development may not accurately represent emissions over the full range of actual environmental conditions and management practices.10,17–20 These studies suggest that emissions are dynamic and can be affected by wind speed, temperature, atmospheric pressure, gas venting management and oil offloading strategies and frequency. In addition, some processes that emit CH4 may be entirely missed. Thus, emissions can deviate substantially from those calculated from static EFs in bottom-up inventories like the NAEI. Overall, GHG emission estimates have the highest integrity when verified by direct, top-down atmospheric flux measurements.5,20–23
Currently, the best strategy to directly measure emissions from offshore facilities is unclear. The US EPA's Other Test Method (OTM) 33A24 is potentially confounded by the marine boundary layer and it is unclear if these traditional methods can be used to generate representative emission estimates.25,26 Tracer release methods are also commonly used to quantify site-wide emissions onshore,27 however, the transportation and installation of the required compressed gas cylinders is a significant safety concern and is hence unlikely to be adopted by operators. Mass balance methods using drones28 would avoid the micrometeorological issues presented to OTM33A and the safety concerns of tracer release, however drone mounted trace gas analysers (fixed wing or otherwise) cannot operate in strong winds and will be limited to fair weather measurements that would not inform the efficacy of the flare under adverse conditions. Satellites, such as the GHGSat instrument suite, are capable of observing site-wide emissions, however, satellites have difficulty with CH4 retrievals over water.29 Developments in remote sensing of CH4 over water may improve in the future and research is already suggesting ways to overcome the over-water retrieval issues.30,31
As technology and methodology for direct measurement of emissions from offshore facilities in the UK remains unproven, bottom-up methodology remains the best way of estimating emissions. However, the suitability of EFs used to derive the emissions remains unclear. In this study we reassess the CH4 loss from extraction and transport of O&G within the UK. This study only estimates losses from offshore extraction in the UK and from UK high-pressure transmission networks and does not estimate emissions from onshore, extraction in other countries or from low-pressure distribution networks. Our aim is to critically evaluate current bottom-up methods of estimating upstream UK CH4 emissions, address their shortcomings, and provide methodologies and recalculations of the estimates as necessary.
2. Upstream CH4 leakage: processes, sources, NAEI estimates, and improved methods of estimation
2.1. Offshore oil and gas extraction processes
Offshore production platforms extract an oil/gas/water mixture from beneath the seabed and pass this mixture into separators, where gas is mostly separated from oil and water (Section S1 and Fig. S1, ESI†). Natural gas is transported to shore via the export line, oil is generally transported to shore via pipeline, while water is treated and then transferred back to the ocean. Ideally, all gas lost through is either recovered or sent to a flare. A full description of gas routing is given in Section S1 and Fig. S1 (ESI†).
2.2. Venting
Sources.
A production platform vents NG for two primary reasons. (1) to control excessive pressures on drill boats when testing well performance; and (2) to vent excess gas during oil and gas processes on a production platform where gas recovery or flaring is not possible because the platform does not have suitable compressors (i.e. does not have vapor recovery units (VRUs)) or transport ability (e.g. pipelines to shore).
NAEI methodology.
The EFs used to generate the NAEI emission rates are not explicitly presented in NAEI documents, but were found in the EMEP/EEA Air Pollutant Emission Inventory Guidebook.32,33 They appear to have been obtained from three unpublished and publicly unavailable sources written between 1992 and 1994 and based on facilities in the UK, Canada and Russia.34–36 We identified UK venting EFs of 270 and 498 Mg CH4 facility−1 for oil-only and combined O&G facilities32 resulting in NAEI emissions of 24.6 Gg CH437 in 2019. Generally, a facility is defined as any floating or fixed platform structure that houses equipment used to extract hydrocarbons and transport them to storage facilities, transport vessels or pipelines to shore. As the primary sources of the EFs were unavailable, judging their quality is impossible.
Improved estimation technique.
To improve the NAEI emission estimate for venting, we use the 2019 vented emissions reported to the North Sea Transition Authority (NSTA) by O&G operators (112 Gg CH4).15 Typically, the volume of gas vented is measured using metering systems with an uncertainty of ±30% and the CH4 content in NG can vary by ±6%.32 We therefore use the root sum square of these values, ±31%, as the uncertainty in the gas vented annually.
2.3. Flaring
Sources.
As with vented emissions, some NG cannot be sent to the export pipelines and is instead sent to the flare on a production platform. Flaring converts hydrocarbons in NG to carbon dioxide, a less potent GHG gas. We assume that all NG lost from condensate tank flashing, vapor recovery units (VRUs), dehydrators, water tanks and compressors is routed to the low pressure (LP) flare, while upset conditions (over pressure of the separators or VRU failure) result in gas being sent to the high pressure (HP) flare (Section S1 and Fig. S1, ESI†).
NAEI methodology.
The NAEI EF, 0.011 Gg CH4 Gg−1 NG flared,38 was used to calculate a flaring emission estimate of 15.6 Gg CH437 in 2019. The NAEI EF is explicitly stated by the NAEI (Supplementary Material Table S1, ESI†), but does not appear in the EMEP document. Its heritage remains unknown32 and corresponds to a NG destruction efficiency (DE) of 0.989. The source of the activity data used to generate the flaring emission estimate is not reported in the NAEI database. The US EPA document referenced by the NAEI defines flare DE as 98% with an associated uncertainty of +2, −3%.39 The US EPA DE was first calculated using measurements made downwind of onshore flares in Tulsa, OK, USA in 198240 and Detroit, MI, USA in 2010,41 both experiments were conducted in wind speeds less than 2 m s−1. The average wind speed offshore in the UK in 2019 was 9.8 m s−1,42 with 95% confidence intervals of 4 m s−1 and 17 m s−1.
Improved estimation technique.
Research has reported that DE is likely influenced by the properties of the flare (i.e. flare gas temperature, gas flow rate, flare diameter, flare jet speed), the environmental conditions in which flaring takes place (i.e. wind velocity, precipitation, temperature, relative humidity, atmospheric pressure), gas composition details (i.e. CH4, VOC, CO2 and O2 composition) and physical condition of the flare (i.e. age, corrosion by salt, injectors blocked by soot).43–48 Here we adopt an algorithm that considers how environmental conditions could affect the flare efficiency. We neglect the effect of varying flaring technology and infrastructure.
Published research on DE is limited48 and is a field of research that has not been updated in 20 years. The only published, quantitative research on flare DE we could find, Johnson and Kostiuk (2002), used controlled NG emissions in a wind tunnel to generate empirical relationships between the cross-wind speed and DE. This study presents flare inefficiency (1-DE) as a function of the wind speed (u, m s−1), flare gas exit velocity (v, m s−1), acceleration due to gravity (g, m s−2), stack outside flare diameter (d, m) and lower heating value of CH4 (LHV, MJ kg−1) (eqn (1)).48 Johnson and Kostiuk (2002) presented coefficients A and B specific to the type of gas being flared.
| 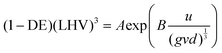 | (1) |
Assuming: (1) flare DE is 0.98 at
u = 2 m s
−1; (2) all other variables (
v,
g,
d and LHV) in
eqn (1) remain constant; (3)
A = 156.4 (MJ kg
−1)
3 and
B = 0.318 for natural gas;
48 and (4) the average
u offshore in the UK in 2019 was 9.8 m s
−1
42 (95% CIs of 4 m s
−1 and 17 m s
−1),
eqn (1) can be used to calculate an average DE of 0.905 (range 0.844 to 0.940).
2.4. Fugitive emissions - process and combustion emissions
Sources.
We define fugitive emissions as CH4 emitted from the process and combustion activities on a production platform during times of non-flaring and non-venting.
NAEI methodology.
Fugitive emissions are presented in the NAEI as offshore fuel combustion emissions and offshore process emissions and estimated at 3.5 and 3.8 Gg CH4 in 2019, respectively. NAEI combustion EFs are 2.4 and 0.18 kg CH4 TJ−1 of oil and NG produced, respectively.38 It is unclear how these EFs have been generated; they seem to be derived from the IPCC Tier 1 EFs for stationary combustion in the energy industry for crude oil and NG of 3 (range 1 to 10) and 1 (0.3 to 3) kg CH4 TJ−1, respectively.14 The IPCC EFs were established using the expert judgement of inventory experts for the 1996 IPCC Guidelines and are still considered valid.14
Improved estimation technique.
We estimate fugitive emissions from a typical offshore facility at 725 Mg CH4 year−1 from combustion (generators, export compressors) and processing (flash from the oil tanks, dehydrators and water treatment) activities. Total emissions include loss from power generation, export compressors, flash gas, dehydrators and water treatment. Facility power supply is typically provided by 50 MW NG turbines,50,51 gas slip from this type of gas turbine is estimated at 1.14 g CH4 MWh−1
52 which results in emissions of 292 Mg CH4 year−1. Similarly, slip from ten 1000 hp export compressors is estimated at 58 Mg CH4 year−1.52 Using the Vasquez–Beggs Solution gas/oil ratio correlation method,53 flash gas from a facility producing 450 m3 oil day−1 is estimated at 374 Mg CH4 year−1. Gas loss from glycol dehydrators is estimated at 276 scf CH4 MMscf−1 NG54 resulting in an average facility producing 119 kg CH4 year−1. Gas loss from water treatment is estimated at 0.415 g CH4 m−3 of produced water,55 an average water production of 1590 m3 water per day15 results in emissions of 200 kg CH4 year−1. If the total 725 Mg CH4 year−1 is routed to LP flare (DE of 98%), total emissions from 323 facilities are estimated at 15 Gg CH4 year−1. While we acknowledge that the numbers used to generate these emissions are based on several assumptions (flare DE, size of generator, number of compressors, and amount of oil, water and gas produced), these ideal values are twice as large as the NAEI estimate and indicate NAEI may be underestimating emissions.
Direct measurement of CH4 emissions from O&G production platforms in the North Sea estimated fugitive emissions during normal extraction operations of 0.19% of production, ranging from 0.04 to 1.41%.4 These emissions could have come from incomplete fuel combustion, equipment leaks or non-optimal operation on the working deck (turbines, engines, heaters, etc.).
2.5. Offshore oil loading
Sources.
Floating production storage and offloading (FPSO) installations are slightly different from the ideal platform. These offshore facilities extract oil and other liquid hydrocarbons, process them offshore, and store them until they are offloaded to a tanker. Methane loss can occur when oil is transferred to a tanker and is a function of the CH4 content in the oil, the movement of the vessels, and the temperature of the oil.33
NAEI methodology.
The EF used by the NAEI, 0.000018, was derived from operators’ data, discussed at the United Kingdom Offshore Operators Association (UKOOA) Atmospheric Emissions Working Group and subsequently published as a UKOOA internal document in 1995.56 This publication is not publicly available and the UKOOA did not respond when contacted about access. The 2019 NAEI estimate from offshore oil loading in the UK was 0.9 Gg.
It is unclear if the UK EF is representative. The CH4 emissions from Norwegian oil loading in 2016 were estimated to be 1.95 Gg (or 10% of the total emissions from upstream O&G production),33,57 compared to 0.95 Gg from offshore oil loading in the UK (or 2.4% of the total CH4 emissions from upstream O&G production). More information on the methods used to derive the current NAEI EF for offshore loading is needed to confirm or refute the apparent UK under/over-estimate of CH4 emissions from this source.
Improved estimation technique.
No direct measurement of CH4 loss from offshore oil loading has been reported and, in the absence of peer-reviewed evidence, we present the US Environmental Protection Agency's approach as an alternative.54 Based on an integrated measurement and modelling approach, the total loading loss of CH4 (CL, kg CH4/103 gallons of oil loaded onto a shuttle tanker) is a function of the true vapor pressure of loaded crude oil, (P, psia), the molecular weight of vapours (M, g mol−1), the vapor growth factor (G = 1.02), the temperature of vapours (T, °R) and the arrival EF (CA, kg CH4/103 gallons of oil loaded) (eqn (2)). This algorithm has been developed by the US EPA to estimate emissions for the loading of crude oil into ships and ocean barges. |  | (2) |
Both P and T are temperature dependent and emissions are higher at higher temperatures, the average temperature in the North Sea varies from 5 to 15 °C. The value of CA depends on the condition of the loading and ranges from 0.33 for a clean boat to 0.86 for an uncleaned ship that had a volatile previous cargo. Taking the values from the EPA document,54 the average CA is 0.199 kg CH4/103 gallons of oil loaded, while at 5 °C CA is 0.181 kg CH4/103 gallons of oil loaded and at 15 °C is 0.457 kg CH4/103 gallons of oil loaded into an uncleaned ship.
2.6. Natural gas transfer by high-pressure pipelines
Sources.
In the UK, most NG is transported from offshore installations to gas terminals using a 45
000 km58 long network of high-pressure transmission pipelines. Gas enters the national transmission network from which it either goes to the distribution network or is stored. Storage facilities include salt caverns, onshore LNG storage sites, and depleted onshore gas fields.59
NAEI methodology.
For 2019 the NAEI estimated CH4 leakage from NG transmission at 3 Gg CH4 corresponding to an EF of 103 kg CH4 leaked Gg−1 NG transported (0.01% loss of gas transported or 67 kg CH4 km−1 pipeline year−1), assuming 29
000 Gg CH4 was produced offshore in 2019.1 This does not account for the transportation of non-UK produced gas. The NAEI EF is claimed to derive from, but not equal to, the EMEP EF of 920 kg of CH4 leaked Gg−1 NG transported (0.092% or 600 kg CH4 km−1 pipeline year−1), which is based on data from the Corinair 1990 database.33
IPCC EFs for pipelines are 130, 1300 and 13
000 kg CH4 km−1 pipeline year−1 for high, medium and low quality pipelines, taken from currently unpublished work by the International Gas Union, and based on data for a dozen countries including Russia and Algeria,14 and suggest the 1990 NAEI EF may be an underestimate. More recent studies estimate CH4 loss from transmission pipelines in the US at between 0.07 and 0.8%,60,61 while CH4 losses from Russian pipelines are estimated at 1.4%.62,63
Updated estimation technique.
To generate a representative emission estimate from NG transport from pipelines we will use the observations of Stephenson et al. (2011). This study was chosen as it was conducted in the US and the most analogous to the UK of the published studies.14,60–63 Stephenson et al. (2011) estimate the CH4 losses from pipelines at 0.07% with an uncertainty range of ±23%.
3. Results
3.1. Reassessed 2019 emission inventory
We present our reassessed 2019 estimates for venting, flaring, fugitive emissions, offshore oil transfer and transfer by pipeline here and compare our estimates with the NAEI CH4 leakage estimates (Table 1). Our estimated total emission for the 2019 upstream UK oil and gas production and transmission activities is 289 Gg CH4 year−1, with an uncertainty range of 112 to 1181 Gg CH4 year−1. The NAEI emission estimate for the same year was 52 Gg CH4 year−1.
Table 1 2019 methane emission estimates for upstream UK oil and gas production and transmission activities. Emissions reported in the UK National Atmospheric Emissions Inventory (NAEI) are compared with emissions calculated using the improved integrated approach detailed in this study. Emissions are presented as Gg CH4 year−1 and Loss as the % of production
Activity |
NAEI Gg year−1 |
EF date |
Improved approach Gg year−1 |
Source date |
Combination of process and fuel combustion emissions.
EF based on non-peer-reviewed and publicly unavailable literature or expert opinion.
EF based on non-peer-reviewed and publicly available literature.
EF derived from on non-peer-reviewed and publicly available literature, but it is not clear how EFs were calculated.
Actual O&G operator's data.
Calculation based on peer-reviewed study and O&G operator's activity data.
Calculation based on peer-reviewed study and activity data derived from the NAEI estimate.
Calculation based on US EPA EF and activity data derived from the NAEI estimate.
|
Venting |
25b |
1992 |
112 (78–146)e |
2020 |
Flaring |
16c |
1982 |
74 (65–169)f |
2002 |
Fugitivea |
7d |
1996 |
76 (9–831)g |
2020 |
Off. oil |
1b |
1995 |
1 (1–2)h |
2008 |
Pipeline |
3d |
1990 |
26 (19–33)g |
2012 |
Total emission |
52 |
|
289 (172–1181) |
|
Loss |
0.13 |
|
0.72 (0.43–2.94) |
|
3.1.1. Venting.
Our 2019 CH4 emissions estimate for offshore venting, as reported by NSTA, is 112 Gg CH4 (range 78 to 146 Gg CH4).15 The NSTA data suggests the NAEI currently underestimates vented CH4 emissions (NAEI estimate 25 Gg CH4 year−1) and, as the NSTA data are reported by operators, it strongly suggests that the fixed EFs of 270 and 498 Mg CH4 facility−1 for oil and O&G facilities, respectively, do not adequately estimate vented emissions from modern offshore facilities. Venting is the largest source of offshore emissions in our reassessed estimate (Table 1).
3.1.2. Flaring.
Using the destruction efficiency (DE), calculated by eqn (1), and data supplied by O&G operators,48 our 2019 estimate for CH4 emissions from offshore flaring is 74 Gg CH4 (NAEI estimate 16 Gg CH4 year−1). Our estimate assumes all UK offshore installations’ flares are optimally physically efficient, i.e. have not degraded with age, are not corroded by salt, injectors are not blocked by soot and gas/flare jet velocities are optimized.43,48 The DE of flares at O&G facilities have been reported by several studies. Onshore, Chambers et al. (2003) used a differential absorption LIDAR to calculate the DE from six flares in Alberta, Canada ranging from 0.55 to 0.98 with a mean DE of 0.84.49 Offshore, aircraft measurements of facilities in the Gulf of Mexico flaring 150 MMscf d−1 NG estimated a mean regional emission of 2800 kg CH4 h−1, corresponding to a regional DE of 0.94.46 Here we note, the average wind speed during the offshore measurements in Mexico was 6.7 m s−1 and corresponds to a DE of 0.94 using eqn (1).46 Both studies suggest the DE is not constant and wind speed should be accounted for, supporting the implementation of eqn (1).
3.1.3. Fugitive emissions.
Using an EF of 0.0019 Gg CH4 Gg−1 NG produced and the 2019 total UK gas production of 40 Tg CH4,15 we estimate total offshore fugitive CH4 emissions at 76 Gg CH4 (NAEI estimate 7 Gg CH4 year−1). The least (0.04%) and most (1.41%) emissive facilities observed by Riddick et al. (2019) coupled to the measurement uncertainty of ±45% are used as the upper and lower uncertainty bounds and propagate forward to emission bounds of 9 and 831 Gg CH4 year−1.
3.1.4. Offshore oil transfer.
Oil produced on UK FPSOs in 2019 has been estimated at 2.2 million m3
15 and emissions from offshore oil loading have been estimated at 1.1 Gg CH4 year−1, range 1.0 to 2.4 Gg CH4 year−1 (NAEI estimate 1 Gg CH4 year−1). The emission estimate calculated using the US EPA approach (eqn (2)) is within the uncertainty bounds of the NAEI estimate. This validates the approach used by the NAEI, although direct measurements could be used to confirm the emission estimates.
3.1.5. Transfer by pipeline.
The most conservative, published measurement of gas loss by pipelines, 0.07%,59,60 results in an emission estimate of 25.9 Gg CH4 year−1 (or 575 kg CH4 km−1 pipeline year−1) with an uncertainty range of 18.6 to 33.1 Gg CH4 year−1 (NAEI estimate 3 Gg CH4 year−1).
3.2. 2019 Revised emission estimates
Table 1 presents CH4 emission estimates from the NAEI and from this study's improved integrated assessment approach. The NAEI reported total 2019 CH4 emissions from upstream O&G operations to be 52 Gg with venting being the largest source of emissions (Table 1). Most of the emission estimates are derived using a bottom up approach that takes 30 to 40 year-old EFs from available unpublished literature (flaring and loss in pipelines), unavailable unpublished literature (venting and offshore oil unloading) or expert opinion (fugitive emissions). Our improved integrated approach uses the findings from more recent, publicly available research to infer CH4 emissions from the extraction and transmission processes from the 323 production platforms and 66 FPSOs listed as operational in the UK in 2019.15 We find emissions to be 289 Gg CH4 (range: 172 to 1181 Gg CH4), more than five times larger than the NAEI estimate (Table 1).
Venting is the largest source of offshore emissions. The emission estimate we used for venting (112 Gg CH4 year−1) taken directly from a UK Government database15 is and more than twice the NAEI total emission (52 Gg CH4 year−1), which are derived using an emission factor. Emissions from combustion and processing activities on production platforms are combined to form fugitive emissions of 76 Gg CH4 year−1 and are based on direct measurement of emissions from offshore platforms in the North Sea.4 Offshore oil unloading emissions of 1 Gg CH4 year−1 were calculated from an algorithm generated by the US EPA and the amount of oil produced by FSPO. Methane emissions from high pressure pipelines are estimated at 26 Gg CH4 year−1, based on an estimate of 575 kg CH4 km−1 pipeline year−1, this value seems reasonable as it suggests the pipelines are of medium to high quality, as identified by the IPCC. Overall, the total emission estimate calculated using recent EFs is over five times larger than the NAEI estimate of 52 Gg CH4.
3.3. Global variability in methane leakage from oil and gas operations
The UK provides annual estimates of the volume of CH4 flared or vented, a clear government flaring/venting policy, and a governmental regulatory body overseeing emission targets, legislation, regulation and monitoring strategies.1,15,64–66 Globally, only Alberta, Canada better regulates CH4 emissions than the UK, using legislation specifying the quantity of gas that can be flared or vented from onshore production.66
The UK was the first major economy to pass a net zero emissions law in 2019 which set a target requiring the UK to bring all greenhouse gas emissions to net zero by 2050.67 In 2021 the UK passed another law that requires carbon emissions be reduced by 78% by 2035 compared to 1990 levels.68 The UK aims to reduce upstream CH4 emissions by: (1) minimizing purge flow vent systems; (2) maximizing flash gas recovery; (3) measuring CH4 emissions using drone sensor surveys and infra-red detection; plus a variety of undefined strategies including: new projects, new techniques, reprioritizing operations (on/offshore), use of digital dashboards, digital machine learning and artificial intelligence technology.69
To gain an understanding of how emissions may vary regionally, facilities around the world are presented in Section S4 (ESI†) grouped into regions with data collated on regional regulation (Section S4, ESI†).64–66 In general, ∼40% of platforms must comply with venting, flaring and leak detection and repair (LDAR) regulations (we have assigned these Category 1 status), 10% of platforms have flaring regulations but no regulation on venting or LDAR (Category 2 status) and we estimate ∼50% of global platforms do not have any regulations controlling their venting, flaring or LDAR practices (Category 3 status).
We suggest Category 1 represents the base-case, similar to regulations in the UK, with CH4 emissions of 0.97% of NG production (Table 2). Category 2 platforms have venting and flaring rates similar to the UK, but gas from process and combustion activities are likely vented to the atmosphere and result in CH4 emissions equal to 1.28% of NG production. Category 3 platforms are a worst-case scenario where CH4 is freely vented to the atmosphere, resulting in the emission of 10.3% of production.
Table 2 Comparison of the loss (% of production) and emissions (Em; Gg CH4 year−1) from an “average” offshore platform producing 400 Gg CH4 year−1 in each of the 3 regulatory Categories (Cat) as shown in Section S3 (ESI)
Source |
Cat 1 |
Cat 2 |
Cat 3 |
|
Loss |
Em |
Loss |
Em |
Loss |
Em |
Venting |
0.28 |
112 |
0.28 |
112 |
9.50 |
3812 |
Flaring |
0.18 |
74 |
0.18 |
74 |
0.00 |
0 |
Fugitive |
0.19 |
76 |
0.58 |
234 |
0.58 |
234 |
Offshore oil |
0.00 |
1 |
0.00 |
0 |
0.00 |
1 |
Pipeline |
0.06 |
26 |
0.06 |
26 |
0.06 |
26 |
Total |
0.72 |
289 |
1.11 |
446 |
10.32 |
4141 |
4. Discussion and conclusions
4.1. Reassessment of CH4 emission for upstream UK oil and gas operations
The 2019 NAEI estimated total emissions from upstream O&G operations (venting, flaring, process emissions, fuel combustion, offshore oil loading, transfer by pipeline and onshore oil/gas terminals) at 52 Gg CH4. Our integrated approach, which uses direct measurements and top-down studies and published data, estimates 2019 CH4 emissions at 289 Gg CH4, five times the current NAEI estimate. This may be a lower bound estimate as (i) venting in the North Sea is reported to have increased from 5 Gg CH4 year−1 in 2016 to 136 Gg CH4 year−1 in 2020 (reported as 112 Gg CH4 year−1 in 2019) despite little change in oil or gas production15 (ii) although we assume flares operate at optimal efficiency it is likely they are not optimized46,48,49 and (iii) we assume that CH4 combustion slip from compressors, VRUs and condensate tanks are routed to the flare which is unlikely to occur.70 Total emissions calculated here are in-line with recent preindustrial carbon-14 estimates that indicate present day fossil CH4 emissions are underestimated by up to 40%71 and are consistent with satellite observation of the Permian Basin in the US that suggest US EPA underestimates emissions by a factor of six.72
4.2. Policy Implications
Accurate estimates of GHG emissions are fundamental to accurate projections of climate change in Earth system models and critical in identifying optimal mitigation targets/strategies.10 The United Kingdom has joined over 100 countries in the Global Methane Pledge to reduce CH4 emissions by at least 30% from 2020 levels by 203011. Reducing emissions from offshore oil rigs will be critical to fulfil this pledge. The difference between current estimates used by NAEI and our estimates, which use more recent research findings, strongly suggests that the current methods of compiling national GHG inventories in the UK, and likely elsewhere, are outdated (oldest EF derived in 1982) and systematically underestimate emissions.10,20 Inventories, such as the NAEI, that rely on EFs obtained from industrial studies and unpublished research that has not been independently validated, require improvement especially as they are currently used to inform the IPCC and will be used in evaluating Methane Pledge commitments. The upstream O&G industry operates in challenging conditions and at the limit of where in situ measurements can realistically be made. Remote sensing of CH4 emissions from offshore facilities is in development and quantification methods have not yet been fully validated.30,31 Direct measurement of emissions from offshore remote production platforms in a range of weather conditions presents significant challenges but given the relative size of the potential emissions, it is important that these emissions be better constrained and more accurately reported in national inventories in order to receive appropriate attention for mitigation. Emission factors should be improved and activity levels for various processes reported transparently.
We estimate that the UK loses 289 Gg CH4 year−1 from upstream O&G production. Our results indicate that the NAEI emissions, which are reported and independently verified by the BEIS, are underestimated by a factor of five. Thus, the impact of UK upstream O&G CH4 emissions on global climate is underestimated and a clear indication of the largest sources and most beneficial mitigation strategies is lacking. Currently, the emission estimates generated by the NAEI are too uncertain to be used in GHG emissions auditing, such as reporting to the IPCC.
Currently, global CH4 emissions from the O&G sector are estimated at 1.6 Tg CH4 year−1, based on a 0.32% baseline leakage rate suggested by the OGCI.73 If global emissions from upstream O&G operations are similar to the UK, i.e. 0.72% of production, they would be approximately five times higher than currently estimated, i.e. 3.6 Tg CH4 year−1. However, the UK estimate in this study is based on UK venting, flaring and LDAR regulations and may not apply to production platforms globally. Rather than the OGCI estimate of 0.32% of production lost, we suggest global CH4 emission from the oil and gas sector could lie between 0.72% (Category 1) and 10% (Category 3) of production, depending on where the gas is extracted.
Although the emissions presented in this study are substantially higher than those currently reported, they present high yield opportunities for mitigation as long as baseline emissions are estimated in a clear and transparent way. Given that net CH4 emissions are a small residual of a large source and sink74 and CH4 has an atmospheric lifetime of ∼12 years, reducing overall leakage by a relatively small percentage could result in a significant reduction in atmospheric concentrations,75,76 a resulting reduction in radiative forcing from CH4, a reduction in the rate of climate warming, and a reduction in the formation of tropospheric ozone which has detrimental effects on human health and vegetation.
4.3. Looking ahead
This study highlights the importance of improving the accuracy of CH4 emission inventories in the UK and globally. The use of direct measurements to improve CH4 emission estimates by generating realistic EFs and activity levels is essential. Direct-measurement techniques are under development by the UK Government and the OGCI.77 In addition to directly improving emission estimates, additional measurements that improve current EFs and generate EFs for previously overlooked processes are also needed.
Detecting CH4 leakage from offshore oil and gas platforms would benefit from improved remote sensing of CH4 over water. Several technologies are making progress with this. The recent development of capturing sun glint reflection from water surrounding the observation target has allowed for offshore CH4 emission quantification using airborne imaging spectrometers in 202131 and satellites in 2022.78 The major shortcoming of these remote sensing technologies is that quantification thresholds are relatively high, 10+ kg CH4 h−1 for aircraft and 100+ kg CH4 h−1 for satellites, and the duration of measurement is very short, less than 10 s for satellites. This means that remote sensing could be used for detecting inefficient flares, large leaks on platforms or very large pipeline leaks, but unlikely to currently be able to quantify leaks with varying rates or smaller continuous leaks. Such methods are also unlikely to observe the majority of offshore emissions, i.e. typically short-duration venting or offshore oil unloading. Continuous monitors would be better at quantifying these smaller intermittent emissions, but to date only one detection system, the Honeywell Rebellion gas cloud imager, has achieved Intrinsically Safe (IS) status. IS certification is expensive and required for a technology to be permitted on an offshore platform. Given that offshore production is a relatively small market (∼1300 offshore platforms worldwide) and many countries do not regulate emissions, the cost of IS status is high. Offshore environments are generally harsh on technology which has meant that offshore continuous monitoring has not been appealing to other system developers. This is likely to remain the case until there is a significant financial incentive for research, development and deployment of sensors suited to the harsh conditions found off-shore.
As countries around the world recognize the importance of reducing CH4 leakage to slow the rate of climate change and attempt to meet the Global Methane Pledge to reduce CH4 emissions by at least 30% from 2020 levels by 2030, funding of efforts to improve detection of CH4 leakage via remote sensing should increase. As a participant in the Global Methane Pledge, with most of its leakage occurring off-shore, the UK is a logical contributor to improve technology for off-shore CH4 leakage, monitoring and reporting. Such efforts would increase the accuracy of its greenhouse gas emission inventory.
As described in the introduction, one common issue with currently available top-down emission quantification methods is that, even though they work over land, it is unclear how well they will work offshore. Onshore leak detection and quantification methods are routinely tested at controlled release sites, such as Colorado State University's Methane Emission Technology Evaluation Center (METEC). Offshore measurements will remain highly uncertain until the methods are tested against controlled releases from offshore facilities. Given the magnitude of emissions indicated by our research, we suggest that the quantification and mitigation of offshore CH4 emissions should be a UK priority.
Author contributions
S. N. R. conceived and designed the study, conducted the formal analysis and wrote the original manuscript. D. L. M. acquired funding, validated results, and edited and helped write the final manuscript.
Conflicts of interest
There are no conflicts to declare.
Acknowledgements
We thank Princeton University's Science, Technology, and Environmental Policy (STEP) program for research support. We also thank, Daniel Varon (Harvard University), Michael Celia (Princeton University), Mary Kang (McGill University), Neil Harris (Cranfield University) and Michelle Cain (Cranfield University) for comments on earlier versions of the manuscript.
Notes and references
- BEIS, Department for Business, Energy & Industrial Strategy. UK Energy Statistics, Statistical Press Release 2019 & Q4 2019, https://assets.publishing.service.gov.uk/government/uploads/system/uploads/attachment_data/file/877047/Press_Notice_March_2020.pdf, (accessed October 25, 2022).
- DUKES, Digest of UK Energy Statistics (DUKES): Natural Gas Chapter 4. DUKES chapter 4: statistics on supply and demand for natural gas. Natural gas imports and exports DUKES 4.5, https://assets.publishing.service.gov.uk/government/uploads/system/uploads/attachment_data/file/1094426/DUKES_4.5.xlsx, (accessed October 25, 2022).
- M. Omara, M. R. Sullivan, X. Li, R. Subramanian, A. L. Robinson and A. A. Presto, Environ. Sci. Technol., 2016, 50, 2099–2107 CrossRef CAS PubMed.
- S. N. Riddick, D. L. Mauzerall, M. Celia, N. R. P. Harris, G. Allen, J. Pitt, J. Staunton-Sykes, G. L. Forster, M. Kang, D. Lowry, E. G. Nisbet and A. J. Manning, Atmos. Chem. Phys. Discuss., 2019, 1–14 Search PubMed.
- T. L. Vaughn, C. S. Bell, C. K. Pickering, S. Schwietzke, G. A. Heath, G. Pétron, D. J. Zimmerle, R. C. Schnell and D. Nummedal, Proc. Natl. Acad. Sci. U. S. A., 2018, 115, 11712–11717 CrossRef CAS PubMed.
- D. J. Zimmerle, L. L. Williams, T. L. Vaughn, C. Quinn, R. Subramanian, G. P. Duggan, B. Willson, J. D. Opsomer, A. J. Marchese, D. M. Martinez and A. L. Robinson, Environ. Sci. Technol., 2015, 49, 9374–9383 CrossRef CAS PubMed.
- IPCC, Summary for Policymakers—Special Report: Global Warming of 1.5 oC, https://www.ipcc.ch/sr15/chapter/spm/, (accessed October 25, 2022).
- IPCC, AR5 Climate Change 2014, https://www.ipcc.ch/report/ar5/wg3/, (accessed October 25, 2022).
- UNEP/CCAC, United Nations Environment Programme and Climate and Clean Air Coalition (2021), Global Methane Assessment: Benefits and Costs of Mitigating Methane Emissions, Nairobi: United Nations Environment Programme., https://www.ccacoalition.org/en/resources/global-methane-assessment-full-report, (accessed October 25, 2022).
- E. G. Nisbet, R. E. Fisher, D. Lowry, J. L. France, G. Allen, S. Bakkaloglu, T. J. Broderick, M. Cain, M. Coleman, J. Fernandez, G. Forster, P. T. Griffiths, C. P. Iverach, B. F. J. Kelly, M. R. Manning, P. B. R. Nisbet-Jones, J. A. Pyle, A. Townsend-Small, A. al-Shalaan, N. Warwick and G. Zazzeri, Rev. Geophys., 2020, 58, e2019RG000675 CrossRef.
- Global Methane Pledge, Global Methane Pledge - Fast action on methane to keep a 1.5 °C future within reach, http://www.globalmethanepledge.org, (accessed September 15, 2022).
- EEMS, Environmental Emissions Monitoring System. Atmospheric Emissions Calculation, https://assets.publishing.service.gov.uk/government/uploads/system/uploads/attachment_data/file/136461/atmos-calcs.pdf, (accessed October 25, 2022).
- UNFCCC, Paris Agreement. United Nations Framework Convention on Climate Change. FCCC/CP/2015/L.9/Rev.1, https://unfccc.int/documents/9064, (accessed October 25, 2022).
- IPCC, IPCC Guidelines for National Greenhouse Gas Inventories., https://www.ipcc-nggip.iges.or.jp/public/2006gl/pdf/1_Volume1/V1_3_Ch3_Uncertainties.pdf, (accessed February 18, 2019).
- OGA, UK Oil and Gas Authority, https://www.arcgis.com/apps/webappviewer/index.html?id=f4b1ea5802944a55aa4a9df0184205a5, (accessed October 25, 2022).
- BEIS, UK greenhouse gas emissions statistics. Historical UK greenhouse gas emissions, https://www.gov.uk/government/collections/uk-greenhouse-gas-emissions-statistics, (accessed October 25, 2022).
- D. A. Turner, I. D. Williams and S. Kemp, Resour., Conserv. Recycl., 2015, 105, 186–197 CrossRef.
- C. E. P. Cerri, X. You, M. R. Cherubin, C. S. Moreira, G. S. Raucci, B. de, A. Castigioni, P. A. Alves, D. G. P. Cerri, F. F. de, C. Mello and C. C. Cerri, PLoS One, 2017, 12, e0176948 CrossRef PubMed.
- W.-B. Yang, C.-S. Yuan, W.-H. Chen, Y.-H. Yang and C.-H. Hung, Aerosol Air Qual. Res., 2017, 17, 2608–2623 CrossRef CAS.
- E. Nisbet and R. Weiss, Science, 2010, 328, 1241–1243 CrossRef CAS PubMed.
- S. N. Riddick, B. R. Hancock, A. D. Robinson, S. Connors, S. Davies, G. Allen, J. Pitt and N. R. P. Harris, Waste Manage., 2018, 73, 210–219 CrossRef CAS PubMed.
- S. N. Riddick, S. Connors, A. D. Robinson, A. J. Manning, P. S. D. Jones, D. Lowry, E. Nisbet, R. L. Skelton, G. Allen, J. Pitt and N. R. P. Harris, Atmos. Chem. Phys., 2017, 17, 7839–7851 CrossRef CAS.
-
H. D. van der Gon, S. Beevers, A. D’Allura, S. Finardi, C. Honoré, J. Kuenen, O. Perrussel, P. Radice, J. Theloke, M. Uzbasich and A. Visschedijk, in Air Pollution Modeling and its Application XXI, ed. D. G. Steyn and S. Trini Castelli, Springer, Netherlands, 2012, pp. 199–204 Search PubMed.
- R. Edie, A. M. Robertson, R. A. Field, J. Soltis, D. A. Snare, D. Zimmerle, C. S. Bell, T. L. Vaughn and S. M. Murphy, Atmos. Meas. Tech., 2020, 13, 341–353 CrossRef CAS.
- J. L. France, P. Bateson, P. Dominutti, G. Allen, S. Andrews, S. Bauguitte, M. Coleman, T. Lachlan-Cope, R. E. Fisher, L. Huang, A. E. Jones, J. Lee, D. Lowry, J. Pitt, R. Purvis, J. Pyle, J. Shaw, N. Warwick, A. Weiss, S. Wilde, J. Witherstone and S. Young, Atmos. Meas. Tech., 2021, 14, 71–88 CrossRef CAS.
- T. I. Yacovitch, C. Daube and S. C. Herndon, Environ. Sci. Technol., 2020, 54, 3530–3538 CrossRef CAS PubMed.
- B. K. Lamb, J. B. McManus, J. H. Shorter, C. E. Kolb, B. Mosher, R. C. Harriss, E. Allwine, D. Blaha, T. Howard, A. Guenther, R. A. Lott, R. Siverson, H. Westburg and P. Zimmerman, Environ. Sci. Technol., 1995, 29, 1468–1479 CrossRef CAS PubMed.
- S. Conley, I. Faloona, S. Mehrotra, M. Suard, D. H. Lenschow, C. Sweeney, S. Herndon, S. Schwietzke, G. Pétron, J. Pifer, E. A. Kort and R. Schnell, Atmos. Meas. Tech., 2017, 10, 3345–3358 CrossRef CAS.
-
A. Ayasse, R. M. Duren, A. K. Thorpe, D. Cusworth, E. A. Kort, A. Gorchov Negron, J. Heckler and G. Asner, Methane Plume Mapping Over Offshore Oil and Gas platforms Using Sun Glint, AGU Fall Meeting, New Orleans, 2021 Search PubMed.
- I. Irakulis-Loitxate, J. Gorroño, D. Zavala-Araiza and L. Guanter, Environ. Sci. Technol. Lett., 2022, 9, 520–525 CrossRef CAS.
- A. K. Ayasse, A. K. Thorpe, D. H. Cusworth, E. A. Kort, A. G. Negron, J. Heckler, G. Asner and R. M. Duren, Environ. Res. Lett., 2022, 17, 084039 CrossRef.
- EMEP/EEA, EMEP/EEA air pollutant emission inventory guidebook 2019, https://op.europa.eu/publication/manifestation_identifier/PUB_THAL19015ENN, (accessed October 25, 2022).
-
H. Brown and D. Root, EEA, EMEP/EEA air pollutant emission inventory guidebook 2016: technical guidance to prepare national emission inventories, 2016.
- Brown and Root, Environmental atmospheric emissions from UK oil and gas exploration and production facilities in the continental shelf area, United Kingdom Offshore Association Limited.
- SRI, Scientific Research Institute of Atmospheric Air Protection. Emissions of hydrocarbons in gas industry, oil production industry, gas-and oil refining industries of Russia, Clearstone Engineering Ltd.
- D. J. Picard, B. D. Ross and D. W. H. Koon, A detailed inventory of CH4 and VOC emissions from upstream oil and gas operations in Alberta, Clearstone Engineering Ltd., 1992 Search PubMed.
- NAEI, UK greenhouse gas emissions statistics, https://naei.beis.gov.uk/data/data-selector?q=152412, (accessed October 25, 2022).
- DEFRA, UK National Atmospheric Emissions Inventory (NAEI) Data - Defra, UK, https://naei.beis.gov.uk/data/.Lastaccessed1/4/21, (accessed August 12, 2021).
- US EPA, U.S. Environmental Protection Agency, AP-42: Compilation of Air Emissions Factors, 2018, https://www3.epa.gov/ttn/chief/ap42/ch13/final/C13S05_02-05-18.pdf, (accessed October 25, 2022).
-
M. McDaniel, Flare efficiency study, U.S. Environmental Protection Agency, Washington, D.C., EPA/600/2-83/052 (NTIS PB83261644), 1983 Search PubMed.
-
B. Ewing, D. Roesler and S. Evans, Performance Test of a Steam-Assisted Elevated Flare with Passive FTIR - Detroit - Clean Air Engineering, Inc. Project No: 10971, 2010, https://downloads.regulations.gov/EPA-HQ-OAR-2012-0133-0047/attachment_15.pdf, (accessed November 1, 2022).
- B. Geyer, R. Weisse, P. Bisling and J. Winterfeldt, J. Wind Eng. Ind. Aerodyn., 2015, 147, 18–29 CrossRef.
- D. T. Allen, D. Smith, V. M. Torres and F. C. Saldaña, Curr. Opin. Chem. Eng., 2016, 13, 119–123 CrossRef.
- D. R. Caulton, P. B. Shepson, M. O. L. Cambaliza, D. McCabe, E. Baum and B. H. Stirm, Environ. Sci. Technol., 2014, 48, 9548–9554 CrossRef CAS PubMed.
- M. Faruolo, A. Caseiro, T. Lacava and J. W. Kaiser, IEEE Geosci. Remote Sens. Mag., 2021, 9, 258–281 Search PubMed.
- D. Zavala-Araiza, M. Omara, R. Gautam, M. L. Smith, S. Pandey, I. Aben, V. Almanza-Veloz, S. Conley, S. Houweling, E. A. Kort, J. D. Maasakkers, L. T. Molina, A. Pusuluri, T. Scarpelli, S. Schwietzke, L. Shen, M. Zavala and S. P. Hamburg, Environ. Res. Lett., 2021, 16, 024019 CrossRef CAS.
- A. Gvakharia, E. A. Kort, A. Brandt, J. Peischl, T. B. Ryerson, J. P. Schwarz, M. L. Smith and C. Sweeney, Environ. Sci. Technol., 2017, 51, 5317–5325 CrossRef CAS PubMed.
- M. R. Johnson and L. W. Kostiuk, Proc. Combust. Inst., 2002, 29, 1943–1950 CrossRef CAS.
-
A. Chambers, Well test flare plume monitoring: results of DIAL measurements in Alberta. Petroleum Technology Alliance Canada Calgary, AB. 1-48, The PTAC Air Issues Research Forum: research priorities, discussion, and networking reception 19 Nov 2003, https://www.osti.gov/etdeweb/biblio/20434087, (accessed October 25, 2022).
- S. Oliveira-Pinto, P. Rosa-Santos and F. Taveira-Pinto, Energy Convers. Manag., 2019, 186, 556–569 CrossRef.
- M. Korpås, L. Warland, W. He and J. O. G. Tande, Energy Procedia, 2012, 24, 18–26 CrossRef.
- US EPA, United States Environmental Protection Agency, AP 42, Fifth Edition, Volume I Chapter 3: Stationary Internal Combustion Sources, 3.1 Stationary Gas Turbines., https://www3.epa.gov/ttnchie1/ap42/ch03/final/c03s01.pdf, (accessed October 25, 2022).
- OK DEQ, Vasquez–Beggs Solution gas/oil ratio correlation method, Oklahoma Department of Environmental Quality, https://www.deq.ok.gov/wp-content/uploads/air-division/PG_VOC_Emissions_Flashing_Losses.pdf, (accessed October 25, 2022).
- US EPA, United States Environmental Protection Agency, AP 42, Fifth Edition, Volume I Chapter 5: Petroleum Industry. 5.2 Transportation and Marketing of Petroleum Liquids.
- S. Yang, W. Yang, G. Chen, X. Fang, C. Lv, J. Zhong and L. Xue, J. Environ. Sci., 2016, 46, 101–108 CrossRef CAS PubMed.
- UKOOA, https://uk-air.defra.gov.uk/assets/documents/reports/empire/naei/annreport/annrep96/app1_29.htm, (accessed November1, 2022).
- NOG, The Norwegian Oil and Gas Association Environmental Report 2017, https://www.norskoljeoggass.no/contentassets/b3bdff43b7ef4c4da10c7db1f3b782e4/environmental-report-2017.pdf, (accessed October 25, 2022).
- OGTC, Oil and Gas Technology Centre. HS413 – Phase 1 Project Report., https://assets.publishing.service.gov.uk/government/uploads/system/uploads/attachment_data/file/866379/Phase_1_-_OGTC_-_Hydrogen_Offshore_Production.pdf, (accessed October 25, 2022).
- OFGEM, GB Gas Storage Facilities, https://www.ofgem.gov.uk/sites/default/files/docs/2019/01/181207_storage_update_website.pdf, (accessed October 25, 2022).
- T. Stephenson, J. E. Valle and X. Riera-Palou, Environ. Sci. Technol., 2011, 45, 10757–10764 CrossRef CAS PubMed.
- C. L. Weber and C. Clavin, Environ. Sci. Technol., 2012, 46, 5688–5695 CrossRef CAS PubMed.
- S. Lechtenböhmer, C. Dienst, M. Fischedick, T. Hanke, R. Fernandez, D. Robinson, R. Kantamaneni and B. Gillis, Int. J. Greenhouse Gas Control, 2007, 1, 387–395 CrossRef.
- J. Lelieveld, S. Lechtenböhmer, S. S. Assonov, C. A. M. Brenninkmeijer, C. Dienst, M. Fischedick and T. Hanke, Nature, 2005, 434, 841–842 CrossRef CAS PubMed.
- GGFR, Global Gas Flaring Reduction Partnership, Global gas flaring tracker report, April 2021, Accessed 12/28/21., https://thedocs.worldbank.org/en/doc/1f7221545bf1b7c89b850dd85cb409b0-0400072021/original/WB-GGFR-Report-Design-05a.pdf, (accessed October 25, 2022).
- World Bank Group., Regulation of associated gas flaring and venting: a global overview and lessons from international experience (English). Global gas flaring reduction - a public-private partnership: No. 3 Washington, D.C.: World Bank Group., https://documents1.worldbank.org/curated/ar/590561468765565919/pdf/295540Regulati1aring0no10301public1.pdf, (accessed October 25, 2022).
- World Bank Group., Guidance on upstream flaring and venting: policy and regulation (English). Global Gas Flaring Reduction (GGFR) Washington, D.C.: World Bank Group., https://documents1.worldbank.org/curated/ar/590561468765565919/pdf/295540Regulati1aring0no10301public1.pdf, (accessed October 25, 2022).
- UK Govt, UK Government. Department for Business, Energy & Industrial Strategy. UK becomes first major economy to pass net zero emissions law. New target will require the UK to bring all greenhouse gas emissions to net zero by 2050., https://www.gov.uk/government/news/uk-becomes-first-major-economy-to-pass-net-zero-emissions-law, (accessed October 25, 2022).
- UK Govt, UK Government. Department for Business, Energy & Industrial Strategy. UK enshrines new target in law to slash emissions by 78% by 2035. The UK's sixth Carbon Budget will incorporate the UK's share of international aviation and shipping emissions for the first time, to bring the UK more than three-quarters of the way to net zero by 2050., https://www.gov.uk/government/news/uk-enshrines-new-target-in-law-to-slash-emissions-by-78-by-2035, (accessed October 25, 2022).
- OGUK, The UK Oil and Gas Industry Association Limited. UK upstream oil and gas sector. Pathway to a net-zero basin: production emissions targets., https://oguk.org.uk/wp-content/uploads/2020/09/OGUK-Production-Emissions-Targets-Report-2020-1.pdf, (accessed October 25, 2022).
- D. H. Cusworth, R. M. Duren, A. K. Thorpe, W. Olson-Duvall, J. Heckler, J. W. Chapman, M. L. Eastwood, M. C. Helmlinger, R. O. Green, G. P. Asner, P. E. Dennison and C. E. Miller, Environ. Sci. Technol. Lett., 2021, 8, 567–573 CrossRef CAS.
- B. Hmiel, V. V. Petrenko, M. N. Dyonisius, C. Buizert, A. M. Smith, P. F. Place, C. Harth, R. Beaudette, Q. Hua, B. Yang, I. Vimont, S. E. Michel, J. P. Severinghaus, D. Etheridge, T. Bromley, J. Schmitt, X. Faïn, R. F. Weiss and E. Dlugokencky, Nature, 2020, 578, 409–412 CrossRef CAS PubMed.
- L. Shen, R. Gautam, M. Omara, D. Zavala-Araiza, J. D. Maasakkers, T. R. Scarpelli, A. Lorente, D. Lyon, J. Sheng, D. J. Varon, H. Nesser, Z. Qu, X. Lu, M. P. Sulprizio, S. P. Hamburg and D. J. Jacob, Atmos. Chem. Phys., 2022, 22, 11203–11215 CrossRef CAS.
- OGCI, A report from the Oil and Gas Climate Initiative September 2018, https://www.ogci.com/wp-content/uploads/2018/09/OGCI_Report_2018.pdf, (accessed October 25, 2022).
- M. Saunois, A. R. Stavert, B. Poulter, P. Bousquet, J. G. Canadell, R. B. Jackson, P. A. Raymond, E. J. Dlugokencky, S. Houweling, P. K. Patra, P. Ciais, V. K. Arora, D. Bastviken, P. Bergamaschi, D. R. Blake, G. Brailsford, L. Bruhwiler, K. M. Carlson, M. Carrol, S. Castaldi, N. Chandra, C. Crevoisier, P. M. Crill, K. Covey, C. L. Curry, G. Etiope, C. Frankenberg, N. Gedney, M. I. Hegglin, L. Höglund-Isaksson, G. Hugelius, M. Ishizawa, A. Ito, G. Janssens-Maenhout, K. M. Jensen, F. Joos, T. Kleinen, P. B. Krummel, R. L. Langenfelds, G. G. Laruelle, L. Liu, T. Machida, S. Maksyutov, K. C. McDonald, J. McNorton, P. A. Miller, J. R. Melton, I. Morino, J. Müller, F. Murguia-Flores, V. Naik, Y. Niwa, S. Noce, S. O’Doherty, R. J. Parker, C. Peng, S. Peng, G. P. Peters, C. Prigent, R. Prinn, M. Ramonet, P. Regnier, W. J. Riley, J. A. Rosentreter, A. Segers, I. J. Simpson, H. Shi, S. J. Smith, L. P. Steele, B. F. Thornton, H. Tian, Y. Tohjima, F. N. Tubiello, A. Tsuruta, N. Viovy, A. Voulgarakis, T. S. Weber, M. van Weele, G. R. van der Werf, R. F. Weiss, D. Worthy, D. Wunch, Y. Yin, Y. Yoshida, W. Zhang, Z. Zhang, Y. Zhao, B. Zheng, Q. Zhu, Q. Zhu and Q. Zhuang, Earth Syst. Sci. Data, 2020, 12, 1561–1623 CrossRef.
- M. Cain, J. Lynch, M. R. Allen, J. S. Fuglestvedt, D. J. Frame and A. H. Macey, npj Clim. Atmos. Sci., 2019, 2, 29 CrossRef PubMed.
- J. Lynch, M. Cain, R. Pierrehumbert and M. Allen, Environ. Res. Lett., 2020, 15, 044023 CrossRef CAS PubMed.
- OGCI, Oil and Gas Climate Initiative. 2025 methane intensity target., https://www.ogci.com/action-and-engagement/reducing-methane-emissions/, (accessed October 25, 2022).
- GHGSat, GHGSat measures its largest emission from a single source ever from Nord Stream 2 leak, https://www.ghgsat.com/en/newsroom/ghgsat-nordstream/, (accessed October 24, 2022).
|
This journal is © The Royal Society of Chemistry 2023 |