DOI:
10.1039/D3DT02555A
(Paper)
Dalton Trans., 2023,
52, 16525-16537
Compositionally engineered Cd–Mo–Se alloyed QDs toward photocatalytic H2O2 production and Cr(VI) reduction with a detailed mechanism and influencing parameters†
Received
7th August 2023
, Accepted 9th October 2023
First published on 25th October 2023
Abstract
With the exceptional advantages of safety, greenness, and low cost, photocatalytic H2O2 generation has kindled a wonderful spark, although being severely hampered by the terrible photoinduced exciton recombination, migration, and surface decomposition. Here, employing reflux method, the Cd–Mo–Se quantum dots of varying molar ratios of Cd and Mo were synthesized using thioglycolic acid as the capping ligand to regulate their growth. This type of metal alloying promotes rapid charge migration, improves light harvesting, and reduces the rate of charge recombination. The improved optoelectronic properties and boosted activity of Cd-rich ternary CMSe-1 QDs led to the observed exceptional photocatalytic H2O2 yield of 1403.5 μmol g−1 h−1 (solar to chemical conversion efficiency, 0.27%) under visible light, outperforming the other ternary and Se-based QD photocatalysts. Additionally, CMSe-1 shows 93.6% (2 h) hazardous Cr(VI) photoreduction. The enhanced catalytic performance of CMSe-1 corresponds to effective charge carrier separation and transfer efficiency, well supported by PL, TRPL, and electrochemical measurements. Photocatalytic H2O2 production was also studied under varying experimental conditions and the scavenger test suggests a superoxide radical intermediate 2-step single electron reduction pathway. The catalyst-assisted Cr(VI) reduction is substantiated by the zero-order kinetics as well as the determination of the pHPZC value. The catalyst can be employed for a maximum of four times while retaining its activity, according to the photostability and reusability test outcomes. This research presents interesting approaches for producing ternary QDs and modified systems for efficient photocatalytic H2O2 production and Cr(VI) reduction.
Introduction
H2O2 has gained considerable attention for its use as a safe and green oxidant in chemical synthesis, papermaking, disinfection, and wastewater treatment.1,2 These days, H2O2 is also utilized as a fuel in cutting-edge one-compartment fuel cells, having output potentials on par with those of traditional hydrogen fuel cells.3 At present, anthraquinone oxidation (AQ) is the conventional technique for the commercial synthesis of H2O2; however, this approach leads to substantial noxious by-products, toxic solvent use, and extensive energy consumption.4,5 Additionally, the direct catalytic combination of H2 and O2 (such as Pd or Au/Pd) has explosion hazards, whereas the electrochemical method has selectivity issues and hence has not yet been used in industrial production.6 Considerable effort has been put into investigating the most efficient and environmentally viable technique to substitute the AQ method and meet the diversified demand.7,8 Photocatalytic H2O2 generation is currently viewed as a revolutionary option as far as the environment and sustainability are concerned.9 However, H2O2 is thermodynamically unstable and tends to dismutate backward into H2O and O2 which reduces the overall yield which is another challenge. Photocatalytic generation of H2O2 is frequently observed when oxygen is permitted to engage with the photocatalyst in the presence of hole-quenching elements (e.g., oxalic acids, alcohols, etc.). Typically, the holes (h+) in the valence band (VB) oxidize H2O/sacrificial agents to H+ and O2/oxidized product, while the electrons (e−) in the conduction band (CB) with the required potential take part in the O2 reduction reaction (ORR). The O2 reduction reaction proceeds via a single-step two-electron (O2 + 2H+ + 2e− → H2O2, 0.68 V vs. NHE) or stepwise single-electron (O2 + e− → ˙O2−, −0.33 V vs. NHE and ˙O2− + e−+2H+ → H2O2, 1.44 V vs. NHE) pathway, producing H2O2. Hence, it is crucial to pick a photocatalyst with the right band edge potentials, along with improved carrier separation and transfer efficiency to achieve an enhanced activity.10,11 In this regard, a wide range of photocatalysts including TiO2, g-C3N4, QDs, RGO-based systems, and metal–organic frameworks (MOFs) have been investigated but to touch the target value for the large-scale industrial production of H2O2 remains challenging.12–21
In addition to this, the release of toxic heavy metal ions, specifically Cr(VI), from industrial wastewater into surface water and groundwater presents a significant risk to both human health and aquatic ecosystem equilibrium.22 This is primarily due to the considerable solubility of Cr(VI) in water, as well as its substantial carcinogenic and acute toxic properties.23 However, Cr(III) has been proven to be less hazardous as compared to Cr(VI) due to its limited oxidation characteristics and bioavailability.24 Significant research interest has been directed towards the development of highly efficient photocatalysts for the reduction of Cr(VI) due to the eco-friendly, renewable, and sustainable nature of the photocatalytic process.25–28 Out of the many recently reported materials, QD-based photocatalytic systems are promising candidates. They show the maximum absorption range in the visible region because of their multiple electron production with a single photon and band gap tunability concerning particle size.29–31
Quantum dots (QDs) are a kind of zero-dimensional photocatalyst with a quantum confinement effect, a greater surface binding potential, tunable optoelectronic properties, and chemical stability that have been extensively explored over the past few years for various photocatalytic applications.32–35 In this context, the author group has made tremendous advancements and developed potent QDs toward water splitting, N2 fixation, and environmental pollutant remediation-related applications.36–39 According to several studies, visible light activates QDs in an aerobic environment and releases superoxide radicals (˙O2−), which are regarded as one of the crucial intermediates for the production of H2O2. Therefore, photocatalytic generation of H2O2 employing QDs as a photocatalyst under visible light conditions may be feasible.40 Moreover, the formation of superoxide radicals by QDs has been reported to give them exceptional selectivity for H2O2. However, the efficiency is still below the benchmark for standard applications. As a result, a variety of modification techniques, including doping, heterojunction formation, and defect engineering are frequently followed to increase the activity of photocatalytic H2O2 generation on QDs.41–43 As seminal studies, Xu et al. investigated the H2O2 production capacity of CdSe QDs that goes via the two-electron and one-electron molecular oxygen reduction process. The group then prepared K, P, N, co-doped hollow carbon polyhedron (KPN-HCP) modified CdSe QDs and reported a four-fold increase in H2O2 generation due to improved O2/H2O surface adsorption, effective electron–hole separation and proton release.44 In a recent study, Ji et al. investigated H2O2 production over CdSe-based core/shell QDs and improved their activity (126 mmol L−1/2 h) via wavefunction engineering, tunable band alignment, and surface–shell structure design.17 Alloyed QDs can be synthesized by making composites between binary QDs and other semiconducting materials. By choosing the appropriate alloy composition, the band gap alignment of alloyed QDs can be widely tuned and optimized, which is attributed to the optical bowing effect and their absorption can be extended into the visible region.45 Alloying Cd and Mo to form ternary Cd–Mo–Se QDs is an efficient way to enhance the photocatalytic efficiency as compared to binary CdSe and MoSe2 QDs by suppressing the exciton recombination rate. The band structure of CdxMo1−xSe QDs can be tuned according to the composition of Cd and Mo, which exhibits better performance in photocatalytic H2O2 production than CdSe.
In this study, alloyed CdxMo1−xSe (CMSe) QDs with a varying content of Cd and Mo along with binary CdSe and MoSe2 QDs were fabricated by the one-step reflux method using an appropriate amount of thioglycolic acid to prevent agglomeration of small sized QDs. The Cd-rich alloyed QDs (CMSe-1, where x = 0.7) show superior performance to the Mo-rich alloyed QDs (CMSe-2, where x = 0.3) with excellent reusability. The higher the Cd proportion, the wider the band gap in the alloyed QDs, which leads to a lower charge recombination rate and higher band potential, which is in good accordance with the data of PL, M–S plot, and EIS measurements. The prepared QDs were examined towards photocatalytic H2O2 production under different reaction conditions and it was found that the activity of CMSe-1 alloyed QDs (1403.5 μmol g−1 h−1) outperforms both the binary QDs. Furthermore, CMSe-1 QDs show noticeable efficiency (93.6% in 2 h) in photocatalytic Cr(VI) reduction at low pH. The mechanisms of both H2O2 production and Cr(VI) reduction have been studied in detail along with the involvement of active species, which is supported by scavenger tests. The presence of defects plays an important role in increased peroxide formation and Cr(VI) reduction. This investigation paves a new avenue in designing compositionally engineered alloyed QDs for achieving improved catalytic efficiency for various photocatalytic energy and environmental-related applications.
Experimental section
Materials
Cd(NO3)2·4H2O, Na2MoO4·2H2O, thioglycolic acid (TGA), NaBH4, KI, and ammonium molybdate were purchased from Merck, India. Selenium powder was purchased from Himedia. All chemicals were of analytical reagent grade, so used as such without any further purification. Throughout the reaction, distilled water was used as a solvent.
Synthesis of Cd–Mo–Se QDs
Binary and alloyed QDs were fabricated by a one pot method by following previously reported literature with slight modification.46 Briefly, NaHSe solutions as Se precursor were synthesized by dissolving Se powder with NaBH4 in chilled water. 159 mg (4 mmol) of NaBH4 was dissolved in 2 mL of chilled distilled water in a flask, followed by the addition of 158 mg (2 mmol) of Se powder. Immediately the flask was placed into an ice bath and H2 gas was bubbled for approximately 60 to 90 min. Finally, the black Se was reduced to transparent NaHSe, and white Na2B4O7 precipitated.47,48 | 4NaBH4 + 2Se + 7H2O ↔ 2NaHSe + Na2B4O7 + 14H2↑ | (1) |
For the typical synthesis of CMSe-1 QDs with 0.7
:
0.3 molar ratio of Cd
:
Mo, Cd(NO3)2·4H2O (436 mg), Na2MoO4·2H2O (147 mg) and TGA (0.5 mL) were dissolved in 200 mL deionized water in a three-neck round bottomed flask with continuous stirring. The pH was adjusted around 11 by 0.1 M NaOH under an N2 atmosphere to prevent oxidation of the material. The freshly synthesized NaHSe was quickly added into the solution and kept refluxing at 100 °C for 3 h. Furthermore, to synthesize QDs with different molar ratios of Cd
:
Mo (1
:
0, 0.7
:
0.3, 0.3
:
0.7, and 0
:
1), the compositions of the Cd and Mo precursors were accordingly changed. Scheme 1 depicts the stabilized CMSe alloyed QD in the presence of TGA under reflux conditions.
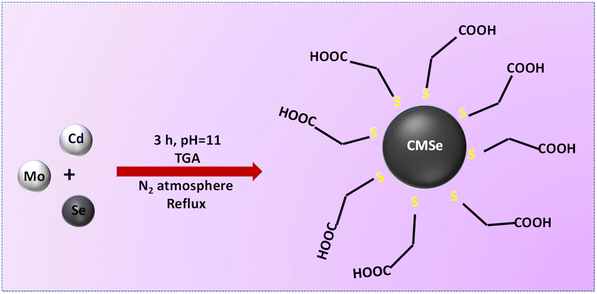 |
| Scheme 1 Schematically illustrating the synthesis procedure of CMSe alloyed QDs. | |
Photocatalytic H2O2 production
The photocatalytic oxygen reduction reactions were performed under 250 W visible light. 20 mg of catalyst was suspended in 20 mL of distilled water containing 5% (v/v) as a sacrificial electron donor. The mixture solution was sonicated for 15 min. Then the suspension was transferred into the reactor and was stirred in the dark with continuous bubbling of O2 to attain the adsorption–desorption equilibrium. After 30 min, the light was focused on the suspension solutions for 2 h to produce H2O2. Then to separate the photocatalyst, the suspension was centrifuged followed by filtration. Further to determine the amount of H2O2 produced, the iodometry method was adopted, where 2 mL of 0.1 M KI and 0.05 mL of 0.01 M ammonium molybdate were mixed with 1 mL of filtrate solution. In such a procedure, I− forms I3− after reacting with H2O2 molecules, and the concentration of I3− is measured using a spectrophotometer to determine how much H2O2 was produced. Additionally, a blank experiment carried out without the use of a photocatalyst or light and confirming that no H2O2 was formed at any concentration confirms the effectiveness of the photocatalyst in the O2 reduction reaction. UV-vis DRS (λ = 350 nm) was applied to detect the concentration of H2O2 produced.
Additionally, the process of decomposing H2O2 was conducted in a 30 mL reactor made of quartz, while being exposed to light. In a standard experimental procedure, a reactor was filled with 15 mL of hydrogen peroxide solution (16 mM) and subsequently subjected to cooling through an ice bath. 20 mg of catalyst was introduced into the reactor and subjected to light exposure under continuous agitation. Following the reaction, the solution was centrifuged and subsequent analysis of H2O2 was conducted.
Photocatalytic Cr(VI) reduction
CMSe-1 QDs were also used to minimize hazardous Cr(VI) to hypoxic Cr(III) owing to their wide-range light absorption capability and photostability causing serious ecological pollution. From the results of photocatalytic H2O2 generation, the highest activity is recorded for CMSe-1 QDs. Therefore, the photocatalytic Cr(VI) reduction was studied using a CMSe-1 catalyst. In the performed experiment, 20 mg of CMSe-1 QDs and 20 mL of 10 ppm Cr(VI) solution were mixed in a beaker. This solution was continuously stirred in the dark for 30 min to attain equilibrium between adsorption and desorption. Subsequently, a 20 W LED was used to illuminate the mixture solution. The photocatalytic reduction of Cr(VI) over CMSe-1 QDs was observed for different times such as 30 min, 60 min, 90 min, and 120 min. The photocatalytic reduction activity of CMSe-1 was also investigated at different pH (3, 6, 9, 12) where the pH was maintained using 1 M HCl and 1 M NaOH. After the reaction, the solution was centrifuged and the supernatant was collected. To prove the photoreduction of Cr(VI) to Cr(III) and quantify the amount of (VI), the colorimetric test was performed, where 2 mL of sulphuric acid (H2SO4, 3 M) and 1 mL of freshly prepared 1,5-diphenylcarbonohydrazide (DPC) were added to 2 mL of the supernatant. After allowing it to stand in the dark for 15 min, the absorbance of the solution was measured at 540 nm using a UV-vis spectrophotometer.
Reusability test of CMSe QDs
After photocatalytic reduction, CMSe-1 QDs were recovered by centrifugation and washed several times with ethanol–water solution, and dried under vacuum. Then the QD was directly applied to the next cycles of H2O2 generation and Cr(VI) photoreduction.
Results and discussion
Structure and morphology
The powder X-ray diffractometry (XRD) pattern of the synthesized samples (CSe, MSe, CMSe-1, and CMSe-2 QDs with molar ratios 1
:
0, 0
:
1, 0.7
:
0.3, and 0.3
:
0.7 of Cd
:
Mo56–58 respectively) is depicted in Fig. 1a. The diffraction peaks obtained from XRD of CMSe-1 and CMSe-2 QDs show peaks of both CdSe and MoSe2 QDs, supporting the formation of an alloyed structure.49 However, the intensity of the peak depends upon the ratio of Cd
:
Mo. The peaks of CMSe-1 and CMSe-2 appeared at around 2θ = 25° (111), 42° (220), and 49° (311) with slight shifting (enlarged view depicted in ESI (S1)†) and can be indexed to the characteristic peaks of CdSe with zinc blende structure, which are well matched with previous data (JCPDS Card No. 19-0191). Similarly, the signature peak at 32° corresponds to the characteristic (100) plane of MoSe2 having a rhombohedral structure (JCPDS Card No. 20-0757). It can be seen that evenly dispersed CMSe-1 are tiny dot-like nanoparticles, visualized from TEM images presented in Fig. 1b and c. An average particle size of Σ4 nm was determined from the particle size distribution plot (inset ESI (S2)†). Due to the tendency of QDs to aggregate, the diameter of the CMSe-1 expands. In parallel, Fig. 1d is the high-resolution TEM (HRTEM) image of CMSe-1 that displays a clear crystalline lattice spacing i.e., d = 0.352 nm, which is assigned to the (111) crystal plane and is in good accordance with XRD of CMSe-1. Also, the disordered and unclear areas confined by the yellow circle indicate the presence of defects. Here, in comparison to the lattice fringes of neat CdSe (dspacing = 0.337 nm for the (111) plane), the alloyed CMSe-1 shows a high d spacing value of 0.352 nm for the (111) plane, which indicates lattice expansion and formation of defect or disorder within the system.50 The polycrystalline structure of CMSe-1 QD can be witnessed from the continuous Deby–Scherrer rings in the selected area electron diffraction (SAED) pattern in Fig. 1e.
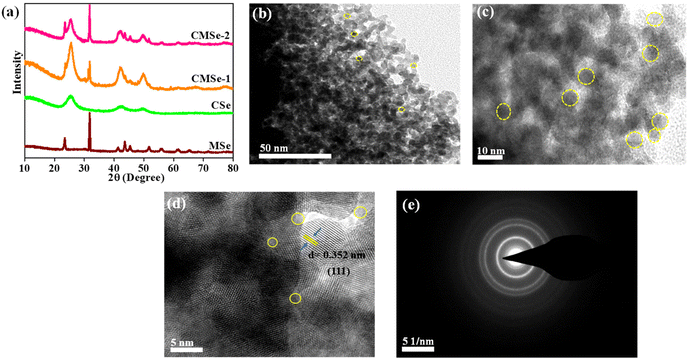 |
| Fig. 1 (a) Powder XRD pattern of CSe, MSe, CMSe-1, and CMSe-2, (b and c) HRTEM images (d) HRTEM fringe pattern (e) SAED pattern of CMSe-1 QD. | |
Furthermore, XPS analysis was carried out to illustrate the surface chemical state and interfacial electronic states of CMSe-1 QD. Fig. 2a displays Cd 3d spectra, which are deconvoluted into two peaks positioned at binding energies (BEs) of 404.8 and 411.6 eV and are attributed to the Cd 3d5/2 and Cd 3d3/2 spin state of Cd(II) respectively.46,51 The high-resolution deconvoluted Mo 3d spectrum also exhibits two peaks at BEs of 228.6 and 231.6 eV, which are assigned to Mo 3d5/2 and Mo 3d3/2 energy levels respectively as shown in Fig. 2b, indicating the +4 oxidation state of Mo.52 Besides, the peaks at BE of 53.6 (Se 3d5/2) and 54.6 eV (Se 3d3/2) in Fig. 2c present the (II) oxidation state of Se in the alloyed QD.53 Moreover, ESI (S3)† presents the survey analysis spectra of CMSe-1 QDs, from which it can be noticed that Cd, Mo, and Se elements are detected, revealing the coexistence of these three elements without any impurity species. In parallel, all the compositional constituents present in CMSe-1 QDs were evaluated from the energy-dispersive X-ray (EDX) (Fig. 2d) study, which is in good accordance with XPS analysis spectra. The color mapping image of CMSe-1 QDs is also included in ESI (S4)† which supports the above observation of elemental content. Additionally, the plotted EPR result in ESI (S5)† shows that the sample CMSe-1 contains a defect with an anisotropic feature brought by super hyperfine splitting.54 The existence of defects in CMSe-1 systems is also well supported by the HRTEM image (Fig. 1d), crucial for the activation and adsorption of molecular oxygen, which increases the formation of H2O2.
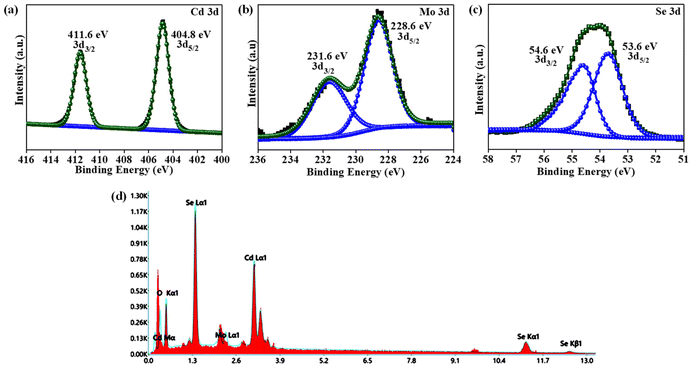 |
| Fig. 2 XPS spectra of (a) Cd, (b) Mo, (c) Se elements, and (d) EDX analysis demonstrating elemental components in CMSe-1. | |
Optical and electrochemical properties
The optical absorption properties of CSe, Mse, CMSe-1, and CMSe-2 QDs are also investigated by UV-vis spectroscopy. As depicted in Fig. 3a, the CMSe-1 and CMSe-2 alloyed QDs exhibit good light absorption with their absorption edges in between that of the binary QDs suggesting alloy formation.55 Moreover, the activity of photocatalysts largely depends on the separation efficiency of electron–hole pairs, which can be estimated by photoluminescence (PL) spectra. Fig. 3b shows the PL spectra of Cse, Mse, CMSe-1, and CMSe-2 QDs photoexcitation at 380 nm, where Mse QDs exhibit the strongest PL intensity due to the highest extent of photogenerated charge carrier recombination. However, the PL intensities of the CMSe-1 and CMSe-2 QDs are notably reduced, indicating that alloying of metals hinders the recombination of charge carriers, and the lowest intensity is seen in CMSe-1 QDs, which is due to the efficient quenching of electron holes. The asymmetric peaks on the PL graph could be caused by band gap transitions and the existence of defects, implying that CMSe-1 QDs like other QDs should have a wide particle size distribution.36 Additionally, the separation efficiency of electron–hole pairs is also supported by electrochemical impedance spectroscopy (EIS) as illustrated in Fig. 3c. Compared to Cse and Mse QDs, the semi-circular diameter of CMSe-1 and CMSe-2 QDs indicates better charge flow although the smallest arc radius is observed for CMSe-1 QD implying the lowest migration resistance for photo-excited charge carriers. Moreover, Fig. 3d represents the transient photocurrent plot of CSe, MSe, CMSe-1, and CMSe-2 QDs under the light on/off conditions. This implies that the highest transfer efficiency of photogenerated charge carriers is for CMSe-1 QDs, which leads to enhanced photocatalytic activity. Adding more, the small spikes in the photocurrent plot of binary QDs upon interrupted light irradiation indicate the faster recombination of holes and electrons. Additionally, Fig. 3e represents the linear sweep voltammetry (LSV) analysis of the synthesized binary and alloyed QDs with the potential bias range from −0.8 to 0.0 V. All of them exhibit cathodic photocurrent densities and the highest negative photocurrent density (−0.868 mA cm−2) is determined from CMSe-1 QDs, resulting in the most accelerated photogenerated charge carrier separation and hence better activity.
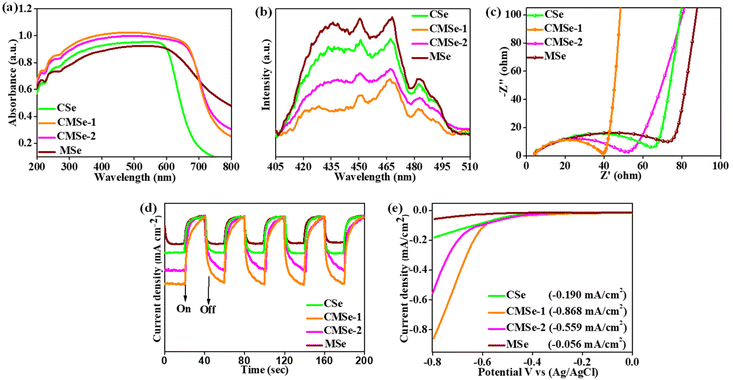 |
| Fig. 3 Analysis of optoelectronic properties of CSe, MSe, CMSe-1, and CMSe-2 by (a) UV-vis DRS absorbance spectra, (b) PL spectra, (c) EIS spectra, (d) transient photocurrent response, (e) linear sweep voltammetry plot. | |
Based on the Bode phase plot of CSe, MSe, CMSe-1, and CMSe-2 QDs, displayed in Fig. 4a, it can be stated that the frequency regions can be used to quickly identify the lifespan of the injected electron. Applying eqn (1), the lifespan of the injected electrons was calculated.
| The life span of electrons (τ) = 1/2Πƒmax | (1) |
where
τ and ƒ
max correspond to the lifetime of photoinjected electrons and the minimum reverse frequency of the photocatalyst. The lifetime of these electrons was somehow correlated with the shift in the peak position along the frequency region
i.e., drift to the lower or higher position. In
Fig. 4a, CMSe-1 QDs have the lowest frequency value (ƒ
max) and longest duration (
τ = 53.6 μs) of electron lifespan, resulting in enhanced photocatalytic activity. The photoinjected electron lifespan calculated for CMSe-2, CSe, and MSe are 41.5, 37.5, and 37.4 μs respectively. The substantially longer injected exciton lifespan of CMSe-1 QDs indicates their fast interfacial charge carrier transfer dynamics. Similarly, the time-resolved photoluminescence spectroscopy (TRPL) of the as-prepared CMSe-1 and CMSe-2 QDs was conducted to evaluate the lifespan of photogenerated charge carriers, which is shown in
Fig. 4b. A bi-exponential model as specified in
eqn (2) was used to fit the acquired decay curves.
| Fit = A + α1 exp(−t1/τ1) + α2 exp(−t2/τ2) | (2) |
where
A is a constant and relative contributions are symbolised by
α1 and
α2. The time after a pulsed laser excitation is represented by
t. In this context,
τ1 and
τ2 denote the decay lifetimes, respectively, which elucidate the processes of radiative recombination and nonradiative relaxation of photo-excitons. Using
eqn (3) and the parameters listed in Table S1 (ESI
†), the average lifetime (
τavg) was calculated.
| 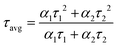 | (3) |
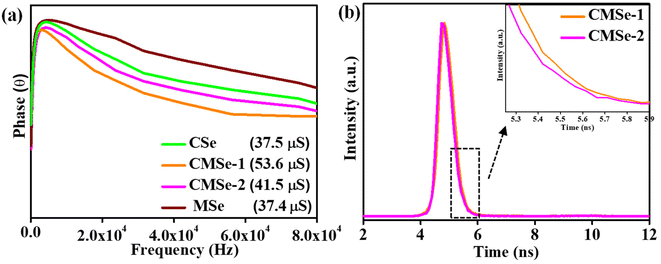 |
| Fig. 4 (a) Bode phase plot of CSe, MSe, CMSe-1, and CMSe-2 (b) TRPL plot of CMSe-1 and CMSe-2. | |
The CMSe-1 exhibits an average lifetime of 1.53 nanoseconds, surpassing the average lifetime of CMSe-2 (1.51 nanoseconds). This discrepancy suggests that the increased concentration of Cd in CMSe-1 contributes to enhanced exciton dissociation and slow decay of charge carriers on the surface, thereby favourably impacting the photocatalytic activity.
Adding more to the investigation, the Kubelka–Munk function was used to estimate the band gap energy (Eg) of CMSe-1 and CMSe-2 QDs and was found to be 1.76 and 1.70 eV respectively, as illustrated in Fig. 5a. Additionally, the resulting Urbach energies of CMSe-1 and CMSe-2 are determined to be 0.26 eV and 0.19 eV as given in ESI (S6).† Increased disorder or defects in the system are indicated by the high Urbach value of the alloyed CMSe-1 QD. The system becomes more disorderedly, which may be attributed to lattice expansion and, consequently, an increase in Cd concentration may affect the band edge potentials by causing a variation in the band gap energy. Lattice expansion or absence of continuous lattice fringes is related to defect presence. Also, this defect formation is well supported by HRTEM and EPR analysis.56–58
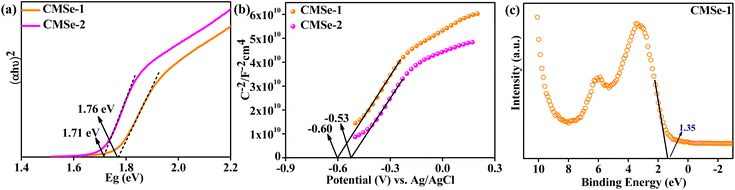 |
| Fig. 5 (a) Tauc plot for the determination of band gap, (b) M–S plot to obtain conduction band potential of CMSe-1 and CMSe-2 QD, (c) XPS VB plot of CMSe-1. | |
Determining the valence band (VB) and conduction band (CB) positions of CMSe-1 and CMSe-2 QDs helps to better explain the mechanism of photocatalytic H2O2 production. The flat band potential of CMSe-1 and CMSe-2 QDs were obtained from the Mott–Schottky plots and estimated as −0.60, and −0.53 V (vs. Ag/AgCl at pH 7) respectively as shown in Fig. 5b. The positive slopes of the M–S plot evidence CMSe-1 QDs as an n-type semiconductor. The CB minima of CMSe-1 and CMSe-2 are then recalculated by using eqn (4) to be −0.41 and −0.34 eV (vs. NHE at pH 7), respectively and their respective VB band positions are 1.35 and 1.37 eV based on eqn (5). Because the CB maximum of CMSe-1 QDs (−0.41 eV, vs. NHE) is higher than that of O2/˙O2−(−0.046 V vs. NHE), a substantial amount of ˙O2− is generated on the CMSe-1 surface which then reacts with H+ generated by the oxidation process (used sacrificial agent) resulting in more H2O2 generation.
|  | (4) |
where
EAg/AgCl represents the flat band potential of materials, and the pH of the electrolyte was found to be 6.8, and the reference electrode constant
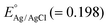
. Likewise, the VB potential of CMSe-1 (1.35 eV) can be evaluated by XPS VB spectroscopy as displayed in
Fig. 5c. The primary shoulder pattern of the first peak at the ends with the least binding energy correlates to the VB potential of CMSe-1 in the XPS VB spectra.
Applications
Photocatalytic H2O2 generation
The photocatalytic performances of the synthesized QDs were examined towards H2O2 generation under visible light irradiation. The concentration of H2O2 produced by bare CdSe and MoSe2 QDs are 408.15 μmol g−1 h−1 and 212.76 μmol g−1 h−1 respectively. It is notable from Fig. 6a, that CMSe-1 QD reveals the highest H2O2 production of 1403.5 μmol g−1 h−1 which is 3.4 and 6.6 times higher than those of binary CdSe and MoSe2 respectively due to the suppressed exciton recombination rate. CMSe-1 QDs with a Cd/Mo ratio of 0.7/0.3 outperforms CMSe-2 QDs (963 μmol g−1 h−1) including binary QD (CdSe and MoSe2). The Cd-rich catalyst (CMSe-1) displays better photocatalytic efficiency than the Mo-rich alloyed QDs (CMSe-2) because the higher proportion of Cd widens the bandgap of CMSe-1 QDs as compared to that of the CMSe-2 QDs. Hence, the electron–hole recombination rate decreases and leads to better activity. Besides, the solar to chemical conversion efficiency (SSC) using CMSe-1 was determined to be 0.27% at λmax ≥ 420 nm using eqn (S1) (detailed formulation in the ESI†). Simultaneously, the decomposition of H2O2 becomes a critical issue as it reduces the overall H2O2 yield including material loss. Furthermore, from Fig. 6b it can be visualized that by using CMSe-1 as a photocatalyst, the rate of H2O2 generation in an O2-saturated solution is dramatically high, whereas in an N2 or Ar environment, very negligible H2O2 was discovered revealing that O2 plays a major role in photocatalytic H2O2 generation and also confirms the photoreduction of the supplied O2 to H2O2. Additionally, the active species trapping experiments were performed to figure out the reaction pathways of photocatalytic H2O2 production using EDTA, dimethyl sulphoxide (DMSO), isopropanol (IPA), and p-benzoquinone (BQ) as the scavengers of holes (h+), electrons (e−), hydroxyl radical (OH˙), and superoxide radical (˙O2−) respectively. In the absence of a certain sacrificial agent, CMSe-1 demonstrated the best photocatalytic H2O2 performance e.g., when EDTA is utilized as the sacrificial agent, the yield of H2O2 increases dramatically because it captures holes, allowing more electrons for O2 reduction. In contrast, employing DMSO, H2O2 production reduces significantly, implying the important role of electrons in the O2 reduction reaction. ˙OH and ˙O2− are trapped by adding IPA and p-BQ, respectively. H2O2 yield is reduced in the presence of p-BQ, but greatly boosted upon the addition of IPA as it is a sacrificial agent. This shows that ˙O2− rather than ˙OH is the active species during H2O2 production. Notably, when p-BQ or DMSO is added to the solution, there is essentially no H2O2 generation indicating that the oxygen reduction reaction is the primary source of H2O2 production, which can be confirmed from Fig. 6c. Based on the above observation, it can be projected that O2 reduction goes predominantly by the superoxide radical mediated single electron two-step route. Further, the superoxide radical generation ability of CMSe-1 QDs is confirmed through the NBT test following our previously reported literature. In this reduction process, alcohol as a sacrificial agent not only oxidizes the holes but also releases H+ which further interacts with the superoxide intermediate producing H2O2. Besides, defect presence in CMSe-1 not only retards the recombination process but encourages O2 adsorption and activation by lowering the Gibbs free energy of O2 adsorption (rate-determining stage) promoting hydroperoxy intermediate (*OOH) generation which then transfers to H2O2. A similar type of observation was also made by Zou et al. and Li et al.59,60 Furthermore, the photoproduction of H2O2 under different pH conditions is evaluated and displayed in Fig. 6d. It is observed that at pH 6, the synthesized photocatalyst exhibits the highest catalytic activity. At a lower pH of 3, the catalytic efficiency slightly decreases which might be due to the occurrence of the competitive H2 evolution reaction. Thereafter, an alkaline medium also hinders the formation of H2O2. The reusability of catalyst is an important parameter in deciding its efficacy and practicability. In the present case, four successive cycles of testing were performed on the photocatalytic stability of CMSe-1 QDs. Upon exposure to light for the first three cycles, the ability to generate H2O2 is remarkably stable; however, by the fourth cycle, the H2O2 concentration had slightly dropped as illustrated in Fig. 6e. The loss of catalyst quantity during recovery and catalyst aggregation due to continued usage is blamed for the reported modest drop in activity during reusability. The remarkable stability is closely related to the supplied XRD of CMSe-1 QD which is nearly identical before and after usage, as shown in ESI (S7).†
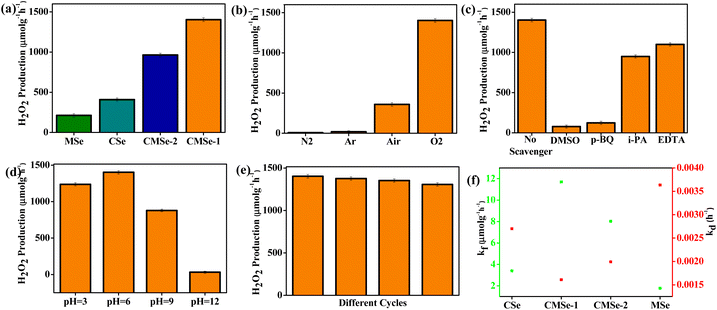 |
| Fig. 6 (a) Yield of photocatalytic H2O2 generation using different photocatalysts under 250 W visible light irradiation for 2 h, (b) H2O2 production rate under different saturated atmospheres (c) Influence on H2O2 generation rate in the presence of different scavengers, (d) The pH effect on the photocatalytic H2O2, and (e) reusability plot of CMSe-1, (f) kf and kd for H2O2 generation rate of photocatalysts (CSe, MSe, CMSe-1, and CMSe-2). | |
Meanwhile, the decomposition of H2O2 occurs simultaneously, and this is a competitive reaction involving electrons and holes. The decomposition rate of H2O2 with an initial concentration of 1000 μM under light illumination was studied and is shown in ESI (S8).† To analyze the kinetics, the applied eqn (6) and (7) are given below:
| 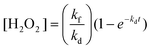 | (6) |
where
kf and
kd represent the formation and decomposition rate constant of H
2O
2, respectively, which combinedly estimate the final H
2O
2 yield. Zeroth and first-order kinetics are applied to determine the value of
kf and
kd respectively. From
Fig. 6f, it is concluded that CMSe-1 having the highest
kf and lowest
kd displays the highest production of H
2O
2 among other samples. The aforementioned observation suggests that enhanced light-harvesting capacity and facilitated charge mobility of CMSe-1 are advantageous for both the photocatalytic formation as well as degradation of H
2O
2. Conversely, the rate of formation of CMSe-1 increases more than the rate of decomposition does, resulting in the overall remarkable rate of H
2O
2 production.
Photocatalytic Cr(VI) reduction
Cr(VI) is grouped under the toxic heavy metal category having carcinogenic effects on bio-diversity; therefore, its elimination or transfer to less toxic Cr(III) form is the most encouraging approach. In this regard, the efficiency of CMSe-1 QDs towards photocatalytic Cr(VI) reduction was carefully examined. However, Cr(VI) does not undergo self-degradation in the presence of light or catalyst only. As depicted in Fig. 7a, 93.6% of Cr(VI) was reduced by CMSe-1 QD within 2 h because of the fastest transfer and separation of charge carriers. Furthermore, a pseudo-first-order kinetic model of CMSe-1 QDs was comprehensively analysed for 2 h showing the rate constant (k) value of 0.0498 for CMSe-1 as shown in Fig. 7b. Moreover, pH is a key factor in Cr(VI) reduction because protons are more readily available at lower pH levels, and the surface of the photocatalyst becomes positively charged and attracts Cr2O72− anions, which speeds up the adsorption process. The redox reaction took place in the solution at higher pH levels, preventing Cr(VI) reduction. In this regard, Fig. 7c illustrates the reduction percentage of Cr(VI) by CMSe-1 at different pH values. The optimum catalytic activity in the photoreduction of Cr(VI) to Cr(III) is observed in acidic pH values (pH = 3) due to the active interaction of Cr anions with cations on the surface of the photocatalysts. Furthermore, the point of zero charges (PZCs) of the CMSe-1 sample was calculated following the drift method as previously reported by our group.29 The pHPZC value of CMSe-1 QDs is found to be 6.5 from the plot shown in ESI (S9).† Generally, the catalyst surface remains positively charged when the pH of the solution is lower than pHPZC, whereas negatively charged when the pH is higher than pHPZC.29 Due to acidic pH, Cr anions exist in the Cr2O72− form and as a catalyst with positive charge which favors effective interaction and successful reduction to hypoxic Cr(III). Moreover, a reusability plot of CMSe-1 QDs towards photocatalytic reduction of Cr(VI) is depicted in Fig. 7d which suggests that the catalyst preserved its activity even up to four continuous cycles without any marginal change in performance. Additionally, a comparison table for H2O2 production and Cr(VI) reduction of several photocatalysts is presented in Table S2,† which shows the superior activity of CMSe-1 QDs as a single photocatalyst.
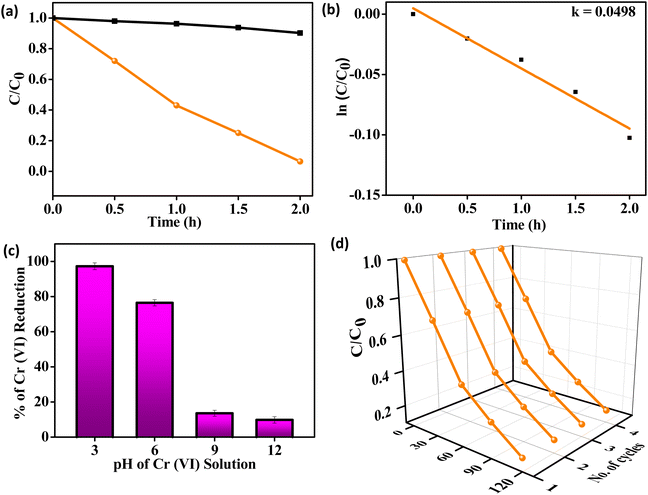 |
| Fig. 7 (a) Photocatalytic Cr(VI) reduction by CMSe-1 under visible light irradiation at various intervals of time, (b) kinetics of first-order mechanism of CMSe-1, (c) comparison of photocatalytic Cr(VI) reduction by CMSe-1 at different pH, (d) reusability test of CMSe-1. | |
Mechanistic approach
The preceding experimental work points to the plausible mechanism depicted in Scheme 2 to have a thorough grasp of photocatalytic H2O2 production and Cr(VI) reduction over the CMSe-1 photocatalyst. In this paper, the band structures of CMSe-1 and CMSe-2 are determined from UV-vis DRS and Mott–Schottky (MS) analysis, and VB-XPS. As the catalyst is exposed to visible light, electrons and holes are produced in the CB and VB respectively. The photogenerated holes in the VB of the CMSe-1 cannot oxidize water because the VB potential of CMSe-1 QD (+1.35 eV) is substantially lower than the necessary potential for water oxidation (2.68 eV).61 Additionally, H2O2 is generated in trials run neither in the dark nor without photocatalysts; hence the O2 reduction to H2O2 by CMSe-1 is likely fuelled by the photocatalytic pathway as represented in the eqn (8)–(12). The dissolved O2 can be reduced directly by a couple of electrons to generate H2O2 (eqn (10)) or by an indirect stepwise route via ˙O2− intermediate, which subsequently reacts with protons and electrons to produce H2O2 (eqn (11) & (12)). As the necessary condition, the CB of CMSe-1 (−0.41 eV) has enough potential of forming superoxide radicals (−0.046 V vs. NHE), further confirmed by NBT analysis (ESI (S10)†). Similarly, the holes in VB of CMSe-1 (+1.35 eV), oxidise the alcohol producing H+. Previously, from radical scavenger experiments, we confirmed that ˙O2− and e− play an integral part in the formation of H2O2via direct and indirect oxygen reduction channels. Additionally, confirmation regarding the formation of ˙O2− radicals was further obtained by EPR analysis, using 5,5-dimethyl-1-pyrroline N-oxide (DMPO) as the spin-trapping reagent. ESI (S11)† shows the peaks of DMPO-˙O2− under light irradiation. Below mentioned equations reflect the overall steps of H2O2 generation. | CMSe-1 + hν → CMSe-1(e− + h+) | (8) |
| CH3CH2OH + 2h+ → CH3CHO + 2H+ | (9) |
| O2 + 2e− + 2H+ → H2O2 | (10) |
| O2˙− + e− + 2H+ → H2O2 | (12) |
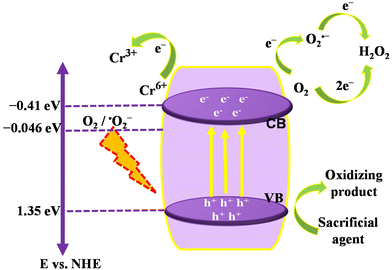 |
| Scheme 2 Plausible mechanism showing separation and migration of photogenerated charge carriers over CMSe-1 QDs for photocatalytic H2O2 production and Cr(VI) reduction. | |
Additionally, during the photoreduction process of Cr(VI), e− and h+ are generated the same way as discussed above. The photogenerated electrons are utilized for the reduction of Cr(VI) and some electrons are engaged in the production of superoxide radicals, which indirectly reduce Cr(VI) to Cr(III).25Eqn (13) represents the photoreduction of Cr(VI) to Cr(III):
| Cr2O72− + 14H+ + 6e− → 2Cr3+ + 7H2O | (13) |
Conclusion
In summary, it is simple to develop Cd–Mo–Se QDs using the bottom-up reflux approach from their respective precursors at a basic pH under an inert atmosphere with varying Cd and Mo concentrations. TGA is employed as a capping ligand which prevents agglomeration and promotes stabilizing growth. Cd–Mo–Se QDs by significant alloying of Cd and Mo along with defect presence are responsible for this enhancement in their activity. With improved absorption capacity and a lower charge recombination rate, Cd-rich CMSe-1 QDs produce H2O2 (1403.5 μmol g−1 h−1) at a rate that is significantly higher than that of Mo-rich CMSe-2 QD and binary QDs (CdSe and MoSe2), which is well correlated with optical and electrochemical analysis. The CMSe QDs respond to the production of H2O2 in the visible light range where the solar-to-chemical conversion efficiency over CMSe-1 was determined to be 0.27% at λ ≥ 420 nm. The scavenger test clarifies the superoxide-mediated O2 reduction route and the efficiency of Cr(VI) reduction over CMSe-1 is reported to be 93.6% in 2 h. Both the reduction of oxygen and the reduction of Cr(VI) follow zero-order kinetics. However, it is discovered that electrons are the primary pertinent active species for H2O2 generation and Cr(VI) reduction. CMSe-1 shows noticeable photocatalytic efficiency; however, to further strengthen the performance of the designed material, some modifications can be considered such as cation or anion alloying to prepare quaternary and quinary QDs. Moreover, the recombination rate of excitons can be further lowered by doping and forming heterojunctions of CMSe-1 with other suitable catalysts. These discoveries are crucial for the development of QD photocatalysts in the context of energy conversion and environmental remediation.
Conflicts of interest
The authors declare no competing financial interest.
Acknowledgements
The authors express their profound gratitude toward Siksha ‘O’ Anusandhan Deemed to be University for giving all the necessary facilities and financial support to carry out this immense research work.
References
- T. M. Gill, L. Vallez and X. Zheng, The role of bicarbonate-based electrolytes in H2O2 production through two-electron water oxidation, ACS Energy Lett., 2021, 6, 2854–2862 CrossRef CAS.
- Y. J. Lin, I. Khan, S. Saha, C. C. Wu, S. R. Barman, F. C. Kao and Z. H. Lin, Thermocatalytic hydrogen peroxide generation and environmental disinfection by Bi2Te3 nanoplates, Nat. Commun., 2021, 12, 180 CrossRef CAS PubMed.
- S. A. M. Shaegh, N. T. Nguyen, S. M. M. Ehteshami and S. H. Chan, A membraneless hydrogen peroxide fuel cell using Prussian Blue as cathode material, Energy Environ. Sci., 2012, 5, 8225–8228 RSC.
- H. Hou, X. Zeng and X. Zhang, Production of hydrogen peroxide by photocatalytic processes, Angew. Chem., Int. Ed., 2020, 59, 17356–17376 CrossRef CAS PubMed.
- X. Shen, Z. Wang, H. Guo, Z. Lei, Z. Liu and L. Wang, Solvent Engineering of Oxygen–Enriched Carbon Dots for Efficient Electrochemical Hydrogen Peroxide Production, Small, 2023, 2303156 CrossRef PubMed.
- J. M. Campos-Martin, G. Blanco-Brieva and J. L. Fierro, Hydrogen peroxide synthesis: an outlook beyond the anthraquinone process, Angew. Chem., Int. Ed., 2006, 45, 6962–6984 CrossRef CAS PubMed.
- W. Fan, Z. Duan, W. Liu, R. Mehmood, J. Qu, Y. Cao, X. Guo, J. Zhong and F. Zhang, Rational design of heterogenized molecular phthalocyanine hybrid single-atom electrocatalyst towards two-electron oxygen reduction, Nat. Commun., 2023, 14, 1426 CrossRef CAS PubMed.
- Z. Wang, G. Li, W. Hou, H. Guo, L. Wang and M. Wu, Insights into the Use of Te–O Pairs as Active Centers of Carbon Nanosheets for Efficient Electrochemical Oxygen Reduction, ACS Nano, 2023, 17, 8671–8679 CrossRef CAS PubMed.
- B. He, Z. Wang, P. Xiao, T. Chen, J. Yu and L. Zhang, Cooperative Coupling of H2O2 Production and Organic Synthesis over a Floatable Polystyrene–Sphere–Supported TiO2/Bi2O3 S–Scheme Photocatalyst, Adv. Mater., 2022, 34, 2203225 CrossRef CAS PubMed.
- B. Liu, C. Bie, Y. Zhang, L. Wang, Y. Li and J. Yu, Hierarchically porous ZnO/g-C3N4 S-scheme heterojunction photocatalyst for efficient H2O2 production, Langmuir, 2021, 37, 14114–14124 CrossRef CAS PubMed.
- Y. Qian, F. Zhang and H. Pang, A Review of MOFs and Their Composites-based Photocatalysts: Synthesis and Applications, Adv. Funct. Mater., 2021, 31, 2104231 CrossRef CAS.
- Y. Yang, B. Cheng and J. Yu, TiO2/In2S3 S-scheme photocatalyst with enhanced H2O2-production activity, Nano Res., 2023, 16, 4506–4514 CrossRef CAS.
- A. Behera, P. Babu and K. Parida, Growth of macroporous TiO2 on B-doped gC3N4 nanosheets: a Z-scheme photocatalyst for H2O2 production and phenol oxidation under visible light, Inorg. Chem. Front., 2021, 8, 1489–1499 RSC.
- B. P. Mishra, L. Biswal, S. Das, L. Acharya and K. Parida, Architecture and Kinetic Studies of Photocatalytic H2O2 Generation and H2 Evolution through Regulation of Spatial Charge Transfer via Z-Scheme Path over a (001) Facet Engineered TiO2@ MXene/B-g-C3N4 Ternary Hybrid, Langmuir, 2023, 39, 957–971 CrossRef CAS PubMed.
- C. Feng, L. Tang, Y. Deng, J. Wang, J. Luo, Y. Liu, X. Ouyang, H. Yang, J. Yuand and J. Wang, Synthesis of leaf–vein–like g–C3N4 with tunable band structures and charge transfer properties for selective photocatalytic H2O2 evolution, Adv. Funct. Mater., 2020, 30, 2001922 CrossRef CAS.
- J. H. Lee, H. Cho, S. O. Park, J. M. Hwang, Y. Hong, P. Sharma, W. C. Jeon, Y. Cho, C. Yang, S. K. Kwak and H. R. Moon, High performance H2O2 production achieved by sulfur-doped carbon on CdS photocatalyst via inhibiting reverse H2O2 decomposition, Appl. Catal., B, 2021, 284, 119690 CrossRef CAS.
- W. Ji, Z. Xu, S. Zhang, Y. Li, Z. Bao, Z. Zhao, L. Xie, X. Zhong, Z. Wei and J. Wang, High-efficiency visible-light photocatalytic H2O2 production using CdSe-based core/shell quantum dots, Catal. Sci. Technol., 2022, 12, 2865–2871 RSC.
- D. Prusty, S. Mansingh, N. Priyadarshini and K. M. Parida, Defect Control via Compositional Engineering of Zn-Cu-In-S Alloyed QDs for Photocatalytic H2O2 Generation and Micropollutant Degradation: Affecting Parameters, Kinetics, and Insightful Mechanism, Inorg. Chem., 2022, 61, 18934–18949 CrossRef CAS PubMed.
- K. K. Das, S. Mansingh, R. Mohanty, D. P. Sahoo, N. Priyadarshini and K. Parida, 0D–2D Fe2O3/Boron-Doped g-C3N4 S-Scheme Exciton Engineering for Photocatalytic H2O2 Production and Photo-Fenton Recalcitrant-Pollutant Detoxification: Kinetics, Influencing Factors, and Mechanism, J. Phys. Chem. C, 2022, 127, 22–40 CrossRef.
- S. Mansingh, D. P. Sahoo, L. Paramanik, M. Sahoo and K. Parida, Robust charge carrier engineering via plasmonic effect and conjugated Π-framework on Au loaded ZnCr-LDH/RGO photocatalyst towards H2 and H2O2 production, Inorg. Chem. Front., 2022, 9, 559–576 RSC.
- Y. Isaka, Y. Kawase, Y. Kuwahara, K. Mori and H. Yamashita, Two–phase system utilizing hydrophobic metal–organic frameworks (MOFs) for photocatalytic synthesis of hydrogen peroxide, Angew. Chem., 2019, 131, 5456–5460 CrossRef.
- S. K. Pradhan, K. Das, R. Bariki, D. Majhi, N. Behera and B. G. Mishra, Facile low temperature reflux synthesis of Bi self-doped Bi2MoO6 and construction of CaFe2O4/Bi2MoO6 0D QD-2D pn heterojunction photocatalyst for efficient bisphenol A degradation and Cr(VI) reduction, Appl. Surf. Sci., 2023, 611, 155607 CrossRef CAS.
- A. Dashti, M. Soodi and N. Amani, Cr(VI) induced oxidative stress and toxicity in cultured cerebellar granule neurons at different stages of development and protective effect of R osmarinic acid, Environ. Toxicol., 2016, 31, 269–277 CrossRef CAS PubMed.
- J. Y. Yue, Y. T. Wang, X. L. Ding, Y. F. Fan, L. P. Song, P. Yang, Y. Ma and B. Tang, Single-atom substitution in donor–acceptor covalent organic frameworks for tunable visible light photocatalytic Cr(VI) reduction, Mater. Chem. Front., 2022, 6, 3748–3754 RSC.
- S. Mansingh, S. Sultana, R. Acharya, M. K. Ghosh and K. M. Parida, Efficient photon conversion via double charge dynamics CeO2−BiFeO3 p–n heterojunction photocatalyst promising toward N2 fixation and phenol–Cr(VI) detoxification, Inorg. Chem., 2020, 59, 3856–3873 CrossRef CAS PubMed.
- K. K. Das, S. Patnaik, B. Nanda, A. C. Pradhan and K. Parida, ZnFe2O4−decorated mesoporous Al2O3 modified MCM–41: a solar–light–active photocatalyst for the effective removal of phenol and Cr(VI) from water, ChemistrySelect, 2019, 4, 1806–1819 CrossRef CAS.
- S. Sultana, S. Mansingh and K. M. Parida, Rational design of light induced self healed Fe based oxygen vacancy rich CeO2 (CeO2 NS–FeOOH/Fe2O3) nanostructure materials for photocatalytic water oxidation and Cr(VI) reduction, J. Mater. Chem. A, 2018, 6, 11377–11389 RSC.
- D. K. Padhi and K. Parida, Facile fabrication of α-FeOOH nanorod/RGO composite: a robust photocatalyst for reduction of Cr(VI) under visible light irradiation, J. Mater. Chem. A, 2014, 2, 10300–10312 RSC.
- D. Kandi, S. Martha, A. Thirumurugan and K. M. Parida, CdS QDs-decorated self-doped γ-Bi2MoO6: a sustainable and versatile photocatalyst toward photoreduction of Cr(VI) and degradation of phenol, ACS Omega, 2017, 2, 9040–9056 CrossRef CAS PubMed.
- J. Yang, Y. Liang, K. Li, G. Yang and S. Yin, One-step low-temperature synthesis of 0D CeO2 quantum dots/2D BiOX (X = Cl, Br) nanoplates heterojunctions for highly boosting photo-oxidation and reduction ability, Appl. Catal., B, 2019, 250, 17–30 CrossRef CAS.
- J. Liu, L. Jing, G. Gao, Y. Xu, M. Xie, L. Huang, H. Ji, J. Xie and H. Li, Ag2S quantum dots in situ coupled to hexagonal SnS2 with enhanced photocatalytic activity for MO and Cr(VI) removal, RSC Adv., 2017, 7, 46823–46831 RSC.
- Y. Yu, T. Ma and H. Huang, Semiconducting Quantum Dots for Energy Conversion and Storage, Adv. Funct. Mater., 2023, 2213770 CrossRef CAS.
- H. Jin, S. Choi, R. Velu, S. Kim and H. J. Lee, Preparation of multilayered CdSe quantum dot sensitizers by electrostatic layer-by-layer assembly and a series of post-treatments toward efficient quantum dot-sensitized mesoporous TiO2 solar cells, Langmuir, 2012, 28, 5417–5426 CrossRef CAS PubMed.
- H. Yang, Y. Liu, J. Hao, H. Tang, S. Ding, Z. Wang, F. Fang, D. Wu, W. Zhang, H. Liu and B. Xu, Alloyed green-emitting CdZnSeS/ZnS quantum dots with dense protective layers for stable lighting and display applications, ACS Appl. Mater. Interfaces, 2021, 13, 32217–32225 CrossRef CAS PubMed.
- J. Sahu, D. Prusty, S. Mansingh and K. Parida, A review on alloyed quantum dots and their applications as photocatalysts, Int. J. Hydrogen Energy, 2023, 48, 29097–29118 CrossRef CAS.
- D. Kandi, S. Martha, A. Thirumurugan and K. M. Parida, Modification of BiOI microplates with CdS QDs for enhancing stability, optical property, electronic behavior toward rhodamine B decolorization, and photocatalytic hydrogen evolution, J. Phys. Chem. C, 2017, 121, 4834–4849 CrossRef CAS.
- D. Kandi, D. P. Sahoo, S. Martha and K. Parida, Rational Design of a Coupled Confronting Z–Scheme System Toward Photocatalytic Refractory Pollutant Degradation and Water Splitting Reaction, Adv. Mater. Interfaces, 2019, 6, 1900370 CrossRef.
- D. Prusty, S. Mansingh and K. M. Parida, Synthesis of Z-schemes 0D–3D heterojunction bi-functional photocatalyst with ZnInCuS alloyed QDs supported BiOI MF for H2O2 production and N2 fixation, Catal. Sci. Technol., 2023, 13, 1311–1324 RSC.
- D. Prusty, S. Mansingh, K. K. Das, J. Sahu and K. M. Parida, Non-stoichiometric CuxIn1−xS quantum dots for robust photodegradation of gemifloxacin: influencing parameters, intermediates, and insights into the mechanism, Adv. Environ. Sci., 2022, 1, 769–780 RSC.
- Y. Nosaka and A. Y. Nosaka, Generation and detection of reactive oxygen species in photocatalysis, Chem. Rev., 2017, 117, 11302–11336 CrossRef CAS PubMed.
- S. Lin, N. Zhang, F. Wang, J. Lei, L. Zhou, Y. Liu and J. Zhang, Carbon vacancy mediated incorporation of Ti3C2 quantum dots in a 3D inverse opal g-C3N4 schottky junction catalyst for photocatalytic H2O2 production, ACS Sustainable Chem. Eng., 2020, 9, 481–488 CrossRef.
- P. Ma, X. Zhang, C. Wang, Z. Wang, K. Wang, Y. Feng, J. Wang, Y. Zhai, J. Deng, L. Wang and K. Zheng, Band alignment of homojunction by anchoring CN quantum dots on g-C3N4 (0D/2D) enhance photocatalytic hydrogen peroxide evolution, Appl. Catal., B, 2022, 300, 120736 CrossRef CAS.
- J. Xiong, X. Li, J. Huang, X. Gao, Z. Chen, J. Liu, H. Li, B. Kang, W. Yao and Y. Zhu, CN/rGO@ BPQDs high-low junctions with stretching spatial charge separation ability for photocatalytic degradation and H2O2 production, Appl. Catal., B, 2020, 266, 118602 CrossRef CAS.
- J. Xu, Q. Ji, Y. Wang, C. Wang and L. Wang, Enhanced photocatalytic H2/H2O2 production and tetracycline degradation performance of CdSe
quantum dots supported on K, P, N-co-doped hollow carbon polyhedrons, Chem. Eng. J., 2021, 426, 130808 CrossRef CAS.
- D. Prusty, L. Paramanik and K. Parida, Recent advances on alloyed quantum dots for photocatalytic hydrogen evolution: a mini-review, Energy Fuels, 2021, 35, 4670–4686 CrossRef CAS.
- J. Han, Y. Liu, F. Dai, R. Zhao and L. Wang, Fabrication of CdSe/CaTiO3 nanocomposties in aqueous solution for improved photocatalytic hydrogen production, Appl. Surf. Sci., 2018, 459, 520–526 CrossRef CAS.
- D. L. Klayman and T. S. Griffin, Reaction of selenium with sodium borohydride in protic solvents. A facile method for the introduction of selenium into organic molecules, J. Am. Chem. Soc., 1973, 95, 197–199 CrossRef CAS.
- W. Schumacher, A. Nagy, W. J. Waldman and P. K. Dutta, Direct synthesis of aqueous CdSe/ZnS-based quantum dots using microwave irradiation, J. Phys. Chem. C, 2009, 113, 12132–12139 CrossRef CAS.
- E. Aslan, O. Birinci, A. Aljabour, F. Özel, I. Akın, I. Hatay Patir, M. Kus and M. Ersoz, Photocatalytic Hydrogen Evolution by Oleic Acid–Capped CdS, CdSe, and CdS0.75Se0.25 Alloy Nanocrystals, ChemPhysChem, 2014, 15, 2668–2671 CrossRef CAS PubMed.
- X. Liu, C. Ma, Y. Yan, G. Yao, Y. Tang, P. Huo, W. Shi and Y. Yan, Hydrothermal synthesis of CdSe quantum dots and their photocatalytic activity on degradation of cefalexin, Ind. Eng. Chem. Res., 2013, 52, 15015–15023 CrossRef CAS.
- Y. Wang, J. Peng, Y. Xu, H. Bai, R. Zhao, J. Han and L. Wang, Hollow In2O3 nanotubes decorated with Cd0.67Mo0.33Se QDs for enhanced photocatalytic hydrogen production performance, Int. J. Hydrogen Energy, 2021, 46, 30393–30401 CrossRef CAS.
- T. Zhao, Z. Xing, Z. Xiu, Z. Li, S. Yang and W. Zhou, Oxygen-doped MoS2 nanospheres/CdS quantum dots/g-C3N4 nanosheets super-architectures for prolonged charge lifetime and enhanced visible-light-driven photocatalytic performance, ACS Appl. Mater. Interfaces, 2019, 11, 7104–7111 CrossRef CAS PubMed.
- L. K. Putri, B. J. Ng, W. J. Ong, H. W. Lee, W. S. Chang, A. R. Mohamed and S. P. Chai, Energy level tuning of CdSe colloidal quantum dots in ternary 0D-2D-2D CdSe QD/B-rGO/O-gC3N4 as photocatalysts for enhanced hydrogen generation, Appl. Catal., B, 2020, 265, 118592 CrossRef CAS.
- Q. Chen, H. Zhang, Y. Feng and B. Miao, High polarizability enhanced EPR, optical linear & nonlinear and electric conductivity: Role of NiFe2O4 nanocrystals in transparent tellurite glass-ceramics, J. Alloys Compd., 2023, 930, 167394 CrossRef CAS.
- X. B. Fan, S. Yu, F. Zhan, Z. J. Li, Y. J. Gao, X. B. Li, L. P. Zhang, Y. Tao, C. H. Tung and L. Z. Wu, Nonstoichiometric CuxInyS quantum dots for efficient photocatalytic hydrogen evolution, ChemSusChem, 2017, 10, 4833–4838 CrossRef CAS PubMed.
- Y. Bai, H. Zhang, B. Xiang, X. Liang, J. Hao, C. Zhu and L. Yan, Selenium defect boosted electrochemical performance of binder-free VSe2 nanosheets for aqueous zinc-ion batteries, ACS Appl. Mater. Interfaces, 2021, 13, 23230–23238 CrossRef CAS PubMed.
- S. Mansingh, S. Subudhi, S. Sultana, G. Swain and K. Parida, Cerium-based metal–organic framework nanorods nucleated on CeO2 nanosheets for photocatalytic N2 fixation and water oxidation, ACS Appl. Nano Mater., 2021, 4, 9635–9652 CrossRef CAS.
- C. Zhang, Y. Xu, C. Lv, L. Bai, J. Liao, Y. Zhai, H. Zhang and G. Chen, Amorphous engineered cerium oxides photocatalyst for efficient nitrogen fixation, Appl. Catal., B, 2020, 264, 118416 CrossRef CAS.
- B. Liu, J. Du, G. Ke, B. Jia, Y. Huang, H. He, Y. Zhou and Z. Zou, Boosting O2 reduction and H2O dehydrogenation kinetics: surface N–hydroxymethylation of g–C3N4 photocatalysts for the efficient production of H2O2, Adv. Funct. Mater., 2022, 32, 2111125 CrossRef CAS.
- R. An, Y. Zhao, H. Bai, L. Wang and C. Li, Decoration of Au NPs on hollow structured BiOBr with surface oxygen vacancies for enhanced visible light photocatalytic H2O2 evolution, J. Solid State Chem., 2022, 306, 122722 CrossRef CAS.
- H. Fattahimoghaddam, T. Mahvelati-Shamsabadi and B. K. Lee, Enhancement in photocatalytic H2O2 production over g-C3N4 nanostructures: a collaborative approach of nitrogen deficiency and supramolecular precursors, ACS Sustainable Chem. Eng., 2021, 9, 4520–4530 CrossRef CAS.
|
This journal is © The Royal Society of Chemistry 2023 |