DOI:
10.1039/D2CS00736C
(Review Article)
Chem. Soc. Rev., 2023,
52, 536-572
Multivalent glycosystems for human lectins
Received
30th August 2022
First published on 22nd December 2022
Abstract
Human lectins are involved in a wide variety of biological processes, both physiological and pathological, which have attracted the interest of the scientific community working in the glycoscience field. Multivalent glycosystems have been employed as useful tools to understand carbohydrate–lectin binding processes as well as for biomedical applications. The review shows the different scaffolds designed for a multivalent presentation of sugars and their corresponding binding studies to lectins and in some cases, their biological activities. We summarise this research by organizing based on lectin types to highlight the progression in this active field. The paper provides an overall picture of how these contributions have furnished relevant information on this topic to help in understanding and participate in these carbohydrate–lectin interactions.
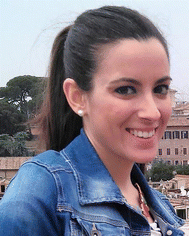
Macarena Martínez-Bailén
| Macarena Martínez Bailén obtained her PhD in Chemistry in 2019 from the University of Seville in the group of Prof. Inmaculada Robina. Her PhD was focused on the synthesis of mono- and multivalent pyrrolidine-based iminosugars and their biological evaluation as inhibitors of glycosidases of pharmacological interest. After completing her PhD she worked as a postdoctoral researcher in the University of Florence in the group of Prof. Francesca Cardona and Prof. Andrea Goti. Recently, she joined the group of Dr Javier Rojo at the Institute of Chemical Research (CSIC) and works in the preparation of glycoconjugates for their application in food allergy immunotherapy. |
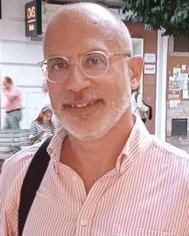
Javier Rojo
| Dr Javier Rojo obtained a PhD degree in Chemistry in 1995 from the Autónoma University of Madrid (UAM), in Spain, under the supervision of Prof. Juan Carlos Carretero. Then, he moved abroad to do postdoctoral stages with Prof. Jean-Marie Lehn at the University Louis Pasteur in Strasbourg (1995–1998) and with Prof. Larry W. McLaughlin at Boston College in USA (1998–1999). Since January 2000, he has been a member of the Carbohydrate Group at the Institute of Chemical Research of CSIC, where he is now a Research Professor. His current research interests include carbohydrate multivalent systems, bioconjugates, and molecular recognition. |
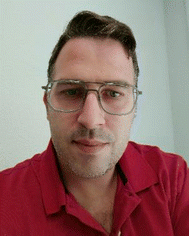
Javier Ramos-Soriano
| Javier Ramos-Soriano received his PhD in Chemistry in 2017. During 2017–2019 he pursued postdoctoral research at Universidad Complutense de Madrid to work in the synthesis of glyconanomaterials as antiviral agents. In 2019, he gained a MSCA Fellowship in Galán's group (Bristol) working on bio-inspired fluorescent glycan-nanoprobes. Recently, he joined the Spanish National Research Council (CSIC) as a Juan de la Cierva-Incorporación Posdoctoral Fellow. He has been awarded the International Carbohydrate Organisation Young Researcher Award 2022. His research interests are focused on the development of glycodendritic systems as tools to understand and intervene in biological processes related to pathogen infection and immune systems. |
1. Introduction
The term lectin was coined for the first time in 1954 by W.C. Boyd1 as plant agglutinins. Since then, the discovery of new activities for this type of protein has extended the definition to a more general term and nowadays lectins can be described as proteins with a non-immunoglobulin nature and no enzymatic activity that recognise carbohydrates. For many years, plant lectins have been used as versatile tools to interact with carbohydrates but, despite all these studies, their physiological roles in plants are still under investigation.2,3 We must go back to the ninetieth century for the first description of an animal lectin4 activity when Weir Mitchell5 demonstrated the haemagglutination caused by rattlesnake venom although the entity responsible of this activity was not identified as a lectin at that time.
Human lectins are ubiquitous in organs and tissues and show, similar to other lectins, selectivity for specific carbohydrates.6 In contrast to plant lectins, animal lectins and in particular human lectins have attracted a lot of interest due to their role in relevant biological events including inflammation, immunity, cancer, pathogen infection, etc.7–10 Many efforts have been devoted to reveal the fundamental role of these human lectins in these physiological and pathological events. In fact, some human lectins such as some C-type lectins, galectins and sialic acid-binding immunoglobulin-like lectins (Siglecs), among others, are considered important targets for the development of therapeutics for human diseases. C-type lectin receptors (CLRs) are a large family of lectins, including Dendritic Cell-Specific Intercellular adhesion molecule-3 Grabbing Non-integrin (DC-SIGN), Langerin or selectins, that bind to carbohydrates in a calcium-dependent manner. These lectins are defined by having one or more characteristic carbohydrate recognition domains (CRDs). They often oligomerise into homodimers, homotrimers, and higher-ordered oligomers, which increase their avidity for multivalent ligands.11,12 Some details of these lectins (namely DC-SIGN, Langerin, selectins, galectins and Siglecs) will be provided in the corresponding sections.
Carbohydrate–lectin interactions are characterised by a weak affinity in the millimolar range, which is compensated in nature by multivalent interactions. This fact, known as the glycocluster effect,13 increases the avidity as well as the selectivity of the recognition event. This multivalent interaction implies the participation of several copies of the carbohydrate ligand as well as the lectin's CRDs. For this reason, carbohydrate multivalent tools are necessary to get information about these binding events.14 A large variety of these multivalent platforms have been used as cores for this carbohydrate multivalent presentation, including dendrimers, dendrons, nanoparticles, polymers, liposomes, peptides, proteins, calixarenes, cyclodextrins, carbon nanoforms, etc. (Fig. 1).
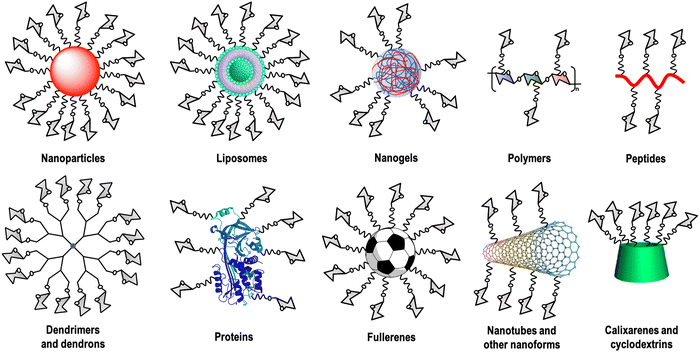 |
| Fig. 1 Different carbohydrate multivalent systems described as interacting with lectins. | |
In general, the synthesis of multivalent glycosystems requires the attachment of carbohydrate ligands (natural or synthetic ones) on scaffolds that allow multiple functionalisation with these ligands. Achieving high valences in many cases requires the use of very efficient coupling strategies. In this kind of multivalent system, several covalent bonds are generated simultaneously in one synthetic step. This is a key point in the preparation of these glycoconjugates as well as a real challenge. For instance, an inefficient coupling produces a mixture of compounds with very similar chemical and physical properties (solubility, size, shape, polarity, etc.) that are very difficult to purify in order to obtain monodisperse and discrete (macro)molecules. Due to this fact, the click chemistry15 (a synthetic approach awarded with the Nobel Prize in Chemistry in 2022) has undoubtedly emerged as a very potent and efficient tool to create carbohydrate multivalent systems.16 In particular, the copper(I) alkyne-azide cycloaddition (CuAAC) coupling reaction17–19 and the non-metal counterpart reaction, the strain promoted alkyne-azide cycloaddition (SPAAC) coupling reaction,20 have been extensively used as the chosen coupling strategy to avoid problems as it is showed in many of the examples discussed in this review. Whereas small glycosystems, such as glycodendrimers or glycodendrons, could be completely characterised by using standard spectroscopic and analytical techniques (Fourier transform infrared (FTIR) spectroscopy, NMR spectroscopy and mass spectrometry), for the characterisation of large glycosystems, different conventional techniques applied in materials sciences such as thermogravimetric analysis (TGA), Raman spectroscopy, X-ray photoelectron spectroscopy (XPS), dynamic light scattering (DLS), scanning electron microscopy (SEM), small angle X-ray scattering (SAXS) and transmission electron microscopy (TEM), among others, could provide insights into the physicochemical properties of this kind of macromolecules. For more details about the characterisation of the glycosystems described in this review, the interested reader is directed to the corresponding original references.
Numerous carbohydrate multivalent systems designed to interact with lectins can be found in the literature. The plethora of different multivalent scaffolds available facilitates the achievement of the adequate parameters to prepare glycosystems capable of interacting efficiently with lectins as demonstrated with affinities (or apparent affinities) up to the nanomolar (sometimes even in the picomolar) range. To this aim, different techniques have been used for the quantification of carbohydrate–lectin interactions. For instance, surface plasmon resonance (SPR) assays with an immobilised glycosylated protein on the biosensor chip and a particular soluble lectin (indirect competition assay) or using a particular oriented chip surface and soluble glycosystems (SPR direct interaction) provide IC50 values as well as ELISA (enzyme linked immunosorbent assay); isothermal titration calorimetry (ITC) provides various thermodynamic parameters – enthalpy, entropy, free energy (binding constant values), and stoichiometry, among others.
In this review, we will summarise the contribution of the glycoscientific community to this area, particularly during the last 10 years of developments from simple glycodendritic structures to very sophisticated ones to give an overview of the diversity of the scaffolds used. To facilitate the description of all these diverse multivalent systems, the review has been organised into chapters corresponding to the lectin target. As it can be seen below, most of this work is focused on a few human lectins, mainly DC-SIGN, Langerin, selectins, galectins and Siglecs. These lectins play well-defined relevant roles in cancer, infection, immunity, and inflammation and for this reason, they have dominated the major part of the developments in this field.
2. Dendritic cell-specific intercellular adhesion molecule-3 grabbing non-integrin (DC-SIGN)
Dendritic Cell-Specific Intercellular adhesion molecule-3 Grabbing Non-integrin, whose acronym is DC-SIGN (CD209), is a type II trans-membrane C-type lectin receptor (CLR) mainly expressed by myeloid dendritic cells (DCs) and subpopulations of macrophages, but it is also found in dermal, interstitial and monocyte-derived DCs.21,22 This lectin, characterised by a single C-terminal CRD, is assembled as a tetramer in the cellular membrane thanks to its extended coiled–coil region (the neck of the protein) that allows simultaneous presentation of four CRDs.23–26 The branched high mannose oligosaccharide (Man9GlcNAc2), a N-glycan present on glycoproteins and one of the natural ligands for this receptor, is able to interact efficiently with these CRDs. The high mannose oligosaccharide is found in several viral envelope glycoproteins such as the gp120 of HIV,27 the GP1 of Ebola28 or the spike glycoprotein of SARS-CoV-2.29 In spite of high mannose, DC-SIGN recognises other carbohydrates,23,30 mainly fucosylated oligosaccharides such as Lewis-type antigens (Lewis X (Lex) or Lewis Y (Ley)), and blood antigens in a Ca2+-dependent manner.31 Moreover, non-carbohydrate ligands as antagonists for DC-SIGN have been explored; however, the lack of selectivity has avoided the exploration of the real applications of these types of molecules.32
DC-SIGN is located in patches on the cell surface and plays undoubtedly a pivotal role in antigen capture and presentation, uptake and intracellular signalling.33 In fact, since its discovery,34 intense efforts have been devoted to unravelling the role that DC-SIGN could play in pathogen infection,35–38 since it recognises glycoconjugates expressed on the surface of several pathogens, including viruses (HIV, Ebola, Cytomegalovirus (CMV), Dengue, SARS), bacteria (M. tuberculosis, S. pneumoniae), parasites (Leishmania, S. mansoni) or fungi (C. albicans, A. fumigatus), among others.39,40 Besides the role that DC-SIGN plays in infection processes, the discovery of DC-SIGN as a target for immunomodulatory approaches,41–45 thanks to its antigen uptake and signalling properties, has attracted interest to this lectin. In this regard, DC-SIGN could be exploited as a promising candidate for the development of immunotherapies targeting DCs46,47 against important human pathologies such as cancer, allergy, and autoimmune diseases.10,48–51
In the particular case of viruses, DC-SIGN is the gate for virus entrance facilitating the cis- and/or trans-infection of cells of several viruses such as HIV,22 CMV,52 hepatitis C,53,54 Dengue,55,56 Ebola,57 Zika58 and SARS-CoV-2,59–61 among others. Due to this fact, the design of antiviral compounds with inhibitory activities capable of competing with the virus and thus blocking the homotetrameric DC-SIGN receptor can be considered a very attractive strategy and has sparked much interest in DC-SIGN targeting using carbohydrate multivalent compounds. For this purpose, the special disposition of the oligomannose binding sites close to a square and separated by around 40 Å between corners and around 55 Å for diagonals should be taken into account (Fig. 2).26 This clustering creates a multivalent surface to capture pathogens through multivalent interactions involving the recognition of highly glycosylated pathogen envelope glycoproteins. With all the information available concerning carbohydrate–protein interactions,13 the most affordable approach to increase the binding affinity, and consequently better understanding these recognition processes, requires the design of carbohydrate multivalent systems. For this aim, a plethora of different multivalent scaffolds have been used to prepare glycostructures targeting DC-SIGN.62–64 In fact, different scaffolds such as gold nanoparticles,65 liposomes,66 polymers,67 proteins,68 and dendrimers62,69 have been explored. In the present review, we will discuss the most recent developments in the synthesis of multivalent glycosystems (from small to larger ones) for targeting the DC-SIGN receptor.
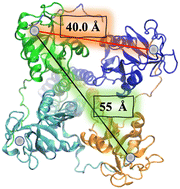 |
| Fig. 2 SAXS-derived model of the DC-SIGN ECD homotetramer. The four CRDs of DC-SIGN are exposed at the vertices of a square (around 40 Å) with a diagonal distance of ∼55 Å. For clarity, only the Ca2+ ions of the carbohydrate binding sites are represented (grey). | |
2.1. Glycodendrimers and glycodendrons
In deep contrast to other complex platforms, glycodendrimers and glycodendrons are structurally well-defined homogeneous monodisperse entities, which can be synthesised in a step-wise approach following a convergent or a divergent strategy. Many examples can be found in the literature in the last 10 years concerning the use of dendrimers and dendrons including a wide range of dendritic architectures that incorporate from simple to complex carbohydrates into their structures. This provides glycostructures with different valences and a full control of the multimeric presentation of biologically relevant carbohydrates. In particular, glycodendritic structures with different cores, repetitive units, linkers, and sugar residues have been extensively used as tools to study and interfere in biological processes involving DC-SIGN.62,70–76 A relevant example is the multimeric presentation of nine mannose residues in a multivalent scaffold based on polyalkynylated compounds as building blocks that allowed the evaluation of DC-SIGN-mediated internalisation and uptake by DCs.77 Using flow cytometry and confocal microscopy efficient interaction with no promotion of cell maturation or cytokine expression and the internalisation into the DCs within late endosomes of mannosylated glycodendron via a DC-SIGN-dependent mechanism under physiological conditions were demonstrated.
Encouraged by these promising preliminary results, our research group investigated the inhibitory ability of mannose-based glycodendrimers employing small and accessible simple scaffolds with a low-medium valency (from 4 to 18) (Fig. 3(A)).78 These scaffolds decorated with alkyne groups were coupled to mannose bearing a terminal azide in the short spacer at the anomeric position as well as to a small trivalent mannose-based glycodendron using a convergent strategy via the CuAAC click chemistry reaction. The activity of these glycodendrimers was tested in indirect competition experiments using a SPR biosensor with immobilised mannose-bovine serum albumin (Man-BSA) on the chip and the use of soluble tetrameric extracellular domain (ECD) of DC-SIGN (Fig. 3(C), left). In these experiments, a clear increase of the affinity towards DC-SIGN correlated with the increase of valency was observed for these glycodendrimers, reaching IC50 values from 767 μM for the tetravalent compound to 36 μM for the 18-valent construct (Fig. 3(A)).
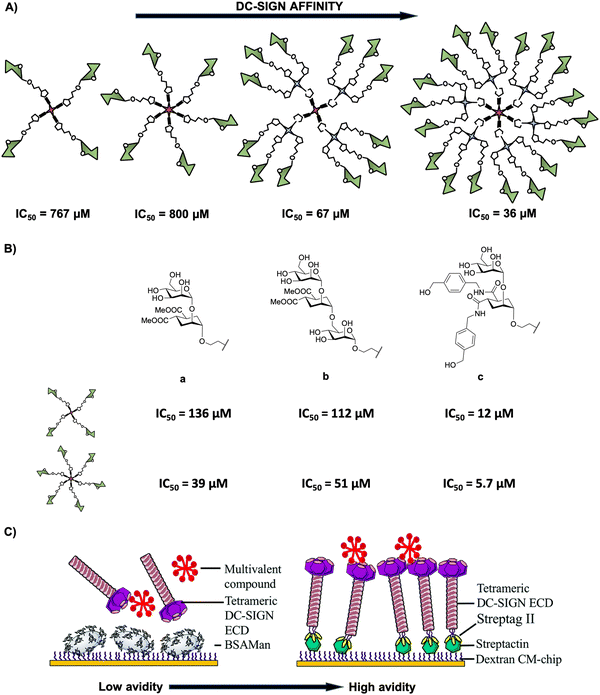 |
| Fig. 3 (A) General representation of mannosylated glycodendrimers with 4, 6, 12 and 18 copies of carbohydrates and their corresponding IC50 values. (B) Structures of pseudomannobiose (a), pseudomannotriose (b) and modified pseudodisaccharide where the methyl ester was replaced by amides with an aromatic ring (c) and their corresponding IC50 values for tetra- and hexavalent glycodendrimers. (C) Representative cartoon of SPR-based indirect (DC-SIGN vs. multivalent compound towards a BSA-Man surface, left) and direct (multivalent compound towards a DC-SIGN surface, right) assays. Reproduced with permission from ref. 80. Copyright 2020, The Royal Society of Chemistry. | |
As a good alternative to the natural sugars (O-glycosides), C-glycoside-based glycodendrimers with valences from 4 to 12 for targeting DC-SIGN have been reported by our research group in collaboration with Moravcová's group.79 Again, the results showed an increase in the affinity of these glycodendrimers with the increase of valency with similar activity (IC50 values in the micromolar range) to that found for the corresponding O-partners. The use of L-fucose and D-mannose attached by C-glycosidic bonds, instead of O-linked, improved substantially their physiological stability (against enzymatic degradation by glycosidases) as well as the binding properties due to their higher conformational dynamics. This work highlighted that C-glycomimetics could be a good alternative to natural O-sugars, being more stable compounds.
Additionally, mimetics of the mannose disaccharide Manα1,2Man and the linear trisaccharide Manα1,2Manα1,2Man were used as ligands for DC-SIGN (Fig. 3(B)-a, b). Preliminary studies of Boltorn-type glycomimetic dendrimers decorated with these pseudomannobiose (Fig. 3(B)-a) and pseudomannotriose (Fig. 3(B)-b) showed better affinities as binders to DC-SIGN and strong stability against enzymatic degradation by glycosidases compared to their corresponding natural sugars.81 Moreover, these Boltorn-type glycomimetic dendrimers were able to block both cis- and trans-infection of Ebola virus (EBOV) in the nanomolar range and the trans-infection of CD4+ cells in two HIV infection models using B-THP-1 cells expressing DC-SIGN and explant cervix tissue.82,83 Later, the synthesis of glycodendrimers with 4 and 6 copies of these glycomimetics (Fig. 3(B)-a, b) and a new modified pseudodisaccharide (Fig. 3(B)-c), an evolution of the former one previously mentioned where methyl esters were replaced by amides with an aromatic ring,78 were carried out. The affinity for DC-SIGN was improved compared to the aforementioned simple mannosylated glycodendrons (Fig. 3(A)) using the same experiment (SPR-based indirect competition assays). For instance, the new modified pseudodisaccharide hexadendrimer (IC50 of 5.7 μM) resulted to be 140- and 7-fold better than the corresponding mannose (IC50 of 800 μM) and pseudomannobiose (IC50 of 39 μM) analogues, respectively. The most interesting results were obtained for the hexavalent dendrimer with the modified pseudodisaccharide (Fig. 3(B)-c), showing an IC50 in the low μM range for the inhibition of DC-SIGN-mediated HIV-1 (as well as Dengue virus) infection of DC-SIGN+ Raji-cells in a dose-dependent manner. Additionally, this hexavalent glycodendrimer was able to inhibit HIV-1 infection of human cervical tissues showing relevant immunological properties as an adjuvant.84
More recently, Fieschi and co-workers80 tested the avidity for DC-SIGN of higher-valency glycoclusters decorated with the aforementioned glycomimetics by SPR analysis. The glycoclusters used a rigid tetravalent cyclopeptide as a core platform, differing in the way of how the 16 ligands were displayed by using the same cyclopeptide or dendrons with a higher degree of flexibility as branching points. To overcome the limits of traditional SPR-based indirect competition assays (DC-SIGN/BSA-Man surface), mainly the lack of information on affinity constants, the authors implemented a SPR direct interaction using DC-SIGN oriented chip surfaces that in some way mimics the real cell surface situation (Fig. 3(C)). This approach would be better to evaluate the surface-generated avidity preserving tetrameric DC-SIGN accessibility and the topology of its active sites. The results revealed that the scaffold architecture, the valency and the glycomimetic-based ligand play a crucial role to reach nanomolar affinities for DC-SIGN in comparison with the indirect approach where affinities were in the micromolar range. For synthesis and other examples of multivalent glycocyclopeptides, the interested reader is directed to a recent review of this issue reported by Renaudet and co-workers.85
As an alternative to cyclopeptides, calixarene or its congener, thiacalixarene, manno-86 or fucoclusters based on pseudopeptides or tetrahydroxamic acid-hydroxamic acids have been further investigated for the inhibition of DC-SIGN-dependent viral infections.80,87 Both libraries of compounds contained strong inhibitors (in the nanomolar range) for the cis-infection of DC-SIGN-Jurkat cells by viral particles pseudotyped with Ebola virus glycoprotein (EBOVGP). Moreover, these glycoclusters showed excellent results as competitive ligands for the HCMV-gB-recombinant glycoprotein interaction with monocyte-derived DCs expressing DC-SIGN. However, no significant impact of the scaffold topologies was found since similar results were obtained with 1,3-alternate conformation of the thiacalix[4]arenes and the cone conformation of calix[4]arenes (Fig. 4).
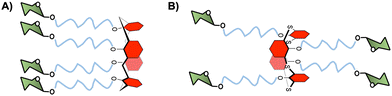 |
| Fig. 4 (A) Cone conformation of the glycocalix[4]arenes, and (B) 1,3-alternate conformation of glycothiacalix[4]arenes. | |
In spite of the increased interest in synthesising multivalent ligands (mainly glycodendrimers), especially for lectin binding,88 most strategies have been centred on the use of relatively simple, flexible and accessible scaffolds to build them in a relatively unspecific fashion, focusing to reach a high valency without a careful design. This has not provided the best approach to allow an adequate disposition and distance between sugars to simultaneously reach at least two CRDs of the DC-SIGN tetramer. For this reason, a rational design of glycodendrimers should be addressed taking into consideration the structure of DC-SIGN and the spatial orientations of its CRDs. For instance, the group of van Kooyk revealed that the distance was the most significant issue in the design of glycodendrimers more than the number of carbohydrates displayed on their structures.89 They reported the preparation of polyamidoamine (PAMAM)-based glycodendrimers from G3 to G5 generations coated with Lewis-type antigens (Lex, Lea, Leb) with a similar degree of sugar functionalisation (around 16 units). The distances between adjacent sugar units were between 1.8 and 2.7 nm depending on the generation. The larger compound (G5 Lex-PAMAM dendrimer) resulted in being the best competitor to inhibit the binding between DC-SIGN and the gp120 of HIV with a complete inhibition of the trans-infection of CD4+ cells. This type of design based on flexible and uncontrolled scaffolds could be governed by statistical rebinding effects and allowed lectin clustering in solution. In addition, the employment of very high-valency scaffolds90,91 also capitalizes on the possibility of linking simultaneously more than one binding site of the same (chelating) or different (clustering) lectin oligomer.
To overcome this issue, a careful design of effective, smaller and limited valency scaffolds could be presented as an excellent alternative to target DC-SIGN. However, it requires a delicate balance between rigidity and flexibility in order to maximize the possibility of binding events to match productively and simultaneously at least two or more lectin CRDs. Rigidity could favour the association by decreasing the entropy cost, while flexibility may help the ligand system to adapt to the lectin binding site minimizing the enthalpy cost. With an optimal rigidity–flexibility relationship, the avidity can reach several orders of magnitude as compared to that obtained only by statistical rebinding effects.92
In 2015, Bernardi and co-workers93 designed a hexavalent dendrimer composed of two trivalent dendrons78 connected by a rigid rod-like spacer in a spatially defined fashion to bridge two of the four binding sites displayed by DC-SIGN (Fig. 5(A)). The rigidity and planarity of the scaffold could tentatively favour binding by preorganising the glycomimetics, while decreasing the overall entropy of the global system. The compound with six pseudodisaccharides (Fig. 5(A)), a potent glycomimetic previously mentioned,94 inhibited the trans-infection of CD4+ T-lymphocytes by DC-SIGN mediated HIV-1 transmission with an IC50 in the nM range. More recently, it was demonstrated that this compound inhibited (i) the interaction of the SARS-CoV-2 Spike protein with DC-SIGN (IC50 of 10.4 μM, determined by SPR competition assay) and (ii) the DC-SIGN-dependent trans-infection of the SARS-CoV-2 pseudo virus as well as the wild SARS-CoV-2 virus mediated by DC-SIGN+ Jurkat cell line, which can transfer the virus to Vero E6 cells, with an IC50 of 94 nM.59 These data were consistent with the results obtained for the inhibition of HIV infection.93 However, this kind of amphiphilic structure showed a limited solubility in water (up to 2–5 × 10−3 M), allowing the formation of aggregates in an aqueous solution. This fact could generate non-desirable systems with diverse compositions, topology, and assembly dynamics with an increment of the valency and the size via non-covalent interactions. To overcome this limitation, Bernardi and co-workers95 have reported an optimised procedure based on a particular solvent protocol or via centrifugation of the samples, avoiding the formation of undesirable aggregates.
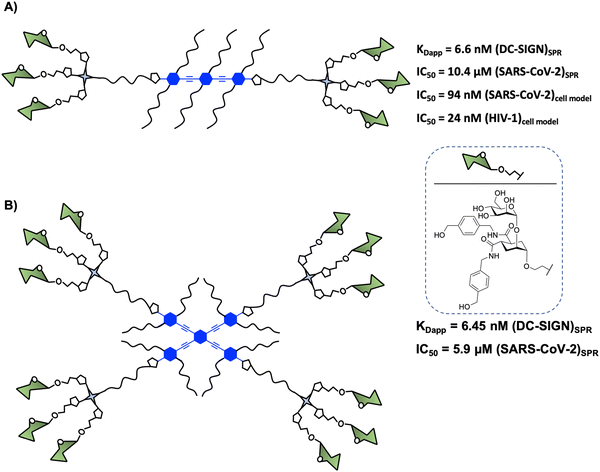 |
| Fig. 5 (A) General structure of a hexavalent dendrimer composed of two trivalent dendrons connected by a rigid rod-like spacer. (B) General structure a cross-shape glycodendrimer composed of four low-valency glycomimetic dendrons connected by a tetravalent rigid phenylene–ethylene core. IC50 and KD values of their interaction with DC-SIGN in different models are indicated for both glycodendrimers. | |
More recently, similar glycodendrimers with a linear rigid “rod-like” core of controllable length and different valences were rationally designed by the same group in order to gain better insight into the role of different binding modes (chelating, clustering and statistical rebinding) in the interaction of glycodendrimers with DC-SIGN.96 For this purpose, some of them differed in the spacer length (from 1 to 3 aromatic rings) sharing the same valency (hexavalent) and the rest of the glycodendrimers presented different valency (di- and hexavalent) sharing the same rigid rod size (three aromatic units). Combining a range of biophysical techniques such as SPR, fluorescence polarisation (FP) or ITC, and molecular modelling, it could be established that the cumulative effects of chelating and statistical rebinding modes were responsible for the high binding potency of this glycodendrimer (Fig. 5(A)) due to a high local concentration of ligands in the proximity of the carbohydrate binding site, being a potent DC-SIGN antagonist. The results also confirmed that the size of the rigid spacer is critical for chelating and thus to improve the binding avidity for DC-SIGN, with the optimal case being that with a spacer containing 3 aromatic rings (Fig. 5(A)). Moreover, they highlighted that a rigid spacer at the dendrimer core together with the length of the rod and the overall valency of the material had a strong positive effect on the IC50 values.93 Again, optimal affinity values were obtained with a long rod containing 3 aromatic rings and a short flexible linker corresponding to the glycodendrimer (Fig. 5(A)), indicating a good preorganisation of the antagonist.
Additionally, this compound, presented in Fig. 5(A), was able to be internalised by dendritic cells via DC-SIGN into the endolysosomal compartments with the production of chemokines and pro-inflammatory cytokines, modulating multiple innate responses (Th1-type response from human immature monocyte-derived DCs). This receptor-mediated internalisation endocytosis was determined by fluorescence and confocal microscopy thanks to the suboptimal intrinsic fluorescence of the rod-like spacer.45 In comparison with fluorescent dyes, one of the advantages of using this kind of spacer, with natural fluorescence, is the possibility of determining its internalisation pathway without modification of its functional interaction with the cell, at least in terms of toxicity and binding.
This glycodendrimer represents the first example that highlighted the relevance of a careful design combining three elements (an effective and selective monovalent ligand, a rigid core of appropriate length and two trivalent dendrons) to achieve high affinity and selectivity towards DC-SIGN, in comparison to other molecules where a large valency was required to reach similar activities. This elegant approach could be applied to obtain molecules capable of matching the specific size, shape and the distance between two contiguous binding sites or even CRDs at opposite corners on other relevant lectins. The conjugation of glycodendrons with potential affinity to the corresponding receptor for developing tailored lectin-targeting devices was considered. For instance, the use of sulphated glycodendrons with this core may be a potential ligand to interact with Langerin (see Section 3), a trimeric lectin, with CRDs spaced by 42 Å, close to the distance between two of the four CRDs in DC-SIGN.
Encouraged by these results, Bernardi, Fieschi and co-workers97 continued working with the multivalent rod scaffold in order to obtain simultaneous binding of the four CRDs of DC-SIGN. Taking into account that CRDs are square-like arranged and separated by around 40 Å between corners and diagonals going from 52 to 60 Å (Fig. 2), the authors carried out the synthesis of a cross-shaped glycodendrimer by CuAAC chemistry between a tetravalent rigid phenylene–ethylene core and four trivalent glycomimetic dendrons (Fig. 5(B)). The ability of this compound to bind to DC-SIGN was evaluated by the SPR direct interaction assay with immobilised targeted lectin on the chip. Unfortunately, the results obtained did not show an increase of the affinity of this glycodendrimer (Fig. 5(B), KDapp = 6.45 nM) with the increase of valency in comparison with the corresponding linear glycodendrimer (Fig. 5(A), KDapp = 6.6 nM), synthetically more accessible. The same effect was observed in SPR inhibition assays using the immobilised spike protein of SARS-CoV-2, where IC50 values of 10.4 and 5.9 μM for the linear and cross-shaped glycodendrimers, respectively, were found. Moreover, they used cell assays to test the ability of this glycodendrimer to inhibit the trans-infection of Jurkat cells by EBOVGP pseudotyped viral particles. In this case, the cross-shaped ligand proved to be more effective than the linear version at blocking DC-SIGN-mediated EBOV infection, reflecting a significant gain of avidity with increased valency. At the same concentration, the cross-shaped ligand blocked EBOV trans-infection by 96%, while the linear version was only 63% effective. These results could be explained based on the lack of translation mobility of the immobilised receptor on the SPR sensor surface in comparison with the cell model where the lectin can laterally translate and may be able to be cross-linked and clustered on the cell membrane.
Ideally, the optimal ligand presentation on a tailored geometry of the scaffold should provide strong affinity as well as selectivity towards a lectin of interest over other lectins characterised by a different spatial arrangement of their CRDs. In fact, a drawback to such multivalent glycostructures is that they cannot distinguish between human lectins that share carbohydrate binding selectivity as they often lack molecular design precision for therapeutic interventions, especially under the challenging in vivo conditions. Binding to lectins with higher and/or tunable selectivity should be based on the distances and orientations between glycan-binding sites on oligomeric defined lectins.
Regarding mannose-binding lectins, selectivity to target DC-SIGN instead of other C-type lectins, such as the homotrimeric Langerin, is indeed crucial in order to inhibit its biological functions without interfering with the protective mechanisms provided by the latter one. For instance, in the context of HIV infections, it has been demonstrated that the role of Langerin in the elimination of HIV virus, in contrast to DC-SIGN,98 is notable, with selectivity being a key point for the design of HIV-1 inhibitors. In Section 3, we provide a snapshot of the progress related to carbohydrate multivalent systems targeting Langerin. The aforementioned rigid dodecavalent glycodendrimer (Fig. 5(B)) carrying the dimannoside mimetic showed a 22-fold selectivity over Langerin.97 For the same purpose, the group of Wang99 has reported the synthesis of glycodendrimers based on a semirigid polyproline tetra-helix macrocyclic scaffold with estimated distances between corners around 3.2 nm. These glycomacromolecules functionalised with trivalent oligomannoside glycodendrons, such as a branched mannotriose or a linear Man4, were evaluated by SPR to bind to DC-SIGN and Langerin. The results revealed the effective interaction with DC-SIGN with KD in the low nanomolar range and showed an almost 4800-fold selectivity for DC-SIGN over Langerin. These enhancements could be associated with the different distances between CRDs in the trimeric Langerin, exposed in a trefoil presentation, and the tetrameric DC-SIGN, 42 and 40 Å, respectively, disfavouring binding to Langerin. The control of the oligomannose pattern on a polyproline tetra-helix macrocycle scaffold could afford selectivity but the synthesis of this scaffold implied a lot of synthetic and HPLC purification steps yielding a scarce amount of the final compound in contrast to Bernardi's scaffold. This could be a limitation in order to address both in vitro and in vivo biological studies.
In spite of efforts to obtain the ideal (both affinity and selectivity) scaffold to target DC-SIGN and to avoid cross-activity problems for other receptors, there are many parameters that cannot be easily predicted a priori with modular assembled nanoscaffolds, such as the architecture of the scaffold, the kind of linker engaged and the flexibility of the system, valency and density of glycans. In this context, glycostructures grafted with sugars or other glycomimetics more sophisticated than simple mannose or fucose monosaccharides, including the natural ligand, the high mannose, or the more synthetically-accessible Man9 epitope, would be expected.
In fact, very few examples of glycodendrimers of low valency decorated with Man9GlcNAc2 or Man9 had been reported in the literature until 2009.100–102 The main limitation of the use of the natural ligand, the high mannose, or the more synthetically-accessible epitope Man9 with β configuration at the reducing end is the synthetic complexity, preventing the accessibility to large amounts of the product required to address biological and preclinical studies. Ley103 and Seeberger,104 among others, have reported the total synthesis of Man9 oligosaccharide; however, these approaches involved many reaction steps with a low overall yield to achieve the final compound. As an alternative to these synthetic precedents, a convergent, very rapid, straightforward and high-yield synthesis of the Man9 ligand using both semi- and synthetic approaches has been recently described.105 These approaches involved shorter time consumption, lower synthesis cost, and higher overall yield of the final ligand in comparison with the others reported previously, paving the way for the preparation of multivalent systems using this ligand. In fact, glycodendrons with unspecific design (i.e. without taking into account the spatial orientations of the CRDs of DC-SIGN) containing both anomers at the reducing end, α- and β-Man9 (Fig. 6), were reported.106 Whereas the natural β-configuration in its rigid natural environment as Man9GlcNAc2-Asn could have a remarkable role in the interaction with DC-SIGN, the impact of this configuration when the ligand is attached to scaffolds through flexible linkers could be less relevant. Using fluorescence polarisation assays, it has been demonstrated that the configuration of the reducing end did not play a relevant role in binding affinity, finding similar KD in the low μM range for both anomers. Therefore, the more synthetically-accessible α-Man9 epitope can be considered as a convenient ligand for DC-SIGN with the advantage of its easy preparation compared to the β-Man9 epimer.
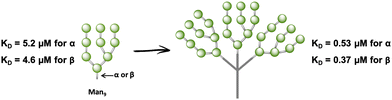 |
| Fig. 6 Cartoon of α- and β-Man9 and their corresponding glycodendrons containing both anomers at the reducing end. KD values of their interaction with DC-SIGN are indicated for both anomers. | |
On the basis of these results, new modular designs to obtain glycodendrimers with adequate disposition of the Man9 epitope to reach all four CRDs of the DC-SIGN receptor would be expected in the coming years.
2.2. Glycopolymers
Glycopolymers, alternative structures to mimic the presentation of oligosaccharides in nature, are capable of binding to lectins such as DC-SIGN.107–110 In contrast to globular scaffolds, linear polymer scaffolds fix the glycan density regardless of the chain length allowing 100% occupancy as long as carbohydrate spacing is optimised. In this context, glycopolymer architecture, valency, size, and/or density of binding elements have been explored in order to determine if these parameters can affect their DC-SIGN binding activities.111–113 For instance, the Hartmann group has recently demonstrated that the architecture of polymeric glycan mimetics can affect the binding avidity for DC-SIGN using linear and monodisperse brush glycopolymers with sequence-defined side chains.114 In fact, DC-SIGN showed higher binding to brush than to linear glycopolymers. For instance, a linear glycopolymer with 43 mannose units showed a Ka value of 32.6 × 104 M−1, whereas a brush glycopolymer with 3 side chains and decorated with a total number of mannose of 12 showed Ka = 5.4 × 104 M−1, with the last one displaying over 6-fold higher affinity to DC-SIGN. Apart from the architecture, the degree of branching, the valency per branch, the overall valency and the length of the polymeric scaffold of brush glycopolymers influenced lectin binding. This preference of DC-SIGN to ligand presentation on linear glycopolymers was also found using polydisperse linear and hyperbranched glycopolymers coated with mannosides and fucosyllactosides.115
Linear glycopolymers have been reported as potential inhibitors of the binding of HIVgp120 to DC-SIGN,116 DC-SIGN-mediated cell-infection by HIV117 or CMV118 or with advanced immunological activity.119 Additionally, cyclodextrin-based glycopolymers resulted to be inhibitors of the binding of HIV envelope glycoprotein gp120 to DC-SIGN at nanomolar concentrations.120 Interestingly, side-chain folded triblock glycopolymers bearing cyclodextrin in an extreme of the chain and adamantane in the opposite extreme for supramolecular host–guest interaction were achieved in order to evaluate the effect on DC-SIGN binding.121 Using SPR technique, the authors demonstrated that the folded polymers decorated with mannose residues enhanced greatly the multivalent binding interaction in comparison to the unfolded linear structures. In the context of the SARS-CoV-2 virus, polydisperse glycopolymers using mannose or a potent glycomimetic DC-SIGN antagonist (a triazole-based mannose derivative) were able to inhibit DC-SIGN-mediated infection and dissemination of SARS-CoV-2 virus at picomolar or nanomolar concentrations.122,123
More recently, glycopolymers obtained via an iterative exponential growth (IEG) synthetic strategy, namely glyco-IEGmers, have been reported with precisely defined and tunable sizes (containing from 8 to 32 carbohydrates units), compositions, topologies (linear or cyclic), and absolute configuration.124 Results from SPR assays with different surface-immobilised forms of human innate immune C-type lectins DC-SIGN, L-SIGN, Langerin, dectin-2 (dendritic cell-associated lectin-2), mincle (macrophage inducible C-type lectin), and DEC-205 (CD205) as well as the two collectin lectins mannose-binding lectin (MBL) and surfactant protein D (SP-D), highlighted that glyco-IEGmer length, topology, and stereochemistry have a substantial direct impact on lectin binding in many cases. From these findings, such molecular features must be considered in future glycopolymer designs for selective targeting.
2.3. Glycosylated carbon nanoforms
In the search for innovative 3D multivalent scaffolds, carbon nanoforms, such as fullerene C60, have been considered as an interesting biocompatible carbon platform for the multivalent presentation of carbohydrates.125–128 In this context, glycofullerenes with a Th octahedral symmetry and globular structure have been used simulating the carbohydrate coating on the viral surface which can interfere with the virus infection process by blocking DC-SIGN.129–131 This topic will be covered in detail in another review of this issue. However, we would like to highlight a few examples since our research group has been working in this field recently.
In brief, our group and Martín's group reported a straightforward strategy based on click reactions (both CuAAC and SPAAC)132–134 to efficiently attach twelve carbohydrate or glycodendron residues simultaneously to alkyne- or cyclooctyne-substituted C60 hexakis-adducts in few steps135 or to achieve the preparation of simple glycofullerene oligomers.136 In order to dramatically increase the valency and the size of these systems, both tridecafullerene superballs137 bearing 120 peripheral mannose or galactose (as negative control) ligands and nanoballs91 grafted with up to 360 α(1,2)mannobioside units,138 increased by 3–4 fold the antiviral activity in comparison with the corresponding mannosylated compounds.30,139,140 It is important to highlight that the giant globular multivalent glycofullerene with 360 sugar units represents the fastest dendrimeric growth reported to date.91
All these mannosylated glyconanostructures resulted in being efficient inhibitors of DC-SIGN-mediated EBOV, ZIKV and/or DENV infections and showed no cytotoxicity in cell lines at the concentrations used in the infection experiments. The results obtained in these experiments revealed the dependence of the inhibition effect on the sugar residues, suggesting that an increase of valency of these systems turned out to achieve improved antiviral activity. Using pseudotyped Ebola viral particles as infectious agents, glycofullerene, glycodendrofullerene and oligomers coated with mannose residues were able to inhibit the infection of DC-SIGN Jurkat cells with IC50s from 2000 for the simplest compound to 32 nM for the oligomers with higher number of sugars (40 mannose units), whereas the tridecafullerene superball bearing 120 pheripheral mannose showed an IC50 of 0.7 nM.135–137 Regarding nanoballs grafted with α(1,2)mannobioside units, the nanoball bearing 360 disaccharides was able to inhibit the viral infection of both Zika and Dengue viruses with an IC50 in the picomolar range (IC50 of 67 pM for ZIKV and IC50 of 35 pM for DENV).91
Moreover, glyconanomaterials constituted by a mannose glycofullerene or a nonavalent glycodendron attached to SWCNT (single-walled carbon nanotube), MWCNT (multi-walled carbon nanotube) and SWCNH (single-walled carbon nanohorn) carbon nanoforms were prepared.141 The team revealed that in addition to the size and morphology of the carbon nanoplatform used, the number of multivalent ligands (mannose residues) was also important for the recognition of DC-SIGN-mediated antiviral binding affinity in a EBOV model. In particular, the 3D architecture of MWCNTs decorated with mannose glycofullerene led to a potent EBOV inhibitor, with no significant cytotoxicity towards host cells.
These results validate the use of glycosylated carbon nanoform-based antivirals as multivalent effective probes to interact with DC-SIGN and inhibit the infection process of some viruses.
2.4. Metal glyconanoparticles
Gold nanoparticles (AuNPs) are the most stable and studied metal-based nano-clusters as multivalent platforms for presentation of carbohydrates.65,142 Among other properties, AuNPs offer a relative control of the size, the globular disposition of the carbohydrates on a large surface and the high multivalency, the facility to attach sugars using terminal thiols as well as an excellent biocompatibility and low-/non-toxicity.143 In particular, the globular disposition, similar to that of fullerenes mentioned above, allows to mimic the presentation of glycosphingolipids at the cell surface as a dense coating covering a large area, becoming a very popular approach to develop glycotools to interact with lectins,144 such as DC-SIGN.145
The group of Penadés has been pioneering the use of AuNPs as potential scaffolds for targeting DC-SIGN.65,144,146 This research group showed that these kinds of materials displaying multiple copies of different oligomannosides, from di- to heptaoligomannosides, were able to mimic the cluster presentation of oligomannosides in the HIV gp120 virus surface, which resulted to be efficient inhibitors of DC-SIGN-mediated trans-infection of human T cells.147 In a competition model using Raji-DC-SIGN cells infected with HIV-1 (JR-Renilla R5), these glycoAuNPs showed IC50 values in the nanomolar range (IC50 = 2.04 nM for di-, IC50 = 1.58 nM for tri-, IC50 = 0.34 nM for tetra-, IC50 = 0.56 nM for penta- and IC50 = 0.53 nM for hepta-mannosides).148 In addition, other carbohydrates, such as α-fucosyl-β-alanyl amide149 or galactofuranose,42 have been employed for the decoration of AuNPs targeting this receptor. However, few examples combine glycodendrons with AuNPs as powerful structural probes for multivalent lectin-glycan binding.150
Our research group reported the construction of glycoAuNPs functionalised with zwitterionic ligands and mannosyl trivalent glycodendrons to create glycodendroAuNPs with diameters smaller than 2 nm and with an intense red to near infrared fluorescence.151 The uptake mediated by multiple endocytic pathways of these AuNPs by human dendritic cells (hDCs) resulted to be 2.5-fold better than that corresponding to non-sugar decorated AuNPs. In contrast, the use of mannan as a mannose receptor (MR) and DC-SIGN inhibitor decreased by 60% the uptake only for the glycodendroAuNP, demonstrating the relevance of the sugar in facilitating the specific receptor uptake by hDCs.
More recently, Zhou and co-workers carried out the preparation of a glycoAuNP coated with the Manα1,2Man mannobioside, as well as the glycodendroAuNP using the corresponding Manα1,2Man glycodendron.152 The binding affinity to DC-SIGN was not improved by increasing the AuNP surface glycan density, showing similar KD for both nanomaterials. The lack of multivalent effect can be associated with steric congestion where not all Manα1,2Man are accessible to interact with DC-SIGN in the case of glycodendroAuNP. Moreover, the glycoAuNP was capable of inhibiting DC-SIGN-mediated EBOV infection in a similar way to that of the corresponding glycodendroAuNP (IC50s of 95 vs. 150 pM, respectively). Interestingly, rather than quantify the binding affinity, the authors exploited AuNPs’outstanding properties (fluorescence quenching, nanoscale size or high TEM contrast) in order to elucidate the different binding modes between a pair of closely related tetrameric lectins, DC-SIGN22,153 and DC-SIGNR (or L-SIGN).154
L-SIGN (CD209L), the liver/lymph node-specific homologue of DC-SIGN, shares 77% sequence homology with DC-SIGN and both related lectins recognise mannose and fucose, although they present different specificities for oligosaccharides.155,156 L-SIGN is presented at the cell surface as a tetramer as DC-SIGN; however, the special arrangement of the four CRDs in this tetramer is not the same that in the case of DC-SIGN, adopting a different conformation (Fig. 7). This fact has a relevant impact on the recognition process of multivalent carbohydrates species.153 It is well stablished that both receptors play a key role in facilitating Ebola, HIV and Zika viral infections, but they can differentially augment viral infectivity. For instance, whereas only L-SIGN can effectively drive West Nile virus infection,157 DC-SIGN was found to be more effective than L-SIGN in transmitting infections for some HIV strains.158,159
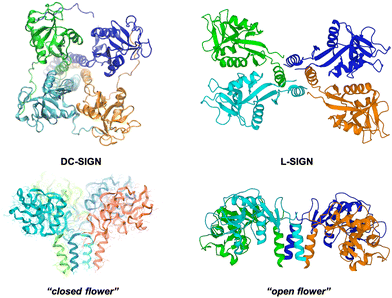 |
| Fig. 7 SAXS-derived model of the tetrameric DC-SIGN (left) and X-ray structure of the tetrameric L-SIGN (right, PDB code 1XAR) and comparison of both CRD arrangements. | |
Using Manα1,2Man glycoAuNP, the same group found that the four CRDs of DC-SIGN tetramer bound simultaneously to one glycoAuNP, whereas each glycoAuNP bound only to two of the four binding sites in L-SIGN via intercross-linking mode of binding. In addition, stronger binding affinity with DC-SIGN over L-SIGN (≈40-fold) was achieved.152 In an EBOV model, they could confirm that glycoAuNP could completely block DC-SIGN to further binding to virus surface EBOVGPs, required to initiate cell entry, but only partially block L-SIGN mediated EBOV infection via an intercross-linking binding mode (Fig. 8(A)). These results were also in agreement with those reported previously by Zhou with the cytotoxic CdSe quantum dots (QDs) grafted with the same glycans,160–163 which significantly limited its potential use as DC-SIGN targeting therapeutic agents, especially under in vivo conditions.
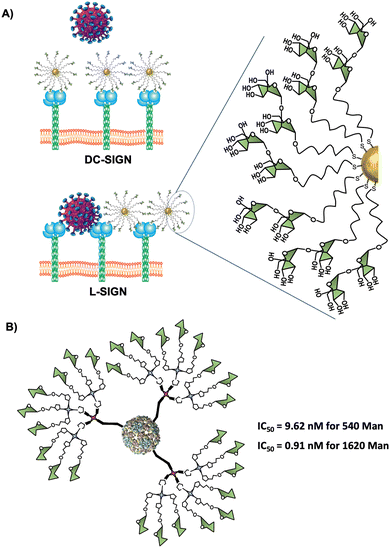 |
| Fig. 8 (A) Schematic representation of the interaction of Manα1,2Man glycoAuNP with the four binding sites of DC-SIGN inhibiting DC-SIGN-dependent viral infection and cross-linking with some binding sites or L-SIGN allowing the virus interaction with unblocked L-SIGN binding sites. (B) General structure of mannosylated glycodendron VLPs functionalised with 540 and 1620 mannose units and their corresponding IC50 values. | |
It is worth mentioning that in many cases, as described above, an increase of the dendrimer generation no longer notices affinity changes, playing a dominant role in ligand presentation. In fact, polydisperse glycoAuNPs required the optimisation of effective ligand density in order to obtain the best potencies, which are reached with an approximately 50% of epitope occupancy, probably due to steric hindrance factors.
Another type of glyconanoparticles based on magnetite (Fe3O4) have been used for highly selective capturing of DC-SIGN expressing cells (DCs) from complex cell populations164 or as dual-modal probes decorated with Lea and Leb oligosaccharides for the recognition by and internalisation into DC-SIGN expressing mammalian cells via endocytosis.165 As an alternative to obtain nanoparticles based on metals in a controlled manner, Kikkeri and co-workers166 described the synthesis of Ru(II)-based glyconanoclusters constituted by chiral Ru(II) complexes and mannose capped β-cyclodextrin using a supramolecular strategy (host–guest approach) which enantioselectively bind to specific C-type lectins: DC-SIGN over ConA and Dectin-1 lectins.
2.5. Virus-like glycoparticles
Virus-like particles (VLPs) are another source of platforms to obtain large sugar-coated structures based on the capsid protein of viruses such as the Qβ bacteriophage. They are employed as vaccine vehicles that induce cellular immunity or as a strong inhibitor in viral infections via the DC-SIGN receptor.90,167 Regarding the latter one, our research group in collaboration with the group of Prof. Davis reported the preparation of well-defined VLP coated with 540 and 1620 mannose residues (Fig. 8(B)).90 For this purpose, L-homopropargylglycine (Hpg)-bacteriophage Qβ coated proteins modified by directed mutagenesis were functionalised with trivalent and nonavalent mannosylated dendrons via a CuAAC reaction (180 covalent bonds formed simultaneously) to yield monodisperse virus-like glycodendriparticles. A strong inhibitory activity with IC50 in the low nanomolar range was found in a pseudotyped EBOV infection model using Jurkat-DC-SIGN as well as monocyte-derived DCs. In particular, VLP coated with 1620 mannose residues (IC50 of 0.91 nM) was almost one order of magnitude more potent than VLP coated with 540 mannose residues (IC50 of 9.62 nM) at inhibiting the infection process. Whereas glycoAuNPs require the optimisation of ligand density, the use of modified VLPs allows the preparation of monodisperse nanoparticles with an adequate distance and spatial disposition of glycans to interact efficiently with DC-SIGN.
2.6. Glycoliposomes
In the literature, only one recent example of liposomes coated with sugars has been reported for targeting human DC-SIGN lectin by Rademacher and co-workers.168 They highlighted that heteromultivalent liposomes coated with natural glycans and mannosides bearing aromatic aglycones showed cooperative avidity for DC-SIGN but not for Langerin. For the glycomimetic moiety, they found a secondary binding pocket located remotely from the DC-SIGN's CRD and an allosteric activation of DC-SIGN.
In brief, DC-SIGN is a lectin of tremendous interest and very active research is ongoing in this area for the design of new glycodendritic structures targeting this receptor in order to exploit polyvalent carbohydrate ligands oriented to novel immunomodulants and vaccine adjuvants as well as antiviral ligands.
3. Langerin
Langerin (CD207) is another transmembrane CLR mainly located on the surface of human Langerhans cells, a subset of immature DCs present in the mucosal and epidermal tissues.169 This pattern recognition receptor oligomerises to form a homotrimer through coiled–coil-α interaction in the neck region for optimal binding of CRDs to glycan ligands.170 The CRDs are separated by around 42 Å between the corners in an almost perfect 3-fold symmetry in the trimer (Fig. 9(B)).171 Langerin's CRDs recognise a broad range of glycan ligands containing mannosylated (mainly terminal mannoses and linear small structures) and fucosylated (such as blood antigens) oligosaccharides. Sulphated glycosaminoglycans (GAGs) such as keratan sulphate (KS), chondroitin sulphate (CS), and heparin-related GAGs are also recognised by Langerin, although these sulphated ligands interact with this lectin mainly through a positive charged groove located at the neck region.170,172–175 Langerin is involved in the recognition, uptake and clearance of pathogens, such as Mycobacteria,176 fungi177,178 and virus98,179 species, including HIV as well as self-antigens, during the first stages of the immune response.
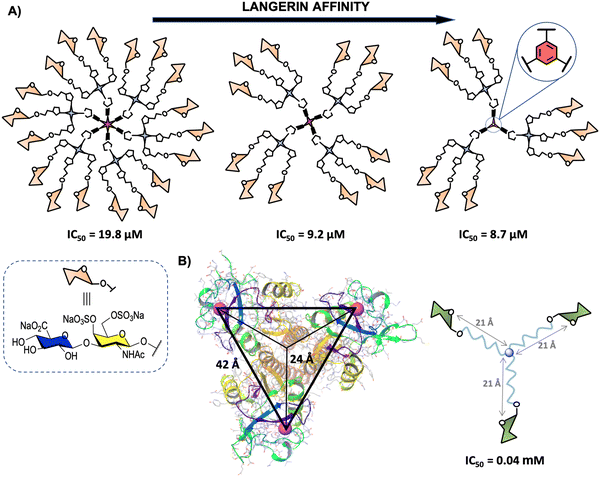 |
| Fig. 9 (A) General representation of second generation glycodendrimers with 18, 12 and 9 copies of chondroitin sulfate type-E disaccharide and their corresponding IC50 values. (B) X-ray structure of the trimeric Langerin (PDB code 3KQG) with the interbinding site distance of approximately 42 Å and 24 Å from the symmetry point and general structure of the best binder glycooligomer. For clarity, only the Ca2+ ions of the carbohydrate binding sites are represented (magenta). | |
In the particular case of GAG oligosaccharides, the high structural heterogeneity, particularly in terms of sulphate group distribution, renders the preparation of multivalent systems carrying natural GAGs rather a challenging task. In fact, only two examples, to the best of our knowledge, have been reported of GAG multivalent systems to interact with Langerin. Both examples were based on well-defined GAG disaccharides that allowed access to high-affinity ligands taking advantage of the multivalent effect in comparison with the corresponding monovalent counterparts.180,181 For the synthesis and different purposes of other GAG multivalent systems, the interested reader is directed to a recently reported review.182
The first example described the preparation of a discrete trimeric system and a linear polymer with an average degree of polymerisation of 33 units of a KS disaccharide with both 6-sulphated positions.181 A clear increase of the affinity against Langerin and the multivalent effect of these GAG glycodendrimers (IC50 of 2.7 μM and 2.1 nM for the trimer and polymer, respectively) were observed, displaying over 100 and 1000-fold higher affinity to Langerin than the monovalent systems. Moreover, the glycopolymer could efficiently bind to bone marrow-derived DCs expressing Langerin as well as modulate Langerin function whereas the monovalent system did not show detectable binding.
The second one, reported by some of us,180 involved the synthesis of a disaccharide displaying the characteristic structural motif of chondroitin sulphate type-E (CS-E) conveniently functionalised with an azido group at the reducing end.183 This disaccharide and the corresponding trivalent system were anchored to modified simple commercially available 1,3,5-trihydroxybenzene, pentaerythritol and bispentaerythritol cores via CuAAC coupling to generate first (tri-, tetra- and hexavalent) and second (nine, twelve and eighteen copies of the CS-E disaccharide) generation glycodendrimers, respectively (Fig. 9(A)).180 The activity of second generation CS-E glycodendrimers was tested in competition experiments using a SPR biosensor with Langerin and immobilised biotinylated heparin on a Strep-Tactin chip. To explore the role of the divalent cation on the interaction, the experiments were performed in the presence of Ca2+ and in the absence of this cation with the addition of EDTA. The results demonstrated a micromolar calcium-independent interaction between these glycodendrimers and Langerin with binding affinities higher than those observed for the monovalent version. Moreover, best results were obtained for the glycodendrimer containing a phenyl aromatic ring as a dendritic core, which could be exploited for the design of new high-affinity Langerin ligands. In contrast to glycodendrons depicted in Fig. 3(A), a clear decrease of the affinity towards Langerin with the increase of valency was observed for these glycodendrimers (Fig. 9(A)).
As mentioned in Section 2, the rational design of carbohydrate multivalent systems as potential binders for a lectin should start from the spatial orientations of the CRDs as well as the geometry of the targeted lectin, in this particular case, the trimeric Langerin. In this context, the simplest platform to reach simultaneously multiple CRDs directly of the Langerin trimer is a 3-armed branched scaffold. In 2019, Hartmann and co-workers184 reported the optimisation of asymmetrically branched precision mannooligomers, shortening or elongating the scaffold, by taking primary CRD distances (24 Å from the symmetry point) known from the crystal structure (Fig. 9(B)). The smallest oligomer with a distance of approximately 21 Å from the symmetry point showed the highest avidity (lowest IC50), whereas a decrease of the affinity was observed with the increment of the length of the arms through the incorporation of additional building blocks. These findings could be associated with a more coiled conformation for the glycooligomers with a higher size hampering the accessibility of the Man ligands to the binding sites.
These preliminary results highlighted that the controlled assembly of trivalent scaffolds decorated with Langerin's ligands could be tuned to increase the affinity towards this lectin and modulated to gain more insight into the selectivity towards different C-type lectin receptors. Taking into account the exceptional dual recognition capacity of Langerin with Ca2+-dependent and independent binding sites and their pivotal role in the defence mechanism against pathogen infection, such as HIV infection, more contributions in this area are expected in the coming years.
4. Selectins
Other well-studied members of the C-type lectin family are selectins.185 From the structural point of view, selectins are composed of several domains (C-terminal cytoplasmic, transmembrane, epidermal growth factor-like (EFG) and N-terminal) and a variable number of short consensus repeats (SCRs), being the tetrasaccharide sialyl Lewisx (sLex) the natural ligand. There are three members of the selectin family that are expressed by different cell types: E-selectins (CD62E) by activated endothelial cells, P-selectins (CD62P) by platelets and endothelial cells and L-selectins (CD62L) by leukocytes.186 Consequently, the target of E- and P-selectins could be interesting for monitoring endothelial cells, while focusing on L-selectins would permit the control of leukocytes. Selectins are involved in the inflammatory response by the migration of leukocytes. In diseases with an inflammatory component, an excess of leukocytes can be detrimental, so the target of leukocytes trafficking with selectin antagonists can be postulated as a promising anti-inflammatory treatment.187
Since the affinity of selectins for monovalent sLex carbohydrates is low, one strategy to target selectins is the development of multivalent carbohydrate ligands.64,188,189 Selectins have a unique CRD and appear as monomers (except for the case of P-selectin, whose dimerization has been proposed190), but this feature does not prevent the use of multivalent systems for enhancing the binding towards selectins, as other mechanisms for binding can be proposed, e.g. statistical effect (rebinding) or clustering effects (that implies the simultaneous interaction of a multivalent ligand with several lectins presented in close proximity at the cell surface) responsible for improving the affinity.
This research field have been extensively revised elsewhere,191,192 but in the last 10 years very few examples of multivalent derivatives as potential selectin ligands have been described. The Reissig's group contributed to this field with the preparation of aminopyran-derived divalent and trivalent compounds with free OH or the O-sulphated version. Unfortunately, only a small part of the prepared library could be tested in SPR experiments as selectin inhibitors due to solubility issues. Some of these compounds demonstrated inhibitory activity in the low micromolar range towards L-selectin (IC50 = 2 μM for the aminopyran-derived trivalent compound in its O-sulphated version).193 More recently, these authors have extended their methodology by employing azidopyran derivatives for the preparation of multivalent carbohydrate mimics by the CuAAC reaction. Among the O-sulphated derivatives tested, they observed inhibitory activity towards L- and P-selectins also in the micromolar range (IC50 = 0.6–100 μM for L-selectin and IC50 = 1.1–30 μM for P-selectin).194,195 Aminopyrans have also been recently employed by Tavernaro et al.196 in the development of gold, silver and iron oxide nanospheres and quantum dots for selectin targeting. Competitive SPR experiments pointed out that Au-based nanospheres containing sulphated aminopyrans behaved as L-selectin inhibitors in the low nanomolar range (IC50 = 0.35–1.9 nM). These results indicate that a higher valency achieved by preparing nanoparticles lead to more active compounds if they are compared with the derivatives of lower valence prepared by the group of Reissig.193–195 In brief, the 5–10 nm sized multivalent nanoparticles inhibited leukocyte migration and supported the importance of the application of nanocarriers in the design of systems with improved selectin-binding properties.
Other cores employed to anchoring carbohydrate motifs to study selectin binding included DNA complexes,197 liposomes198,199 and polymeric materials.200 As it will be discussed in the case of galectins (see Section 5), the N-(2-hydroxypropyl)-methacrylamide (HPMA) polymer represents an interesting backbone for the development of biocompatible systems with applications in lectin targeting. Multivalent presentation of sLex tetrasaccharides onto HPMA scaffolds was reported by Zentel and co-workers,201 who synthesised homo- and heteropolymers by incorporating an additional O-sulphated tyramine side chain. SPR experiments indicated good inhibitory activities of both types of polymers towards E-, P- and L-selectin, the heteropolymeric architecture being the one with the most interesting inhibitory profile in the low micromolar to the nanomolar range against the three selectins (IC50 = 11 μM for E-selectin, IC50 = 0.9 μM for L-selectin and IC50 = 70 nM for P-selectin). The same year, Bartneck et al.202 studied the potential of these glycopolymers to bind to different types of living cells.
The research made in this field highlights the potential of mimicking the carbohydrates displayed on the cell surfaces for achieving biocompatible drugs with application in the treatment of lectin-related diseases.
5. Galectins
Galectins (Gals) are soluble lectins implicated in a wide variety of biological processes.203,204 They play an important role in cellular adhesion and signalling205 and are divided into several families based on their topology, but all of them have a CRD with specificity for β-galactosides.108 Fifteen members of this family (Gal-1-15)204 have been described and they can be classified into three subgroups depending on their architecture: (i) prototypic galectins containing one CRD (Gal-1, 2, 5, 7, 10, 11, 13, 14 and 15); (ii) the tandem-repeat group presenting two CRDs connected by an amino acid linker (Gal-4, 6, 8, 9 and 12); and (iii) the chimera-type galectin 3 (Gal-3), which is the only member of this subgroup and possesses a single CRD and an N-terminal collagen-like tail.206 Some of these galectins seems to be specific of some species, i.e. galectin-5 and galectin-6 are found in rodents, galectin-11 reported in sheep and galectin-15 in sheep and goat.204
Selective galectin targeting is necessary for a better understanding of the mechanisms involved in the biological processes in which these lectins are implicated. As commented in Section 2, the development of lectin binders with an effective selectivity/avidity ratio would provide more efficient inhibitors in the environment of the less specific cell surface glycans and abundant serum glycoproteins. In this section, an overview of multivalent glycosystems targeting galectins is presented, organized by their multimeric architecture.
5.1. Low-valency glycosystems
Galectin-1 (Gal-1) represents an important biological target of the galectin family. This lectin, that belongs to the proto-type subgroup, may associate as a non-covalent homodimer207–209 and is implicated in the multivalent interactions with the glycoconjugates that are present on the cell surface.210–213 Some low-valency glycoclusters have been explored as galectin inhibitors.214,215 Taking into account that Gal-1 is implicated in HIV-1 binding and infectivity of CD4+T cells,216–218 St-Pierre et al.219 developed a trivalent lactoside derivative that supressed efficiently the Gal-1 mediated HIV-1 infection of host cells. The group of Vidal also explored the avidity of low-valency glycoclusters for Gal-1 by using more sophisticated tetravalent cores, i.e. porphyrin and calixarenes of different topologies.220 The binding between the tetravalent derivatives and Gal-1 was studied by haemagglutination inhibition assays (HIA) and SPR. The results obtained by HIA demonstrated a clear preference of Gal-1 for the porphyrin and the cone conformer of calixarene glycoclusters. The data obtained by HIA indicated that these tetramers inhibited haemagglutination in the micromolar range (minimum inhibitory concentration of 2–5 μM, which were lower values than the 1.25 mM obtained for the monovalent reference). However, the SPR results were not in accordance with the conclusions obtained by HIA and these derivatives displayed IC50 values in the same range (IC50 = 36–351 μM for the tetravalent compounds and IC50 = 229 μM for the monovalent counterpart). These different trends highlight the need of using distinct analytical techniques in the study of the multivalent carbohydrate–protein interactions.
Low-valency compounds can also target other lectins than Gal-1. Galectin-4 (Gal-4) is a tandem-repeat lectin highly expressed in gastrointestinal tissues and is responsible for epithelial glycoprotein transport. This protein is quite sensible to the clustered presentation of glycans.221 Murphy and co-workers222,223 reported different di-, tri- and tetravalent lactosides that displayed micromolar inhibition of Gal-3 (IC50 = 13–140 μM) and Gal-4 (IC50 = 8–120 μM) binding to asialofetuin (ASF). Some of them also proved to be effective in cell-binding assays using human pancreatic carcinoma cells. Moreover, in order to make this kind of assays more similar to the physiological situation, the authors tested some of these glycoclusters in histochemical experiments to study galectin interactions in cellular tissues.224 The multivalent interactions established between synthetic multimers (decorated with three and six carbohydrate residues) with the prototypic human Gal-7 have been mapped by X-ray crystallography and DLS experiments. In brief, the results indicated the formation of cross-linked systems with the protein in all cases.225
5.2. Glycodendrimers
Gal-3 plays a crucial role in cancerogenesis.226 For this reason, it has been considered a target for promising alternatives in cancer diagnosis and therapy. Moreover, extracellular Gal-3 is implicated in the adhesion, invasion and migration of cancer cells and can promote the apoptosis of immune cells.227–230 The survival of cancer cells is mediated by intracellular Gal-3.231–233 In this sense, the in vivo inhibition of Gal-3 binding would directly intervene in cancerous events. Multivalent presentations of Gal-3 inhibitors are adequate to enhance Gal-3 binding as this human lectin forms a pentamer or higher oligomers when is in its natural environment.234–236 In fact, it has been proposed that Gal-3 oligomerizes through its N-terminal domain and this structure controls its extracellular functions.203,235
As described in the previous sections, glycodendrimers are versatile architectures for studying lectin binding, and have also been explored in the case of galectins. Based on the high affinity of Gal-3 for lactose-derived PAMAM dendrimers described previously,237 the Cloninger's group contributed to this field with the preparation of four generations of lactose-PAMAM dendrimers containing from 15 to 100 carbohydrate residues.238 They studied the effect of these glycosystems on Gal-3 mediated cellular aggregation on three cancer cell lines (A-549 lung carcinoma cells, DU-145 prostate cancer cells, and HT-1080 fibrosarcoma cancer cells). The authors demonstrated that cellular aggregation mediated by Gal-3/MUC-1 in cancer cells can be modulated by the size of the glycodendrimers. The smallest dendrimer with 15 carbohydrate residues appeared as the most effective compound to avoid cellular aggregation, which means that there existed a competitive binding of this glycodendrimer to Gal-3, diverting Gal-3 binding to the Thomsen-Friedenreich (TF)-antigen on MUC-1 (Fig. 10(A)). In contrast, the biggest dendrimer with 100 disaccharide units promoted cellular aggregation by cross-linking (Fig. 10(A)).
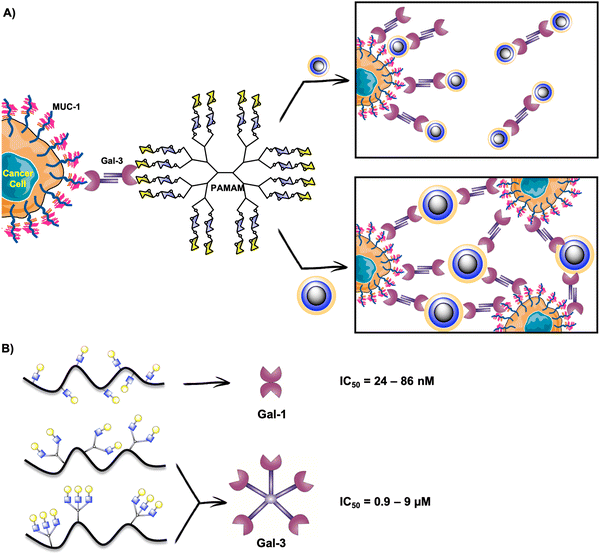 |
| Fig. 10 (A) Effect of PAMAM glycodendrimers on Gal-3 mediated cellular aggregation on cancer cells. (B) Binding preferences of LacNAc-HPMA copolymers for Gal-1 or Gal-3 depending on LacNAc presentation. | |
Later on, LacNAc functionalised PAMAM dendrimers coated with disaccharide units (between 10 and 95) prepared by chemoenzymatic synthesis were reported taking advantage of the interest of LacNAc-based materials in the investigation of galectin-mediated tumour processes. The sugar density effect of these dendrimers on cellular aggregation was studied in the same cancer cell lines (A-549, DU-145 and HT-1080). Again, glycodendrimers with a smaller number of sugar units inhibited the cellular aggregation induced by Gal-3 in the three cell lines. Larger dendrimers (with an average of 41 and 95 sugar units) induced some cellular aggregation, which could mean that larger dendrimers were capable of binding Gal-3 to form bigger Gal-3/glycodendrimer aggregates that would cross-link cells.239 In comparison with the aforementioned lactose-based dendrimers,238 LacNAc-based dendrimers inhibited cellular aggregation more effectively than the lactose analogues, even if the binding affinity of each disaccharide differs only by 3-fold potency in favour of LacNAc. This research group has also explored dendritic polyglycerols as another type of low toxic and biocompatible cores for the preparation of lactose-functionalised glycodendrimers to study Gal-3 mediated cellular aggregation with DU-145 prostate cancer cells.240 The authors observed that a higher micromolar concentration of glycodendrimer promoted cellular aggregation in analogy to the effect observed in the case of bigger lactose-PAMAM dendrimers previously reported.238 On the contrary, a lower concentration resulted in the inhibition of the formation of cellular aggregates.240
Taking into account that PAMAM glycodendrimers promoted Gal-3 aggregation,241 Cousin and Cloninger extended this methodology to target Gal-1.242 These authors prepared a family of lactose-functionalised glycodendrimers using a PAMAM scaffold similar to those previously prepared.238 The glycodendrimers promoted Gal-1 aggregation into nanoparticles and were assayed in DU-145 cancer cells to understand the role of Gal-1 in cellular aggregation and tumour formation. The competitive binding of the glycodendrimers for Gal-1 avoided the Gal-1 mediated cellular aggregation of DU-145 cells, especially in the cases of dendrimers bearing 15 and 20 glycan residues.242
As previously mentioned, the search for ligands with higher avidity and selectivity towards galectins is of high interest to study the interactions established between these lectins and glycodendrimers. The group of Cloninger has taken advantage of the versatility of PAMAM glycodendrimers for targeting Gal-1 and Gal-3 and has developed analytical tools to study the lectin–glycodendrimer interactions. The authors developed an ELISA method by the adsorption of carbohydrate-functionalised PAMAM dendrimers on polystyrene surfaces. By incorporating low affinity (galactose) or high affinity (lactose) carbohydrates into the dendrimers, the protein–carbohydrate interactions depended on the number of sugar ligands anchored to the PAMAM carrier.243 Moreover, this concept has also been exploded by Gade et al. for the preparation of heterogeneous microarrays of glycodendrons with the purpose of discriminating not only between galectins, but also for other C-type lectins.244
In order to study a distinct core for the preparation of glycodendrimers, Roy's group developed a series of glycodendrimers with an increasing number of attached lactose units by using a cyclotriphosphazene core as a branching point. The glycodendrimers containing up to 90 lactosyl moieties were evaluated as inhibitors of the proteolytically processed version of human Gal-3 (trGal-3) in a SPR assay and displayed IC50 values in the low micromolar range (IC50 = 0.16–0.55 μM). Although the inhibitory activity was better with the increase of valency, in terms of multivalent effect, the best results were obtained for dendrimers presenting 6, 10 and 15 lactose units (rp/n = 37, 53 and 28, respectively), higher valences being detrimental for the multivalent potency of the system.245
In summary, all the findings reported in the literature point out that galectin targeting by glycodendrimers may afford valuable tools for the tuning of cancerous events, leading to the development of useful systems to target cancer cells.
5.3. Polymeric glycosystems
Considering that glycopolymers have a tunable capacity to target galectins,246–248 and based on the versatility of polymeric materials that permit the creation of multivalent systems by anchoring a repeating unit in a polymeric backbone, some methacrylamide-type copolymers presenting lactose units have been reported as strong binders of Gal-3.249 The HPMA copolymer is an interesting scaffold due to its biocompatibility and water solubility.250 Chytil and co-workers251 reported that LacNAc-HPMA copolymers were able to discriminate (up to 300-fold) in a competitive ELISA assay between Gal-1 and Gal-3 based on the saccharidic architecture anchored to the polymeric HPMA carrier. An individual presentation of LacNAc afforded higher avidities for Gal-1 in the nanomolar range (IC50 = 24–86 nM for Gal-1), whereas a clustered bi- or trivalent presentation was preferred for Gal-3 (IC50 = 0.9–9 μM for Gal-3), (Fig. 10(B)). LacNAc-based tetrasaccharides anchored to the same scaffold resulted to be more potent Gal-3 inhibitors than the corresponding disaccharidic analogues (IC50 = 0.023–0.51 μM vs. IC50 = 1.7–10 μM) and demonstrated effectiveness in the inhibition of Gal-3 mediated processes such as T-lymphocyte apoptosis and the migration of human colorectal (DLD-1) and prostate (PC3) human cancer cells.252 The interactions of some of these LacNAc-based glycopolymers and their monovalent analogues with Gal-1 and Gal-3 have been recently studied by STD-NMR spectroscopy, cryo-electron microscopy (cryo-EM) and dynamic light scattering (DLS) to gain insight into the interaction framework with galectins. Unfortunately, NMR experiments were not able to determine accurately the interactions of these galectins with the glycopolymers due to their large molecular weight that was beyond the experimental limits. To solve this limitation, the authors carried out titration experiments, and the results obtained together with the conclusions raised in the study using the single building blocks indicated the formation of cross-linked systems in the case of Gal-1. Regarding Gal-3, the formation of such systems could not be determined, suggesting that the interaction would proceed through statistical effects. Cryo-EM and DLS experiments further supported that the presence of cross-linked systems was more pronounced in the case of Gal-1.253
Multivalent architectures composed of thiodigalactosides (TDGs) have been previously reported as effective Gal-3 binders.254 In this context, Vrbata et al.255 have recently extended the use of TDGs to prepare HPMA-based glycopolymers. The ELISA and biolayer interferometry studies performed by the authors demonstrated that a higher glycomimetic content in the polymer was synonym of a higher affinity for Gal-3 in the low micromolar range (IC50 = 0.48–6.2 μM). The TDGs were differently substituted at the C-3 position of the terminal galactose, and although none of the tested glycopolymers displayed not more than 4-times better selectivity towards Gal-3 over Gal-1, they behaved as anticancer agents, especially the derivative functionalised at C-3 with a 4-cyanophenyl group attached through a triazole moiety (IC50 = 0.59 μM), which was the most interesting candidate as an immunoprotective agent thanks to its antimigratory, antiproliferative and antiangiogenic properties. In brief, these results postulate water-soluble glycopolymers as potential therapeutics in Gal-3 mediated cancerous events. Due to the similar binding preferences of Gal-1 and Gal-3, the research made in the preparation of glycoderived systems that are able to discriminate between both galectins is of great interest to unveil the specific parameters that govern the mechanisms of action of these lectins.
As previously mentioned, Gal-1 targeting by arrays of carbohydrates represents an interesting alternative to enhance the binding affinity.256,257 In particular, it has been observed that multivalent ligands are capable of binding Gal-1 by the formation of aggregates through cross-linking and clustering effects.237,258,259 For instance, Belardi et al. studied Gal-1-mediated cross-linking in cell membranes by using fluorescently labelled lactose-derived glycopolymers prepared by reversible fragmentation chain transfer (RAFT) polymerisation. The glycopolymers were inserted into live cell membranes through a lipid tail incorporated in their structure that allowed at the same time a controlled orientation at the cell surface. With this methodology, these authors observed evidence for cell cross-linking mediated by Gal-1 and provided a new approach to explore the galectin interaction framework.260
Polypeptides represent another type of biodegradable polymeric materials that resemble the peptidic component of natural occurring glycoproteins.261 In this context, Heise and co-workers developed block-sequenced glycopolypeptides (octablock, tetrablock, diblock and statistical-composed) and studied their binding properties towards several lectins by SPR and turbidity experiments. These authors observed that the galactose disposition along the polypeptide backbone affected the lectin binding, and regarding Gal-3, the most favoured architecture was the tetrablock presentation, which showed the highest affinity constant (Ka = 6.81 × 105 M−1) of the series.262
5.4. Glyconanoparticles, glyconanorods and glyconanofibers
In analogy with DC-SIGN (see Section 2), nanomaterials represent versatile scaffolds that offer a wide variety of possibilities in the preparation of multivalent systems for galectin targeting. Among them, nanoparticles (Fig. 11) have been exploded by several research groups and interesting results have been reported. Pei's group developed nanospheres with numerous alkynyl and azido groups on their surface. This dual functionality allowed the incorporation of lactosyl moieties to prepare glyconanoparticles (GNPs) and subsequently a fluorescent agent to obtain fluoroglyconanoparticles (FGNPs). The GNPs exhibited specific binding for Gal-3, and the FGNPs were internalised by Jurkat cells, opening a new frontier in the development of cell imaging and drug delivery systems.263 In this context, CuAAC was also exploded in the preparation of biocompatible iron oxide GNPs. By using protein and cell chips, the galactose-functionalised glyconanoparticles showed binding affinity for Gal-9 (which is a member of the tandem-repeat subgroup and has been suggested to be implicated in the regulation of the immune system264) and an effective internalisation by human HepG2 cancer cells.265 Apart from iron, gold nanoparticles (AuNPs) decorated with lactosyl units to target Gal-3 and incorporating β-cyclodextrins moieties in order to study their drug delivery properties by the encapsulation of the anticancer drug methotrexate (MTX) have also been described. The UV-Vis studies suggested an enhanced load of MTX due to the presence of β-cyclodextrins on the surface of AuNPs.266 Other examples of AuNPs were reported by Liu et al.,267 who prepared lactose-modified chitosan (termed CTL or Chitlac) AuNPs to investigate the interactions between CTL and Gal-1 by SPR. The analysis of the interaction between Gal-1 and CTL-PEGylated AuGNPs showed the formation of agglomerates due to the high binding affinity observed (with a calculated affinity constant of 1 × 105 M−1, which was higher than that observed for lactose268). Finally, fluorescent quantum-dots coated with LacNAc moieties also demonstrated effectiveness in Gal-3 binding, improving the affinity up to 184-fold with respect to that of the monovalent LacNAc counterpart.269
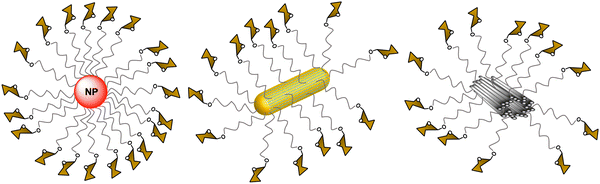 |
| Fig. 11 Representative structures (from left to right) of glyconanoparticles, glyconanorods and glyconanofibers. | |
Moving from spherical to other nanoarchitecture geometries, the preparation of lactose-functionalised gold nanorods (Fig. 11) with selective binding for Gal-3 in DLD-1 cancer cells has also been reported.270 The exploitation of this kind of interaction could be useful for the development of nanocarriers that would monitor the lectin. Moreover, self-assembled glyconanofibers (Fig. 11) decorated with LacNAc moieties along the peptidic backbone of the nanofiber displayed higher binding affinity for Gal-1 compared to Gal-3 in a qualitative SDS-PAGE analysis, and were effective in the inhibition of the Jurkat T cell apoptosis mediated by Gal-1.271 This research group further explored the effect of the nanofiber sugar composition in galectin affinity by anchoring LacdiNAc units and observed a change in the biological activity towards the selective recognition of Gal-3 in the presence of Gal-1,272 which was in accordance with the selectivity observed for this disaccharide for Gal-3.273 Surprisingly, this new generation of nanofibers did not inhibit Gal-3 mediated apoptosis of Jurkat T cells in serum media. However, when the serum content was reduced, the LacdiNAc nanofibers effectively inhibited cell death, so it was demonstrated that the serum glycoproteins competed with the nanofibers in the binding process.
In summary, this class of multivalent compounds represents an interesting alternative for the development of water-soluble bioactive systems with a tunable galectin selectivity depending on the carbohydrate content. The research made in this area further highlights the importance of the glycan presentation on the scaffolds to modulate binding events with lectins.
5.5. Glycoproteins
In contrast to the aforementioned glycopolymers (see Section 5.3), the development of neo-glycoproteins using biocompatible albumins as scaffolds permits the 3D presentation of glycans thanks to the presence of free lysine residues that are chemically modifiable.274–276 Based on previous findings that postulated LacdiNAc and LacNAc–LacNAc as ligands of Gal-3,277,278 the conjugation of tetrasaccharides composed of LacNAc–LacNAc and LacdiNAc–LacNAc units to BSA afforded multivalent neo-glycoproteins (Fig. 12(A)) whose potential to act as ligands of Gal-1 and Gal-3 was studied in an ELISA-type assay. The results showed higher affinity of the glycoproteins for Gal-3 over Gal-1, and this trend was more pronounced in the case of the LacdiNAc–LacNAc–BSA glycoproteins, which showed up to 60-fold higher binding for Gal-3 over Gal-1 when low conjugation degrees (up to 8.8 conjugated lysine residues) were used. Regarding Gal-3 binding, the ELISA assay revealed that LacdiNAc–LacNAc conjugates showed lower KD values than the LacNAc–LacNAc analogues (KD = 0.03–0.46 μM vs. KD = 0.11–0.97 μM, Fig. 12(A)), which means better binding to Gal-3.279 Further Molecular Dynamics (MD) simulations corroborated the high affinity of the LacdiNAc–LacNAc–tetrasaccharide for the binding site of Gal-3 over the LacNAc–LacNAc analogue due to the establishment of additional interactions between Gal-3 and the extra N-acetamido moiety present in the LacdiNAc moiety.280 Moreover, these authors extended this methodology for the biotinylation of the tetrasaccharide unit of the previously prepared neo-glycoproteins, and observed that at low BSA glycosylation degrees (up to an average number of 9 modified lysine residues), the new generation of glycoproteins showed a higher binding affinity for Gal-3 (KD = 0.05–0.63 μM) than the corresponding non-biotinylated counterparts (KD = 0.23–0.97 μM, data from ref. 279) and displayed an improved higher selectivity for Gal-3 over Gal-1. Nevertheless, the role of the biotin moiety in galectin binding remains unclear and more research would be needed in this aspect.281 Further studies reported a neo-glycoprotein containing LacdiNAc–LacNAc–LacNAc-based hexasaccharides as an interesting alternative to capture Gal-3 from human blood serum samples from gastrointestinal carcinoma patients.282 The truncated version of Gal-3, lacking N-terminal amino acids (1–62) due to matrix-metalloproteinase cleavage in tumour progression, has also been a subject of study.283,284
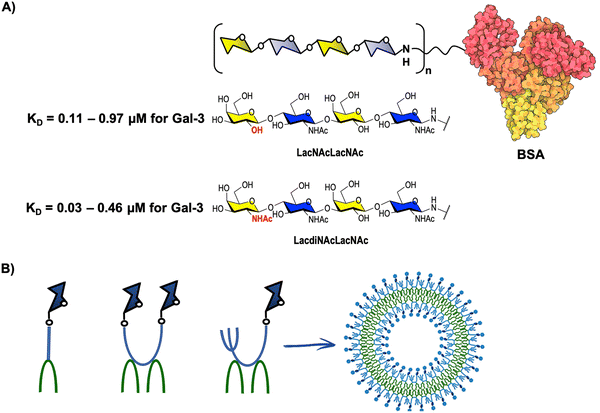 |
| Fig. 12 (A) Representative structures of LacNAc–LacNAc and LacdiNAc–LacNAc-based BSA neo-glycoproteins. (B) Different topologies (from left to right: one carbohydrate head group, two carbohydrate head groups and one carbohydrate head group combined with a hydrophilic arm of non-carbohydrate nature) used for the preparation of Janus glycodendrimers (JGDs) that self-assemble into glycodendrimersomes (GDSs). | |
Not only lactose-based glycans have been anchored to the BSA carrier, thiodigalactosides (TDGs)285 and the TF antigen (Gal(β1-3)GalNAcα)286 have also been explored for the preparation of neo-glycoproteins. Regarding TDGs, the corresponding glycoproteins displayed IC50 values in the low nanomolar range (IC50 = 1.88–19.4 nM)285 and ranked among the most potent Gal-3 inhibitors when compared with the LacNAc-based glycoproteins previously described (IC50 = 60–90 nM).279 In contrast, the multivalent presentation of an azido-functionalised version of the TF antigen onto the BSA scaffold did not afford enhanced binding affinity, but resulted effective in the inhibition of Gal-3 binding to asialofetuin (IC50 = 11–17 μM).286
The human analogue of BSA, the human serum albumin (HSA), is another adequate multivalent scaffold to prepare neo-glycoproteins that may target Gal-3. In this context, Wang et al.287 reported the construction of glycoproteins by the click chemistry reaction between an azido-functionalised HSA and an alkynyl-glycan. SPR and cell-based assays indicated that the obtained glycoprotein bound human Gal-3 selectively and inhibited the attachment of Gal-3 to human prostate and lung cancer cell lines. This study suggests that HSA-linked glycoligands may have potential in blocking the functions of Gal-3 in cancerous events. In this sense, a chemoenzymatic method combined with click chemistry reactions to develop a new family of multivalent C-3-modified LacdiNAc-based glycomimetics anchored to HSA has been recently reported.288 The corresponding multivalent glycoproteins had an inhibitory activity in the nanomolar range (IC50 = 6.5–55.8 nM) and displayed up to a 4209-fold increased inhibitory potency per glycan respect with the monovalent lactose counterpart towards Gal-3. The authors concluded that a higher degree of occupancy than five glycans per molecule of serum albumin did not afford a higher inhibitory potency and instead of that, a “plateau” is reached. These glycoproteins also rank among the most potent multivalent ligands for Gal-3 as they have IC50 values in the low nanomolar range and demonstrated to protect T-lymphocytes against Gal-3-induced apoptosis in Jurkat cells. However, when tested on colorectal adenocarcinoma DLD-1 cells, although the neo-glycoproteins demonstrated potential in targeting Gal-3, the presence of the glycomimetic moiety did not show specific binding to Gal-3 and maybe this issue needs to be further explored in order to find out the factors that control the binding process. Nevertheless, this class of glycoproteins can be of high interest as theranostic agents in biomedical research.288
5.6. Amphiphilic glycosystems
Programmable amphiphilic systems that would mimic biological membranes are versatile tools of high interest in biomedical applications and in this context, the creation of these systems has also been explored in galectin targeting. Bonduelle et al.289 contributed to this field with the development of nanoparticles composed of amphiphilic glycopolypeptides that incorporated galactose, lactose or galactan. When tested towards Gal-1 and Gal-3 in haemagglutination assays, which are based on the capacity to agglutinate red blood cells by their affinity for glycans on their surface, the nanoparticles presenting galactan displayed a binding affinity two orders of magnitude higher than the corresponding galactose or lactose counterparts did.
Later on, the group of Hartmann studied the Gal-3 binding of multivalent lactose-functionalised glycomacromolecules whose valency was further augmented by lipid-conjugation and the subsequent formation of liposomes. These authors observed that the higher is the valency of the system, the better is the inhibitory potency towards Gal-3 as showed in ELISA assays to determine the inhibition of Gal-3 binding to ASF, ranging from IC50 values of 37–123 μM for the glycomacromolecules to IC50 values of 12 μM and even to the nanomolar range for the liposomes (IC50 = 300 nM).290 Further ELISA and SPR studies on the glycomacromolecules interactions with Gal-3 revealed that the additional insertion of non-glycosidic aromatic moieties, creating heteromultivalent glycomacromolecules, enhanced Gal-3 binding from 1.5 to 3-fold, probably due to the establishment of additional hydrophobic interactions with the lectin.291
The construction of higher amphiphilic systems can be assessed by the self-assembling of Janus dendrimers (JDs) into vesicles known as dendrimersomes (DSs).292 The decoration of these dendrimers with carbohydrates taking advantage of the positive features of these molecules in lectin targeting can afford glycodendrimersomes (GDSs) that have potential applications on lectin binding.293 Percec and co-workers294 explored this methodology to prepare a series of Janus glycodendrimers with three types of topologies: (a) one carbohydrate head group; (b) two carbohydrate head groups; and (c) one carbohydrate head group combined with a hydrophilic arm of a non-carbohydrate nature, in order to study whether a higher carbohydrate excess is needed to biological targeting (Fig. 12(B)). Among them, mixed-type GDSs (c) (Fig. 12(B)) displayed the most efficient binding to human Gal-7 in agglutination assays, indicating that a diluted presentation of ligands, less sterically hindered, enhanced lectin binding. Later on, the authors performed agglutination assays with several variants of the tandem-type human Gal-8 (that differed in the amino acid linker between both CRDs) to further understand how glycan presentation on GDSs can affect their selectivity.295,296 Moreover, they have extended this methodology to study GDS interactions with other members of the galectin family such as Gal-1, Gal-2, Gal-3 and Gal-4 as well as some engineered human lectins derived from them.297–301 Other applications of GDSs involved the preparation of fluorescent hybrid vesicles by the use of bacterial membranes.302 Altogether, these results further substantiate the versatility of amphiphilic systems in galectin targeting.
The huge variety of multivalent glycocompounds reviewed in this section reflects the efforts made by the scientific community and taken together, all these studies afford interesting guidelines for the design of efficient materials with improved biological properties towards galectins.
6. Sialic acid-binding immunoglobulin-like lectins (Siglecs)
Among human lectins, sialic acid-binding immunoglobulin-like lectins (Siglecs) represent an important group of transmembrane receptors composed of 15 different types. Siglecs are mostly expressed on the surface of tumour, haematopoietic and immune cells that specifically bind sialic acid (Neu5Ac)-containing glycans.303,304 These human lectins display diverse biological functions in autoimmune diseases, brain disorders and tumoral processes.303,305–309
6.1. Glycodendrons
Recently, trivalent sialic acid glycodendrons functionalised in the focal position with different molecules such as phthalocyanine as a photosensitiser or tetraphenylethene as an aggregation-induced emission (AIE)-active fluorophore have been used to target different Siglec subtypes.310,311
Regarding the first one, Almeida-Marrero et al.310 have employed a sialic acid glycodendron zinc phthalocyanine derivative, incorporated into the membrane of small unilamellar vesicles, as multivalent ligand for targeting Siglec-10-displaying supported lipid bilayers as proof of concept (Fig. 13). Although these biohybrid systems showed a weak multivalent binding with Siglec-10, they presented superselective interactions between the vesicle and this Siglec-10 cell membrane mimic model. Although more in vitro and in vivo experiments are required, the insertion of a photosensitiser such as phthalocyanine molecules decorated with sialic acid units into liposome nanocarriers could have potential for targeting Siglec-expressing cells and delivery of photosensitiser into tumoral processes.
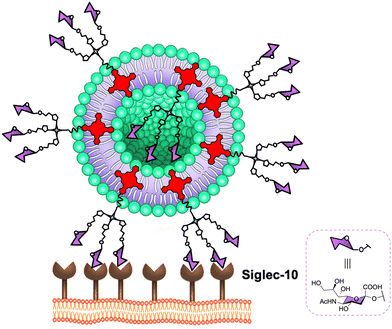 |
| Fig. 13 Schematic representation of the binding to Siglec-10 of sialic acid glycodendron zinc phthalocyanine derivative-incorporated in the membrane of small unilamellar vesicles. | |
Second, an AIE-active tetraphenylethene-functionalised trivalent sialocluster showed selective affinity to CD33-related Siglecs (Siglec-3 and Siglec-5) and the conserved Siglec (Siglec-2) in the micromolar range, measured by microscale thermophoresis (MST) techniques, but no significant binding response for BSA or ConA lectin.311 Thanks to the AIE characteristics, this system was successfully used for the selective visualisation of Siglecs expressed on the surface of mammalian cells such as cytoplasmic membrane of PC-12 cells and cervical cancer HeLa cells. By means of co-localisation experiments, it was demonstrated that the sialocluster was accumulated on cell surfaces in a patch-wise distribution manner, suggesting that it could specifically interact with Siglecs to restrict its intramolecular motions and trigger an efficient turn-on fluorescence. Moreover, trivalent MPBNeu5Ac (where MPB is m-phenoxybenzamide) glycodendrons have been functionalised with antisense oligonucleotides (ASOs) for selective targeting of B-cells through surface Siglec-2 receptor and delivery of these nucleic acid drugs into B-cancer cells.312
6.2. Glycopolymers
Bertozzi and co-workers have used cancer cells carrying lipid-anchored glycopolymers to target Siglec-7 receptor.313 This Siglec subtype is found in immunocompetent cells such as human peripheral blood natural killer (NK) cells, macrophages and monocytes303 with preferred binding of α(2,8)-linked disialic acids314 such as those displayed by ganglioside GD3. These sialic acid-containing oligosaccharides polymers were able to inhibit human NK cell activation through the recruitment of Siglec-7 and had an acquired resistance to NK cells.313
Later, more synthetically-accessible fluorescent-labelled α(2,8)-disialic acid glycopolymers obtained by polymerisation of an allene monomer containing the sugar and a π-allyl nickel complex with an azido group with an excellent polydispersity index were reported to target the same Siglec receptor.315 As a proof of concept, the authors demonstrated that these glycopolymers caused the dissociation of the Siglec-7 and GD3 interaction at a micromolar concentration range, measured by ELISA assays. For the same purpose, α(2,8) disialic acid-containing glycopolymer with a dextran backbone showing an IC50 of 1.0 nM for the inhibition of Siglec-7-GD3 interaction was also reported.316
More recently, the Bertozzi group has focused the attention on Siglec-9, which is found on macrophages and activated T cells, in order to: (a) inhibit neutrophil activation associated with COVID-2019,317 and (b) suppress the immune cell reactivity318 using sialylated glycopolymers.
6.3. Toxins- and virus-like glycoparticles
Siglec-2 (CD22) is a monomer, which is selectively expressed on B-cell lymphomas and leukemias and validated as an attractive target for cell directed cancer therapy. Paulson and co-workers have reported Siglec-2 ligands based on di- and trivalent N-glycans as multivalent scaffold and the conjugation to toxins as well.319 The authors proposed a gain of avidity from the simultaneous interaction of individual branches of the N-glycans and multiple Siglec-2 receptors. In fact, they employed BPCNeu5Ac, MPBNeu5Ac and MPBNeu5FAc (where BPC is biphenyl carbonyl and MPB is m-phenoxybenzamide) as ligands to create the multivalent glycosystems which displayed an increase of binding affinity up to 1500-fold with low nM/high pM avidity in comparison with the corresponding monovalent version. Moreover, these multivalent glycoconjugates showed selectivity for Siglec-2 over other Siglecs such as murine Siglec-2 and sialoadhesin. These multivalent ligands were conjugated to toxins, namely auristatin and saporin, affording nanoparticles that were efficiently endocytosed by Siglec-2 on B lymphoma cells and subsequently kill these cells. It is worth noting that both binding affinity for Siglec-2 and cytotoxicity of N-Glycan ligand toxin conjugates were resulted to be more efficient for triantennary derivative than biantennary ligand and the monovalent analogue, respectively. These results could be considered as an alternative to conventional antibody- and nanoparticle-mediated approaches for the delivery of agents (toxins and other cargo) to Siglec-2 bearing B lymphoma cells using the N-linked glycan scaffold. However, this approach is limited for chemoenzymatic synthesis of aforementioned type of glycans.
Alternatively to toxins, 3D bacteriophage Qβ VLP has been proposed as a multivalent scaffold to target Siglec-2 for photodynamic therapy by using of dual modification with glycan ligands and metalloporphyrin derivatives.320
6.4. Glycoliposomes
In the literature, liposomes coated with Siglec ligands have been reported for targeting human Siglec lectins (Siglecs-1, 2, 3 and 8) looking for different purposes.321–329 As a representative example from Paulson's group, therapeutic targeting of Siglec-8 for the treatment of eosinophil and mast cell disorders using liposomes functionalised with non-sulphated Neu5Acα2-3- and Neu5Acα2-6-Galβ1-4GlcNAc derivatives as Siglec-8 ligands have been used.330 The liposome decorated with 9-N-(2-naphthyl-sulfonyl)-Neu5Acα2-3-[6-O-sulfo] Galβ1-4GlcNAc (6′-O-sulfo NSANeu5Ac) showed strong binding to Siglec-8 and its closest murine functional orthologue Siglec-F, using Siglec-8 and -F expressing Chinese hamster ovary cell-binding assay and flow cytometry. Regarding the Siglec-8, the targeted liposome showed high specificity for this lectin over Siglec-3, -5, and -9. Moreover, other groups have created artificial virus nanoparticles from GM3 ganglioside-liposomes for targeting Siglec-1 (CD169) to stimulate antitumor T cell responses331 or mediated HIV-1 viral entry pathways.332,333
These results could open the door to explore new sialic acid-containing multivalent systems to target B-cells via human Siglecs receptors for innovative therapies in immunoglycotherapy, for example, delivery of agents (toxins, oligonucleotides, drugs and other cargo) to Siglec bearing B lymphoma cells in cancer immunotherapy.
7. Concluding remarks
Lectins are known since long time ago even before the name was coined. For years, these proteins were used as tools to recognise sugars. During the last few decades of the last century, the development of glycoscience and the discovery of the fundamental roles that sugars play in nature have boosted the research in this field. In this context, the scientific community has focused efforts on unravelling the role that lectins have. In particular, human lectins were very attractive due to their implication in many physiological and pathological processes. To achieve this goal, and taking into account the weak interaction with monomeric carbohydrates, sugar multivalent tools were developed with the aim to get this information. Looking at the literature, a plethora of different structures have been described. The number of parameters to be fixed to achieve an ideal structure has made this approach rather challenging. The nature and number (valency) of sugars, their spatial disposition and distribution around a platform or scaffold, the flexibility and size of these structures, the chemical nature of the scaffolds and linkers, among others issues, are relevant factors to be considered, and looking at the structures described, the diversity we can find is huge. In most of the cases, the accessibility of the scaffolds, the achievement of high valences or the preparation of the simplest compounds are the driving forces of these discoveries.
The selection of the most efficient multivalent compounds is a critical issue due to the problems to compare data obtained from the use of different techniques for determining the binding parameters of the interaction of these multivalent systems with the corresponding lectins. As it has been showed in the literature, different KD or IC50 values can be obtained for the same systems when a different technique is used for analysing the binding process. This is not surprising due to the differences in the experimental conditions (ligands and receptors in solution, one of them attached to a surface, concentrations, etc.). For this reason, it is necessary to apply more than one single technique to have a more reliable picture of the real situation. This issue could be very important to select or discard the new multivalent glycoconjugates. Moreover, binding avidities do not always correlate perfectly with biological activity. Moving from binding experiments using the isolated partners (ligands and receptors) to in vitro cellular assays and in particular in vivo experiments can change the final output. In the former cases, a different environment and the participation of other entities (serum proteins, circulating cells, etc.) can interfere with the interaction. For this reason, selection of the best candidates should be done carefully, taking into consideration all these issues.
Regarding selectivity, this is a relevant point in the design of carbohydrate multivalent systems. Lectins are sometimes very promiscuous and recognise more than one type of carbohydrate; in addition, different lectins can interact with the same type of sugars. In this context, ligand selection and in particular, the presentation of these ligands is fundamental to achieve the required selectivity. This selectivity is critical to develop compounds with excellent in vivo profiles, avoiding the presence of side effects. In this sense, efforts are focused on the development of selective glycomimetics designed for a particular receptor such is the case of the DC-SIGN versus Langerin or L-SIGN previously mentioned. This approach is focused on achieving high affinities and selectivities, which lead to compounds with good profiles. Moreover, this could also affect to the valency required to reach good affinities and therefore to the size of the multivalent systems. In fact, this should be considered in terms of the availability in vivo, the clearance and the accumulation of these compounds in some organs, which influence directly in their effectiveness and toxicity.
Over the last few years, new compounds with a careful design of the sugar presentation, considering the distances and the orientation among the multivalent carbohydrate recognition domains of the lectins, have been envisaged. This in combination with more selective ligands should lead to effective compounds that fulfil the requirements necessary for biomedical applications. Using this strategy, in particular for lectins presenting more than one CRD, better affinities have been achieved using lower valences in comparison with other multivalent systems based on high valency scaffolds. Therefore, this should be the future direction of this topic.
In this review, we have tried to collect and discuss the most notably examples described during the last few years. Considering all the findings up to now, improvements from the point of view of the carbohydrate ligands and the rational design of the scaffolds will mark the progress in the field. There is still a lot of room for new advances in this active topic and notably contributions are expected in the coming years. The implication of human lectins in diseases like cancer, inflammation, pathogen infections among others has led to consider these lectins as targets for the development of new therapeutic strategies. Carbohydrates are not ideal compounds from the drugable point of view and few examples are in the market as commercial therapeutics; moreover, carbohydrate multivalent systems as large compounds are not the prototype of therapeutics due to the reluctance of the Pharma companies to deal with these types of substances. Besides, more and more biologics are arriving to the market (i.e. antibodies) as unique alternatives to treat diseases like cancer due to the lack of effectiveness and selectivity of the actual drugs. For this reason, the door is open to new developments and contributions of potential dugs based on carbohydrate multivalent compounds.
Independent of this application, carbohydrate multivalent systems will remain as excellent tools to study lectins and the processes where they are involved furnishing key knowledge in this field.
Conflicts of interest
There are no conflicts to declare.
Acknowledgements
This research was funded by grant from MCIN/AEI/10.13039/501100011033 (PID2020-118403GB-I00) and co-funded by the European Regional Development Fund (ERDF) “A way of making Europe”. J. R.-S. acknowledges the support of Juan de la Cierva-Incorporación (IJC2020-044072-I) financed by MCIN/AEI/10.13039/501100011033 and Unión Europea NextGenerationEU/PRT. M. M.-B. thanks to the Consejería de Transformación Económica, Industria, Conocimiento y Universidades de la Junta de Andalucía for a Talent Hub Postdoctoral fellowship (POSTDOC_21_00204).
References
- W. C. Boyd and E. Shapleigh, Specific Precipitating Activity of Plant Agglutinins (Lectins), Science, 1954, 119, 419, DOI:10.1126/science.119.3091.419.
- T. De Coninck and E. J. M. Van Damme, Review: The multiple roles of plant lectins, Plant Sci., 2021, 313, 111096, DOI:10.1016/j.plantsci.2021.111096.
- M. Tsaneva and E. J. M. Van Damme, 130 years of Plant Lectin Research, Glycoconjugate J., 2020, 37, 533–551, DOI:10.1007/s10719-020-09942-y.
-
D. C. Kilpatrick, Handbook of Animal Lectins: Properties and Biomedical Applications, John Wiley & Sons, Inc., Hoboken, New Jersey, 2000 Search PubMed.
- S. Weir Mitchell, Researches upon the Venom of the Rattlesnake: with an Investigation of the Anatomy and Physiology of the Organs Concerned, Smithson. Contrib. Knowl., 1860, 12, VI Search PubMed.
- C. D. Raposo, A. B. Canelas and M. T. Barros, Human Lectins, Their Carbohydrate Affinities and Where to Find Them, Biomolecules, 2021, 11, 188, DOI:10.3390/biom11020188.
- C. P. Mason and A. W. Tarr, Human Lectins and Their Roles in Viral Infections, Molecules, 2015, 20, 2229–2271, DOI:10.3390/molecules20022229.
- T. Yau, X. Dan, C. C. W. Ng and T. B. Ng, Lectins with Potential for Anti-Cancer Therapy, Molecules, 2015, 20, 3791–3810, DOI:10.3390/molecules20033791.
- X. L. Dan and T. B. Ng, Lectins in Human Cancer: Both a Devil and an Angel?, Curr. Protein Pept. Sci., 2013, 14, 481–491, DOI:10.2174/13892037113149990065.
- G. D. Brown, J. A. Willment and L. Whitehead, C-type lectins in immunity and homeostasis, Nat. Rev. Immunol., 2018, 18, 374–389, DOI:10.1038/s41577-018-0004-8.
- A. N. Zelensky and J. E. Gready, The C-type lectin-like domain superfamily, FEBS J., 2005, 272, 6179–6217, DOI:10.1111/j.1742-4658.2005.05031.x.
- W. I. Weis, M. E. Taylor and K. Drickamer, The C-type lectin superfamily in the immune system, Immunol. Rev., 1998, 163, 19–34, DOI:10.1111/j.1600-065x.1998.tb01185.x.
- J. J. Lundquist and E. J. Toone, The Cluster Glycoside Effect, Chem. Rev., 2002, 102, 555–578, DOI:10.1021/cr000418f.
- R. Roy, P. V. Murphy and H.-J. Gabius, Multivalent Carbohydrate-Lectin Interactions: How Synthetic Chemistry Enables Insights into Nanometric Recognition, Molecules, 2016, 21, 629, DOI:10.3390/molecules21050629.
- H. C. Kolb, M. G. Finn and K. B. Sharpless, Click Chemistry: Diverse Chemical Function from a Few Good Reactions, Angew. Chem., Int. Ed., 2001, 40, 2004–2021, DOI:10.1002/1521-3773(20010601)40:11<2004::aid-anie2004>3.3.co;2-x.
- A. K. Agrahari, P. Bose, M. K. Jaiswal, S. Rajkhowa, A. S. Singh, S. Hotha, N. Mishra and V. K. Tiwari, Cu(I)-Catalyzed Click Chemistry in Glycoscience and Their Diverse Applications, Chem. Rev., 2021, 121, 7638–7956, DOI:10.1021/acs.chemrev.0c00920.
- V. V. Rostovtsev, L. G. Green, V. V. Fokin and K. B. Sharpless, A Stepwise Huisgen Cycloaddition Process: Copper(I)-Catalyzed Regioselective Ligation of Azides and Terminal Alkynes, Angew. Chem., Int. Ed., 2002, 41, 2596–2599, DOI:10.1002/1521-3773(20020715)41:14<2596::AID-ANIE2596>3.0.CO;2-4.
- C. W. Tornøe, C. Christensen and M. Meldal, Peptidotriazoles on Solid Phase:
[1,2,3]-Triazoles by Regiospecific Copper(I)-Catalyzed 1,3-Dipolar Cycloadditions of Terminal Alkynes to Azides, J. Org. Chem., 2002, 67, 3057–3064, DOI:10.1021/jo011148j.
- E. Haldón, M. C. Nicasio and P. J. Pérez, Copper-catalysed azide–alkyne cycloadditions (CuAAC): an update, Org. Biomol. Chem., 2015, 13, 9528–9550, 10.1039/C5OB01457C.
- J. Dommerholt, F. P. J. T. Rutjes and F. L. van Delft, Strain-Promoted 1,3-Dipolar Cycloaddition of Cycloalkynes and Organic Azides, Top. Curr. Chem., 2016, 374, 16, DOI:10.1007/s41061-016-0016-4.
- T. B. H. Geijtenbeek, R. Torensma, S. J. van Vliet, G. C. F. van Duijnhoven, G. J. Adema, Y. van Kooyk and C. G. Figdor, Identification of DC-SIGN, a Novel Dendritic Cell–Specific ICAM-3 Receptor that Supports Primary Immune Responses, Cell, 2000, 100, 575–585, DOI:10.1016/s0092-8674(00)80693-5.
- T. B. H. Geijtenbeek, D. S. Kwon, R. Torensma, S. J. van Vliet, G. C. F. van Duijnhoven, J. Middel, I. L. M. H. A. Cornelissen, H. S. L. M. Nottet, V. N. KewalRamani, D. R. Littman, C. G. Figdor and Y. van Kooyk, DC-SIGN, a Dendritic Cell–Specific HIV-1-Binding Protein that Enhances trans-Infection of T Cells, Cell, 2000, 100, 587–597, DOI:10.1016/s0092-8674(00)80694-7.
- D. A. Mitchell, A. J. Fadden and K. Drickamer, A Novel Mechanism of Carbohydrate Recognition by the C-type Lectins DC-SIGN and DC-SIGNR. Subunit organization and binding to multivalent ligands, J. Biol. Chem., 2001, 276, 28939–28945, DOI:10.1074/jbc.M104565200.
- H. Feinberg, Y. Guo, D. A. Mitchell, K. Drickamer and W. I. Weis, Extended Neck Regions Stabilize Tetramers of the Receptors DC-SIGN and DC-SIGNR, J. Biol. Chem., 2005, 280, 1327–1335, DOI:10.1074/jbc.M409925200.
- D. Serrano-Gómez, E. Sierra-Filardi, R. T. Martínez-Nuñez, E. Caparrós, R. Delgado, M. A. Muñoz-Fernández, M. A. Abad, J. Jimenez-Barbero, M. Leal and A. L. Corbí, Structural Requirements for Multimerization of the Pathogen Receptor Dendritic Cell-specific ICAM3-grabbing Non-integrin (CD209) on the Cell Surface, J. Biol. Chem., 2008, 283, 3889–3903, DOI:10.1074/jbc.M706004200.
- G. Tabarani, M. Thépaut, D. Stroebel, C. Ebel, C. Vivès, P. Vachette, D. Durand and F. Fieschi, DC-SIGN Neck Domain Is a pH-sensor Controlling Oligomerization. SAXS and hydrodynamic studies of extracellular domain, J. Biol. Chem., 2009, 284, 21229–21240, DOI:10.1074/jbc.M109.021204.
- K. J. Doores, C. Bonomelli, D. J. Harvey, S. Vasiljevic, R. A. Dwek, D. R. Burton, M. Crispin and C. N. Scanlan, Envelope glycans of immunodeficiency virions are almost entirely oligomannose antigens, Proc. Natl. Acad. Sci. U. S. A., 2010, 107, 13800–13805, DOI:10.1073/pnas.1006498107.
- H. Feldmann, S. T. Nichol, H.-D. Klenk, C. J. Peters and A. Sanchez, Characterization of Filoviruses Based on Differences in Structure and Antigenicity of the Virion Glycoprotein, Virology, 1994, 199, 469–473, DOI:10.1006/viro.1994.1147.
- Y. Watanabe, J. D. Allen, D. Wrapp, J. S. McLellan and M. Crispin, Site-specific glycan analysis of the SARS-CoV-2 spike, Science, 2020, 369, 330–333, DOI:10.1126/science.abb9983.
- H. Feinberg, D. A. Mitchell, K. Drickamer and W. I. Weis, Structural Basis for Selective Recognition of Oligosaccharides by DC-SIGN and DC-SIGNR, Science, 2001, 294, 2163–2166, DOI:10.1126/science.1066371.
- E. van Liempt, C. M. C. Bank, P. Mehta, J. J. García-Vallejo, Z. S. Kawar, R. Geyer, R. A. Alvarez, R. D. Cummings, Y. van Kooyk and I. van Die, Specificity of DC-SIGN for mannose- and fucose-containing glycans, FEBS Lett., 2006, 580, 6123–6131, DOI:10.1016/j.febslet.2006.10.009.
- M. J. Borrok and L. L. Kiessling, Non-carbohydrate Inhibitors of the Lectin DC-SIGN, J. Am. Chem. Soc., 2007, 129, 12780–12785, DOI:10.1021/ja072944v.
- A. Cambi, F. de Lange, N. M. van Maarseveen, M. Nijhuis, B. Joosten, E. M. H. P. van Dijk, B. I. de Bakker, J. A. M. Fransen, P. H. M. Bovee-Geurts, F. N. van Leeuwen, N. F. Van Hulst and C. G. Figdor, Microdomains of the C-type lectin
DC-SIGN are portals for virus entry into dendritic cells, J. Cell Biol., 2004, 164, 145–155, DOI:10.1083/jcb.200306112.
- B. M. Curtis, S. Scharnowske and A. J. Watson, Sequence and expression of a membrane-associated C-type lectin that exhibits CD4-independent binding of human immunodeficiency virus envelope glycoprotein gp120, Proc. Natl. Acad. Sci. U. S. A., 1992, 89, 8356–8360, DOI:10.1073/pnas.89.17.8356.
- T. B. H. Geijtenbeek and Y. van Kooyk, Pathogens target DC-SIGN to influence their fate DC-SIGN functions as a pathogen receptor with broad specificity, APMIS, 2003, 111, 698–714, DOI:10.1034/j.1600-0463.2003.11107803.x.
- Y. van Kooyk, A. Engering, A. N. Lekkerkerker, I. S. Ludwig and T. B. H. Geijtenbeek, Pathogens use carbohydrates to escape immunity induced by dendritic cells, Curr. Opin. Immunol., 2004, 16, 488–493, DOI:10.1016/j.coi.2004.05.010.
- T. B. H. Geijtenbeek, J. den Dunnen and S. I. Gringhuis, Pathogen recognition by DC-SIGN shapes adaptive immunity, Future Microbiol., 2009, 4, 879–890, DOI:10.2217/fmb.09.51.
- F. Zhang, S. Ren and Y. Zuo, DC-SIGN, DC-SIGNR and LSECtin: C-Type Lectins for Infection, Int. Rev. Immunol., 2014, 33, 54–66, DOI:10.3109/08830185.2013.834897.
- Y. van Kooyk and T. B. H. Geijtenbeek, DC-SIGN: escape mechanism for pathogens, Nat. Rev. Immunol., 2003, 3, 697–709, DOI:10.1038/nri1182.
- N. Rahimi, C-type Lectin CD209L/L-SIGN and CD209/DC-SIGN: Cell Adhesion Molecules Turned to Pathogen Recognition Receptors, Biology, 2020, 10, 1, DOI:10.3390/biology10010001.
- A. Hodges, K. Sharrocks, M. Edelmann, D. Baban, A. Moris, O. Schwartz, H. Drakesmith, K. Davies, B. Kessler, A. McMichael and A. Simmons, Activation of the lectin DC-SIGN induces an immature dendritic cell phenotype triggering Rho-GTPase activity required for HIV-1 replication, Nat. Immunol., 2007, 8, 569–577, DOI:10.1038/ni1470.
- F. Chiodo, M. Marradi, J. Park, A. F. J. Ram, S. Penadés, I. van Die and B. Tefsen, Galactofuranose-Coated Gold Nanoparticles Elicit a Pro-inflammatory Response in Human Monocyte-Derived Dendritic Cells and Are Recognized by DC-SIGN, ACS Chem. Biol., 2014, 9, 383–389, DOI:10.1021/cb4008265.
- S. I. Gringhuis, T. M. Kaptein, B. A. Wevers, M. van der Vlist, E. J. Klaver, I. van Die, L. E. M. Vriend, M. A. W. P. de Jong and T. B. H. Geijtenbeek, Fucose-based PAMPs prime dendritic cells for follicular T helper cell polarization via DC-SIGN-dependent IL-27 production, Nat. Commun., 2014, 5, 5074, DOI:10.1038/ncomms6074.
- M. Peters, P. M. Guidato, K. Peters, D. A. Megger, B. Sitek, B. Classen, E. M. Heise and A. Bufe, Allergy-Protective Arabinogalactan Modulates Human Dendritic Cells via C-Type Lectins and Inhibition of NF-κB, J. Immunol., 2016, 196, 1626–1635, DOI:10.4049/jimmunol.1502178.
- A. Berzi, S. Ordanini, B. Joosten, D. Trabattoni, A. Cambi, A. Bernardi and M. Clerici, Pseudo-Mannosylated DC-SIGN Ligands as Immunomodulants, Sci. Rep., 2016, 6, 35373, DOI:10.1038/srep35373.
- W. W. Unger, A. J. van Beelen, S. C. Bruijns, M. Joshi, C. M. Fehres, L. van Bloois, M. I. Verstege, M. Ambrosini, H. Kalay, K. Nazmi, J. G. Bolscher, E. Hooijberg, T. D. de Gruijl, G. Storm and Y. van Kooyk, Glycan-modified liposomes boost CD4+ and CD8+ T-cell responses by targeting DC-SIGN on dendritic cells, J. Controlled Release, 2012, 160, 88–95, DOI:10.1016/j.jconrel.2012.02.007.
- Y. van Kooyk, W. W. J. Unger, C. M. Fehres, H. Kalay and J. J. García-Vallejo, Glycan-based DC-SIGN targeting vaccines to enhance antigen cross-presentation, Mol. Immunol., 2013, 55, 143–145, DOI:10.1016/j.molimm.2012.10.031.
- F. Palomares, J. Ramos-Soriano, F. Gomez, A. Mascaraque, G. Bogas, J. R. Perkins, M. Gonzalez, M. J. Torres, A. Diaz-Perales, J. Rojo and C. Mayorga, Pru p 3-Glycodendropeptides Based on Mannoses Promote Changes in the Immunological Properties of Dendritic and T-Cells from LTP-Allergic Patients, Mol. Nutr. Food Res., 2019, 63, 1900553, DOI:10.1002/mnfr.201900553.
- F. Palomares, F. Gomez, M. C. de la Fuente, N. Perez-Sanchez, M. J. Torres, C. Mayorga, J. Rojo and J. Ramos-Soriano, Fucodendropeptides induce changes in cells of the immune system in food allergic patients via DC-SIGN receptor, Carbohydr. Res., 2022, 517, 108580, DOI:10.1016/j.carres.2022.108580.
- T. B. H. Geijtenbeek and S. I. Gringhuis, Signalling through C-type lectin receptors: shaping immune responses, Nat. Rev. Immunol., 2009, 9, 465–479, DOI:10.1038/nri2569.
- Y. van Kooyk and G. A. Rabinovich, Protein-glycan interactions in the control of innate and adaptive immune responses, Nat. Immunol., 2008, 9, 593–601, DOI:10.1038/ni.f.203.
- F. Halary, A. Amara, H. Lortat-Jacob, M. Messerle, T. Delaunay, C. Houlès, F. Fieschi, F. Arenzana-Seisdedos, J.-F. Moreau and J. Déchanet-Merville, Human Cytomegalovirus Binding to DC-SIGN Is Required for Dendritic Cell Infection and Target Cell trans-Infection, Immunity, 2002, 17, 653–664, DOI:10.1016/S1074-7613(02)00447-8.
- S. Pöhlmann, J. Zhang, F. Baribaud, Z. Chen, G. J. Leslie, G. Lin, A. Granelli-Piperno, R. W. Doms, C. M. Rice and J. A. McKeating, Hepatitis C Virus Glycoproteins Interact with DC-SIGN and DC-SIGNR, J. Virol., 2003, 77, 4070–4080, DOI:10.1128/JVI.77.7.4070-4080.2003.
- P. Y. Lozach, H. Lortat-Jacob, A. De Lacroix De Lavalette, I. Staropoli, S. Foung, A. Amara, C. Houlès, F. Fieschi, O. Schwartz, J.-L. Virelizier, F. Arenzana-Seisdedos and R. Altmeyer, DC-SIGN and L-SIGN Are High Affinity Binding Receptors for Hepatitis C Virus Glycoprotein E2, J. Biol. Chem., 2003, 278, 20358–20366, DOI:10.1074/jbc.M301284200.
- E. Navarro-Sanchez, R. Altmeyer, A. Amara, O. Schwartz, F. Fieschi, J.-L. Virelizier, F. Arenzana-Seisdedos and P. Desprès, Dendritic-cell-specific ICAM3-grabbing non-integrin is essential for the productive infection of human dendritic cells by mosquito-cell-derived dengue viruses, EMBO Rep., 2003, 4, 723–728, DOI:10.1038/sj.embor.embor866.
- P. Liu, M. Ridilla, P. C. Patel, L. Betts, E. Gallichotte, L. Shahidi, N. L. Thompson and K. Jacobson, Beyond attachment: roles of DC-SIGN in dengue virus infection, Traffic, 2017, 18, 218–231, DOI:10.1111/tra.12469.
- C. P. Alvarez, F. Lasala, J. Carrillo, O. Muñiz, A. L. Corbí and R. Delgado, C-Type Lectins DC-SIGN and L-SIGN Mediate Cellular Entry by Ebola Virus in cis and in trans, J. Virol., 2002, 76, 6841–6844, DOI:10.1128/JVI.76.13.6841-6844.2002.
- D. L. Carbaugh, R. S. Baric and H. M. Lazear, Envelope Protein Glycosylation Mediates Zika Virus Pathogenesis, J. Virol., 2019, 93, e00113–e00119, DOI:10.1128/JVI.00113-19.
- M. Thépaut, J. Luczkowiak, C. Vivès, N. Labiod, I. Bally, F. Lasala, Y. Grimoire, D. Fenel, S. Sattin, N. Thielens, G. Schoehn, A. Bernardi, R. Delgado and F. Fieschi, DC/L-SIGN recognition of spike glycoprotein promotes SARS-CoV-2 trans-infection and can be inhibited by a glycomimetic antagonist, PLoS Pathog., 2021, 17, e1009576, DOI:10.1371/journal.ppat.1009576.
- R. Amraei, W. Yin, M. A. Napoleon, E. L. Suder, J. Berrigan, Q. Zhao, J. Olejnik, K. B. Chandler, C. Xia, J. Feldman, B. M. Hauser, T. M. Caradonna, A. G. Schmidt, S. Gummuluru, E. Mühlberger, V. Chitalia, C. E. Costello and N. Rahimi, CD209L/L-SIGN and CD209/DC-SIGN Act as Receptors for SARS-CoV-2, ACS Cent. Sci., 2021, 7, 1156–1165, DOI:10.1021/acscentsci.0c01537.
- F. A. Lempp, L. B. Soriaga, M. Montiel-Ruiz, F. Benigni, J. Noack, Y.-J. Park, S. Bianchi, A. C. Walls, J. E. Bowen, J. Zhou, H. Kaiser, A. Joshi, M. Agostini, M. Meury, E. Dellota Jr., S. Jaconi, E. Cameroni, J. Martinez-Picado, J. Vergara-Alert, N. Izquierdo-Useros, H. W. Virgin, A. Lanzavecchia, D. Veesler, L. A. Purcell, A. Telenti and D. Corti, Lectins enhance SARS-CoV-2 infection and influence neutralizing antibodies, Nature, 2021, 598, 342–347, DOI:10.1038/s41586-021-03925-1.
- J. Ramos-Soriano and J. Rojo, Glycodendritic structures as DC-SIGN binders to inhibit viral infections, Chem. Commun., 2021, 57, 5111–5126, 10.1039/D1CC01281A.
- A. Bernardi and S. Sattin, Interfering with the Sugar Code: Ten Years Later, Eur. J. Org. Chem., 2020, 4652–4663, DOI:10.1002/ejoc.202000155.
- B. Lepenies, J. Lee and S. Sonkaria, Targeting C-type lectin receptors with multivalent carbohydrate ligands, Adv. Drug Delivery Rev., 2013, 65, 1271–1281, DOI:10.1016/j.addr.2013.05.007.
- M. Marradi, F. Chiodo, I. García and S. Penadés, Glyconanoparticles as multifunctional and multimodal carbohydrate systems, Chem. Soc. Rev., 2013, 42, 4728–4745, 10.1039/C2CS35420A.
- N. Jayaraman, K. Maiti and K. Naresh, Multivalent glycoliposomes and micelles to study carbohydrate-protein and carbohydrate-carbohydrate interactions, Chem. Soc. Rev., 2013, 42, 4640–4656, 10.1039/c3cs00001j.
- I. Pramudya and H. Chung, Recent progress of glycopolymer synthesis for biomedical applications, Biomater. Sci., 2019, 7, 4848–4872, 10.1039/c9bm01385g.
- K. Vong, T. Yamamoto and K. Tanaka, Artificial Glycoproteins as a Scaffold for Targeted Drug Therapy, Small, 2020, 16, 1906890, DOI:10.1002/smll.201906890.
- M. Sánchez-Navarro and J. Rojo, Targeting DC-SIGN with carbohydrate multivalent systems, Drug News Perspect., 2010, 23, 557–572, DOI:10.1358/dnp.2010.23.9.1437246.
- L. Dehuyser, E. Schaeffer, O. Chaloin, C. G. Mueller, R. Baati and A. Wagner, Synthesis of Novel Mannoside Glycolipid Conjugates for Inhibition of HIV-1 Trans-Infection, Bioconjugate Chem., 2012, 23, 1731–1739, DOI:10.1021/bc200644d.
- E. Blattes, A. Vercellone, H. Eutamène, C.-O. Turrin, V. Théodorou, J.-P. Majoral, A.-M. Caminade, J. Prandi, J. Nigou and G. Puzo, Mannodendrimers prevent acute lung inflammation by inhibiting neutrophil recruitment, Proc. Natl. Acad. Sci. U. S. A., 2013, 110, 8795–8800, DOI:10.1073/pnas.1221708110.
- S. Munneke, K. Kodar, G. F. Painter, B. L. Stocker and M. S. M. Timmer, The modular synthesis of multivalent functionalised glycodendrons for the detection of lectins including DC-SIGN, RSC Adv., 2017, 7, 45260–45268, 10.1039/C7RA08872H.
- G. Biagiotti, E. Purić, I. Urbančič, A. Krišelj, M. Weiss, J. Mravljak, C. Gellini, L. Lay, F. Chiodo, M. Anderluh, S. Cicchi and B. Richichi, Combining cross-coupling reaction and Knoevenagel condensation in the synthesis of glyco-BODIPY probes for DC-SIGN super-resolution bioimaging, Bioorg. Chem., 2021, 109, 104730, DOI:10.1016/j.bioorg.2021.104730.
- S. Duinkerken, S. K. Horrevorts, H. Kalay, M. Ambrosini, L. Rutte, T. D. de Gruijl, J. J. Garcia-Vallejo and Y. van Kooyk, Glyco-Dendrimers as Intradermal Anti-Tumor Vaccine Targeting Multiple Skin DC Subsets, Theranostics, 2019, 9, 5797–5809, DOI:10.7150/thno.35059.
- A. Di Maio, A. Cioce, S. Achilli, M. Thépaut, C. Vivès, F. Fieschi, J. Rojo and N.-C. Reichardt, Controlled density glycodendron microarrays for studying carbohydrate–lectin interactions, Org. Biomol. Chem., 2021, 19, 7357–7362, 10.1039/D1OB00872B.
- L. Cattiaux, V. Porkolab, F. Fieschi and J.-M. Mallet, New branched amino acids for high affinity dendrimeric DC-SIGN ligands, Bioorg. Med. Chem., 2018, 26, 1006–1015, DOI:10.1016/j.bmc.2017.12.036.
- R. Ribeiro-Viana, J. J. García-Vallejo, D. Collado, E. Pérez-Inestrosa, K. Bloem, Y. van Kooyk and J. Rojo, BODIPY-Labeled DC-SIGN-Targeting Glycodendrons Efficiently Internalize and Route to Lysosomes in Human Dendritic Cells, Biomacromolecules, 2012, 13, 3209–3219, DOI:10.1021/bm300998c.
- N. Varga, I. Sutkeviciute, R. Ribeiro-Viana, A. Berzi, R. Ramdasi, A. Daghetti, G. Vettoretti, A. Amara, M. Clerici, J. Rojo, F. Fieschi and A. Bernardi, A multivalent inhibitor of the DC-SIGN dependent uptake of HIV-1 and Dengue virus, Biomaterials, 2014, 35, 4175–4184, DOI:10.1016/j.biomaterials.2014.01.014.
- B. Bertolotti, I. Sutkeviciute, M. Ambrosini, R. Ribeiro-Viana, J. Rojo, F. Fieschi, H. Dvořáková, M. Kašáková, K. Parkan, M. Hlaváčková, K. Nováková and J. Moravcová, Polyvalent C-glycomimetics based on L-fucose or D-mannose as potent DC-SIGN antagonists, Org. Biomol. Chem., 2017, 15, 3995–4004, 10.1039/c7ob00322f.
- V. Porkolab, C. Pifferi, I. Sutkeviciute, S. Ordanini, M. Taouai, M. Thépaut, C. Vivès, M. Benazza, A. Bernardi, O. Renaudet and F. Fieschi, Development of C-type lectin-oriented surfaces for high avidity glycoconjugates: towards mimicking multivalent interactions on the cell surface, Org. Biomol. Chem., 2020, 18, 4763–4772, 10.1039/D0OB00781A.
- J. Luczkowiak, S. Sattin, I. Sutkevičiūtė, J. J. Reina, M. Sánchez-Navarro, M. Thépaut, L. Martínez-Prats, A. Daghetti, F. Fieschi, R. Delgado, A. Bernardi and J. Rojo, Pseudosaccharide Functionalized Dendrimers as Potent Inhibitors of DC-SIGN Dependent Ebola Pseudotyped Viral Infection, Bioconjugate Chem., 2011, 22, 1354–1365, DOI:10.1021/bc2000403.
- S. Sattin, A. Daghetti, M. Thépaut, A. Berzi, M. Sánchez-Navarro, G. Tabarani, J. Rojo, F. Fieschi, M. Clerici and A. Bernardi, Inhibition of DC-SIGN-Mediated HIV Infection by a Linear Trimannoside Mimic in a Tetravalent Presentation, ACS Chem. Biol., 2010, 5, 301–312, DOI:10.1021/cb900216e.
- A. Berzi, J. J. Reina, R. Ottria, I. Sutkeviciute, P. Antonazzo, M. Sanchez-Navarro, E. Chabrol, M. Biasin, D. Trabattoni, I. Cetin, J. Rojo, F. Fieschi, A. Bernardi and M. Clerici, A glycomimetic compound inhibits DC-SIGN-mediated HIV infection in cellular and cervical explant models, AIDS, 2012, 26, 127–137, DOI:10.1097/QAD.0b013e32834e1567.
- A. Berzi, N. Varga, S. Sattin, P. Antonazzo, M. Biasin, I. Cetin, D. Trabattoni, A. Bernardi and M. Clerici, Pseudo-Mannosylated DC-SIGN Ligands as Potential Adjuvants for HIV Vaccines, Viruses, 2014, 6, 391–403, DOI:10.3390/v6020391.
- D. Goyard, A. Martin-Serrano Ortiz, D. Boturyn and O. Renaudet, Multivalent glycocyclopeptides: conjugation methods and biological applications, Chem. Soc. Rev., 2022, 51, 8756–8783, 10.1039/d2cs00640e.
- I. Morbioli, V. Porkolab, A. Magini, A. Casnati, F. Fieschi and F. Sansone, Mannosylcalix[n]arenes as multivalent ligands for DC-SIGN, Carbohydr. Res., 2017, 453–454, 36–43, DOI:10.1016/j.carres.2017.10.017.
- K. Chakroun, M. Taouai, V. Porkolab, J. Luczkowiak, R. Sommer, C. Cheneau, D. Mathiron, M. A. Ben Maaouia, S. Pilard, R. Abidi, C. Mullié, F. Fieschi, P. J. Cragg, F. Halary, R. Delgado and M. Benazza, Low-Valent Calix[4]arene Glycoconjugates Based on Hydroxamic Acid Bearing Linkers as Potent Inhibitors in a Model of Ebola Virus Cis-Infection and HCMV-gB-Recombinant Glycoprotein Interaction with MDDC Cells by Blocking DC-SIGN, J. Med. Chem., 2021, 64, 14332–14343, DOI:10.1021/acs.jmedchem.1c00818.
- A. Bernardi, J. Jiménez-Barbero, A. Casnati, C. De Castro, T. Darbre, F. Fieschi, J. Finne, H. Funken, K.-E. Jaeger, M. Lahmann, T. K. Lindhorst, M. Marradi, P. Messner, A. Molinaro, P. V. Murphy, C. Nativi, S. Oscarson, S. Penadés, F. Peri, R. J. Pieters, O. Renaudet, J.-L. Reymond, B. Richichi, J. Rojo, F. Sansone, C. Schäffer, W. B. Turnbull, T. Velasco-Torrijos, S. Vidal, S. Vincent, T. Wennekes, H. Zuilhof and A. Imberty, Multivalent glycoconjugates as anti-pathogenic agents, Chem. Soc. Rev., 2013, 42, 4709–4727, 10.1039/C2CS35408J.
- J. J. Garcia-Vallejo, N. Koning, M. Ambrosini, H. Kalay, I. Vuist, R. Sarrami-Forooshani, T. B. H. Geijtenbeek and Y. van Kooyk, Glycodendrimers prevent HIV transmission via DC-SIGN on dendritic cells, Int. Immunol., 2013, 25, 221–233, DOI:10.1093/intimm/dxs115.
- R. Ribeiro-Viana, M. Sánchez-Navarro, J. Luczkowiak, J. R. Koeppe, R. Delgado, J. Rojo and B. G. Davis, Virus-like glycodendrinanoparticles displaying quasi-equivalent nested polyvalency upon glycoprotein platforms potently block viral infection, Nat. Commun., 2012, 3, 1303, DOI:10.1038/ncomms2302.
- J. Ramos-Soriano, J. J. Reina, B. M. Illescas, N. de la Cruz, L. Rodríguez-Pérez, F. Lasala, J. Rojo, R. Delgado and N. Martín, Synthesis of Highly Efficient Multivalent Disaccharide/[60]Fullerene Nanoballs for Emergent Viruses, J. Am. Chem. Soc., 2019, 141, 15403–15412, DOI:10.1021/jacs.9b08003.
- G. Bachem, E.-C. Wamhoff, K. Silberreis, D. Kim, H. Baukmann, F. Fuchsberger, J. Dernedde, C. Rademacher and O. Seitz, Rational Design of a DNA-Scaffolded High-Affinity Binder for Langerin, Angew. Chem., Int. Ed., 2020, 59, 21016–21022, DOI:10.1002/anie.202006880.
- S. Ordanini, N. Varga, V. Porkolab, M. Thépaut, L. Belvisi, A. Bertaglia, A. Palmioli, A. Berzi, D. Trabattoni, M. Clerici, F. Fieschi and A. Bernardi, Designing nanomolar antagonists of DC-SIGN-mediated HIV infection: ligand presentation using molecular rods, Chem. Commun., 2015, 51, 3816–3819, 10.1039/c4cc09709b.
- N. Varga, I. Sutkeviciute, C. Guzzi, J. McGeagh, I. Petit-Haertlein, S. Gugliotta, J. Weiser, J. Angulo, F. Fieschi and A. Bernardi, Selective Targeting of Dendritic Cell-Specific Intercellular Adhesion Molecule-3-Grabbing Nonintegrin (DC-SIGN) with Mannose-Based Glycomimetics: Synthesis and Interaction Studies of Bis(benzylamide) Derivatives of a Pseudomannobioside, Chem. – Eur. J., 2013, 19, 4786–4797, DOI:10.1002/chem.201202764.
- S. Ordanini, G. Zanchetta, V. Porkolab, C. Ebel, F. Fieschi, I. Guzzetti, D. Potenza, A. Palmioli, C. Podlipnik, D. Meroni and A. Bernardi, Solution Behavior of Amphiphilic Glycodendrimers with a Rod-Like Core, Macromol. Biosci., 2016, 16, 896–905, DOI:10.1002/mabi.201500452.
-
V. Porkolab, M. Lepšík, S. Ordanini, A. St John, A. Le Roy, M. Thépaut, E. Paci, C. Ebel, A. Bernardi and F. Fieschi, Powerful avidity with a limited valency for virus-attachment blockers on DC SIGN: Combining chelation and statistical rebinding with structural plasticity of the receptor, ChemRxiv, 2022 DOI:10.26434/chemrxiv-2022-4n79q-v2.
- G. Goti, C. Colombo, S. Achilli, C. Vivès, M. Thépaut, J. Luczkowiak, N. Labiod, R. Delgado, F. Fieschi and A. Bernardi, Precision Glycodendrimers for DC-SIGN Targeting, Eur. J. Org. Chem., 2022, e202200113, DOI:10.1002/ejoc.202200113.
- L. de Witte, A. Nabatov, M. Pion, D. Fluitsma, M. A. W. P. de Jong, T. D. de Gruijl, V. Piguet, Y. van Kooyk and T. B. H. Geijtenbeek, Langerin is a natural barrier to HIV-1 transmission by Langerhans cells, Nat. Med., 2007, 13, 367–371, DOI:10.1038/nm1541.
- H.-C. Wen, C.-H. Lin, J.-S. Huang, C.-L. Tsai, T.-F. Chen and S.-K. Wang, Selective targeting of DC-SIGN by controlling the oligomannose pattern on a polyproline tetra-helix macrocycle scaffold, Chem. Commun., 2019, 55, 9124–9127, 10.1039/c9cc03124c.
- V. Y. Dudkin, M. Orlova, X. Geng, M. Mandal, W. C. Olson and S. J. Danishefsky, Toward Fully Synthetic Carbohydrate-Based HIV Antigen Design:
On the Critical Role of Bivalency, J. Am. Chem. Soc., 2004, 126, 9560–9562, DOI:10.1021/ja047720g.
- L.-X. Wang, J. Ni, S. Singh and H. Li, Binding of High-Mannose-Type Oligosaccharides and Synthetic Oligomannose Clusters to Human Antibody 2G12. Implications for HIV-1 Vaccine Design, Chem. Biol., 2004, 11, 127–134, DOI:10.1016/j.chembiol.2003.12.020.
- S.-K. Wang, P.-H. Liang, R. D. Astronomo, T.-L. Hsu, S.-L. Hsieh, D. R. Burton and C.-H. Wong, Targeting the carbohydrates on HIV-1: Interaction of oligomannose dendrons with human monoclonal antibody 2G12 and DC-SIGN, Proc. Natl. Acad. Sci. U. S. A., 2008, 105, 3690–3695, DOI:10.1073/pnas.0712326105.
- P. Grice, S. V. Ley, J. Pietruszka, H. M. I. Osborn, H. W. M. Priepke and S. L. Warriner, A New Strategy for Oligosaccharide Assembly Exploiting Cyclohexane-1,2-diacetal Methodology: An Efficient Synthesis of a High Mannose Type Nonasaccharide, Chem. – Eur. J., 1997, 3, 431–440, DOI:10.1002/chem.19970030315.
- D. M. Ratner, O. J. Plante and P. H. Seeberger, A Linear Synthesis of Branched High-Mannose Oligosaccharides from the HIV-1 Viral Surface Envelope Glycoprotein gp120, Eur. J. Org. Chem., 2002, 826–833, DOI:10.1002/1099-0690(200203)2002:5<826::AID-EJOC826>3.0.CO;2-2.
- J. Ramos-Soriano, M. C. de la Fuente, N. de la Cruz, R. C. Figueiredo, J. Rojo and J. J. Reina, Straightforward synthesis of Man9, the relevant epitope of the high-mannose oligosaccharide, Org. Biomol. Chem., 2017, 15, 8877–8882, 10.1039/C7OB02286G.
- N. de la Cruz, J. Ramos-Soriano, J. J. Reina, J. L. de Paz, M. Thépaut, F. Fieschi, A. Sousa-Herves and J. Rojo, Influence of the reducing-end anomeric configuration of the Man9 epitope on DC-SIGN recognition, Org. Biomol. Chem., 2020, 18, 6086–6094, 10.1039/d0ob01380c.
- J. L. Jiménez Blanco, C. Ortiz Mellet and J. M. García Fernández, Multivalency in heterogeneous glycoenvironments: hetero-glycoclusters, -glycopolymers and -glycoassemblies, Chem. Soc. Rev., 2013, 42, 4518–4531, 10.1039/C2CS35219B.
- S. Cecioni, A. Imberty and S. Vidal, Glycomimetics versus Multivalent Glycoconjugates for the Design of High Affinity Lectin Ligands, Chem. Rev., 2015, 115, 525–561, DOI:10.1021/cr500303t.
- G. Yilmaz and C. R. Becer, Glyconanoparticles and their interactions with lectins, Polym. Chem., 2015, 6, 5503–5514, 10.1039/C5PY00089K.
- G. Yilmaz and C. R. Becer, Glycopolymer code based on well-defined glycopolymers or glyconanomaterials and their biomolecular recognition, Front. Bioeng. Biotechnol., 2014, 2, 39, DOI:10.3389/fbioe.2014.00039.
- G. Yilmaz, L. Messager, A. S. Gleinich, D. A. Mitchell, G. Battaglia and C. R. Becer, Glyconanoparticles with controlled morphologies and their interactions with a dendritic cell lectin, Polym. Chem., 2016, 7, 6293–6296, 10.1039/C6PY01523A.
- G. Yilmaz, V. Uzunova, M. Hartweg, V. Beyer, R. Napier and C. R. Becer, The effect of linker length on ConA and DC-SIGN binding of S-glucosyl functionalized poly(2-oxazoline)s, Polym. Chem., 2018, 9, 611–618, 10.1039/C7PY01939D.
- R.-J. E. Li, T. P. Hogervorst, S. Achilli, S. C. Bruijns, T. Arnoldus, C. Vivès, C. C. Wong, M. Thépaut, N. J. Meeuwenoord, H. van den Elst, H. S. Overkleeft, G. A. van der Marel, D. V. Filippov, S. J. van Vliet, F. Fieschi, J. D. C. Codée and Y. van Kooyk, Systematic Dual Targeting of Dendritic Cell C-Type Lectin Receptor DC-SIGN and TLR7 Using a Trifunctional Mannosylated Antigen, Front. Chem., 2019, 7, 650, DOI:10.3389/fchem.2019.00650.
- F. Shamout, A. Monaco, G. Yilmaz, C. R. Becer and L. Hartmann, Synthesis of Brush-Like Glycopolymers with Monodisperse, Sequence-Defined Side Chains and Their Interactions with Plant and Animal Lectins, Macromol. Rapid Commun., 2020, 41, 1900459, DOI:10.1002/marc.201900459.
- P. Kiran, S. Kumari, J. Dernedde, R. Haag and S. Bhatia, Synthesis and comparison of linear and hyperbranched multivalent glycosides for C-type lectin binding, New J. Chem., 2019, 43, 16012–16016, 10.1039/C9NJ02018G.
- Q. Zhang, J. Collins, A. Anastasaki, R. Wallis, D. A. Mitchell, C. R. Becer and D. M. Haddleton, Sequence-Controlled Multi-Block Glycopolymers to Inhibit DC-SIGN-gp120 Binding, Angew. Chem., Int. Ed., 2013, 52, 4435–4439, DOI:10.1002/anie.201300068.
- C. M. Jarvis, D. B. Zwick, J. C. Grim, M. M. Alam, L. R. Prost, J. C. Gardiner, S. Park, L. L. Zimdars, N. M. Sherer and L. L. Kiessling, Antigen structure affects cellular routing through DC-SIGN, Proc. Natl. Acad. Sci. U. S. A., 2019, 116, 14862–14867, DOI:10.1073/pnas.1820165116.
- S. Brument, C. Cheneau, Y. Brissonnet, D. Deniaud, F. Halary and S. G. Gouin, Polymeric mannosides prevent DC-SIGN-mediated cell-infection by cytomegalovirus, Org. Biomol. Chem., 2017, 15, 7660–7671, 10.1039/C7OB01569K.
- D. A. Mitchell, Q. Zhang, L. Voorhaar, D. M. Haddleton, S. Herath, A. S. Gleinich, H. S. Randeva, M. Crispin, H. Lehnert, R. Wallis, S. Patterson and C. R. Becer, Manipulation of cytokine secretion in human dendritic cells using glycopolymers with picomolar affinity for DC-SIGN, Chem. Sci., 2017, 8, 6974–6980, 10.1039/C7SC01515A.
- Q. Zhang, L. Su, J. Collins, G. Chen, R. Wallis, D. A. Mitchell, D. M. Haddleton and C. R. Becer, Dendritic Cell Lectin-Targeting Sentinel-like Unimolecular Glycoconjugates To Release an Anti-HIV Drug, J. Am. Chem. Soc., 2014, 136, 4325–4332, DOI:10.1021/ja4131565.
- G. Yilmaz, V. Uzunova, R. Napier and C. R. Becer, Single-Chain Glycopolymer Folding via Host–Guest Interactions and Its Unprecedented Effect on DC-SIGN Binding, Biomacromolecules, 2018, 19, 3040–3047, DOI:10.1021/acs.biomac.8b00600.
- J. Cramer, A. Lakkaichi, B. Aliu, R. P. Jakob, S. Klein, I. Cattaneo, X. Jiang, S. Rabbani, O. Schwardt, G. Zimmer, M. Ciancaglini, T. Abreu Mota, T. Maier and B. Ernst, Sweet Drugs for Bad Bugs: A Glycomimetic Strategy against the DC-SIGN-Mediated Dissemination of SARS-CoV-2, J. Am. Chem. Soc., 2021, 143, 17465–17478, DOI:10.1021/jacs.1c06778.
- J. Cramer, B. Aliu, X. Jiang, T. Sharpe, L. Pang, A. Hadorn, S. Rabbani and B. Ernst, Poly-L-lysine Glycoconjugates Inhibit DC-SIGN-mediated Attachment of Pandemic Viruses, ChemMedChem, 2021, 16, 2345–2353, DOI:10.1002/cmdc.202100348.
- M. Hartweg, Y. Jiang, G. Yilmaz, C. M. Jarvis, H. V.-T. Nguyen, G. A. Primo, A. Monaco, V. P. Beyer, K. K. Chen, S. Mohapatra, S. Axelrod, R. Gómez-Bombarelli, L. L. Kiessling, C. R. Becer and J. A. Johnson, Synthetic Glycomacromolecules of Defined Valency, Absolute Configuration, and Topology Distinguish between Human Lectins, JACS Au, 2021, 1, 1621–1630, DOI:10.1021/jacsau.1c00255.
- S. Zhou, P. Trochimczyk, L. Sun, S. Hou and H. Li, Sugar-Functionalized Fullerenes, Curr. Org. Chem., 2016, 20, 1490–1501, DOI:10.2174/1385272820666151207194235.
- E. Castro, A. H. Garcia, G. Zavala and L. Echegoyen, Fullerenes in biology and medicine, J. Mater. Chem. B, 2017, 5, 6523–6535, 10.1039/c7tb00855d.
- A. Muñoz, B. M. Illescas, J. Luczkowiak, F. Lasala, R. Ribeiro-Viana, J. Rojo, R. Delgado and N. Martín, Antiviral activity of self-assembled glycodendro[60]fullerene monoadducts, J. Mater. Chem. B, 2017, 5, 6566–6571, 10.1039/c7tb01379e.
- J. Ramos-Soriano, A. Pérez-Sánchez, S. Ramírez-Barroso, B. M. Illescas, K. Azmani, A. Rodríguez-Fortea, J. M. Poblet, C. Hally, S. Nonell, D. García-Fresnadillo, J. Rojo and N. Martín, An Ultra-Long-Lived Triplet Excited State in Water at Room Temperature: Insights on the Molecular Design of Tridecafullerenes, Angew. Chem., Int. Ed., 2021, 60, 16109–16118, DOI:10.1002/anie.202104223.
- B. M. Illescas, J. Rojo, R. Delgado and N. Martín, Multivalent Glycosylated Nanostructures To Inhibit Ebola Virus Infection, J. Am. Chem. Soc., 2017, 139, 6018–6025, DOI:10.1021/jacs.7b01683.
- J. Ramos-Soriano, M. Ghirardello and M. C. Galan, Recent Advances on Multivalent Carbon Nanoform-Based Glycoconjugates, Curr. Med. Chem., 2022, 29, 1232–1257, DOI:10.2174/0929867328666210714160954.
-
J. Ramos-Soriano, A. Pérez-Sánchez, B. M. Illescas, J. Rojo, R. Delgado and N. Martín, Carbon Nanostructures for Biomedical Applications, The Royal Society of Chemistry, 2021, pp. 56–97 10.1039/9781839161070-00056.
- J.-F. Nierengarten, J. Iehl, V. Oerthel, M. Holler, B. M. Illescas, A. Muñoz, N. Martín, J. Rojo, M. Sánchez-Navarro, S. Cecioni, S. Vidal, K. Buffet, M. Durka and S. P. Vincent, Fullerene sugar balls, Chem. Commun., 2010, 46, 3860–3862, 10.1039/c0cc00034e.
- J. Ramos-Soriano, J. J. Reina, A. Pérez-Sánchez, B. M. Illescas, J. Rojo and N. Martín, Cyclooctyne [60]fullerene hexakis adducts: a globular scaffold for copper-free click chemistry, Chem. Commun., 2016, 52, 10544–10546, 10.1039/c6cc05484f.
- J. Ramos-Soriano, J. J. Reina, B. M. Illescas, J. Rojo and N. Martín, Maleimide and Cyclooctyne-Based Hexakis-Adducts of Fullerene: Multivalent Scaffolds for Copper-Free Click Chemistry on Fullerenes, J. Org. Chem., 2018, 83, 1727–1736, DOI:10.1021/acs.joc.7b02402.
- J. Luczkowiak, A. Muñoz, M. Sánchez-Navarro, R. Ribeiro-Viana, A. Ginieis, B. M. Illescas, N. Martín, R. Delgado and J. Rojo, Glycofullerenes Inhibit Viral Infection, Biomacromolecules, 2013, 14, 431–437, DOI:10.1021/bm3016658.
- J. Ramos-Soriano, B. M. Illescas, A. Pérez-Sánchez, R. Sánchez-Bento, F. Lasala, J. Rojo, R. Delgado and N. Martín, Topological and Multivalent Effects in Glycofullerene Oligomers as EBOLA Virus Inhibitors, Int. J. Mol. Sci., 2022, 23, 5083 CrossRef CAS PubMed.
- A. Muñoz, D. Sigwalt, B. M. Illescas, J. Luczkowiak, L. Rodríguez-Pérez, I. Nierengarten, M. Holler, J.-S. Remy, K. Buffet, S. P. Vincent, J. Rojo, R. Delgado, J.-F. Nierengarten and N. Martín, Synthesis of giant globular multivalent glycofullerenes as potent inhibitors in a model of Ebola virus infection, Nat. Chem., 2016, 8, 50–57, DOI:10.1038/Nchem.2387.
- J. J. Reina, A. Di Maio, J. Ramos-Soriano, R. C. Figueiredo and J. Rojo, Rapid and efficient synthesis of α(1–2)mannobiosides, Org. Biomol. Chem., 2016, 14, 2873–2882, 10.1039/c6ob00083e.
- H. Feinberg, R. Castelli, K. Drickamer, P. H. Seeberger and W. I. Weis, Multiple Modes of Binding Enhance the Affinity of DC-SIGN for High Mannose N-Linked Glycans Found on Viral Glycoproteins, J. Biol. Chem., 2007, 282, 4202–4209, DOI:10.1074/jbc.M609689200.
- M. Thépaut, C. Guzzi, I. Sutkeviciute, S. Sattin, R. Ribeiro-Viana, N. Varga, E. Chabrol, J. Rojo, A. Bernardi, J. Angulo, P. M. Nieto and F. Fieschi, Structure of a Glycomimetic Ligand in the Carbohydrate Recognition Domain of C-type Lectin DC-SIGN. Structural Requirements for Selectivity and Ligand Design, J. Am. Chem. Soc., 2013, 135, 2518–2529, DOI:10.1021/ja3053305.
- L. Rodríguez-Pérez, J. Ramos-Soriano, A. Pérez-Sánchez, B. M. Illescas, A. Muñoz, J. Luczkowiak, F. Lasala, J. Rojo, R. Delgado and N. Martín, Nanocarbon-Based Glycoconjugates as Multivalent Inhibitors of Ebola Virus Infection, J. Am. Chem. Soc., 2018, 140, 9891–9898, DOI:10.1021/jacs.8b03847.
- F. Compostella, O. Pitirollo, A. Silvestri and L. Polito, Glyco-gold nanoparticles: synthesis and applications, Beilstein J. Org. Chem., 2017, 13, 1008–1021, DOI:10.3762/bjoc.13.100.
- D. A. Giljohann, D. S. Seferos, W. L. Daniel, M. D. Massich, P. C. Patel and C. A. Mirkin, Gold Nanoparticles for Biology and Medicine, Angew. Chem., Int. Ed., 2010, 49, 3280–3294, DOI:10.1002/anie.200904359.
- M. Marradi, M. Martín-Lomas and S. Penadés, Glyconanoparticles: Polyvalent Tools to Study Carbohydrate-Based Interactions, Adv. Carbohydr. Chem. Biochem., 2010, 64, 211–290, DOI:10.1016/S0065-2318(10)64005-X.
-
P. Di Gianvincenzo, F. Chiodo, M. Marradi and S. Penadés, in Methods Enzymol., ed. N. Düzgüneş, Academic Press, 2012, vol. 509, pp. 21–40 Search PubMed.
- F. Chiodo, M. Marradi, B. Tefsen, H. Snippe, I. van Die and S. Penadés, High Sensitive Detection of Carbohydrate Binding Proteins in an ELISA-Solid Phase Assay Based on Multivalent Glyconanoparticles, PLoS One, 2013, 8, e73027, DOI:10.1371/journal.pone.0073027.
- B. Arnáiz, O. Martínez-Ávila, J. M. Falcon-Perez and S. Penadés, Cellular Uptake of Gold Nanoparticles Bearing HIV gp120 Oligomannosides, Bioconjugate Chem., 2012, 23, 814–825, DOI:10.1021/bc200663r.
- O. Martínez-Ávila, L. M. Bedoya, M. Marradi, C. Clavel, J. Alcamí and S. Penadés, Multivalent Manno-Glyconanoparticles Inhibit DC-SIGN-Mediated HIV-1 Trans-Infection of Human T Cells, ChemBioChem, 2009, 10, 1806–1809, DOI:10.1002/cbic.200900294.
- D. Arosio, F. Chiodo, J. J. Reina, M. Marelli, S. Penadés, Y. van Kooyk, J. J. Garcia-Vallejo and A. Bernardi, Effective Targeting of DC-SIGN by α-Fucosylamide Functionalized Gold Nanoparticles, Bioconjugate Chem., 2014, 25, 2244–2251, DOI:10.1021/bc500467u.
- S. Toraskar, M. Gade, S. Sangabathuni, H. V. Thulasiram and R. Kikkeri, Exploring the Influence of Shapes and Heterogeneity of Glyco-Gold Nanoparticles on Bacterial Binding for Preventing Infections, ChemMedChem, 2017, 12, 1116–1124, DOI:10.1002/cmdc.201700218.
- X. Le Guével, M. Perez Perrino, T. D. Fernández, F. Palomares, M.-J. Torres, M. Blanca, J. Rojo and C. Mayorga, Multivalent Glycosylation of Fluorescent Gold Nanoclusters Promotes Increased Human Dendritic Cell Targeting via Multiple Endocytic Pathways, ACS Appl. Mater. Interfaces, 2015, 7, 20945–20956, DOI:10.1021/acsami.5b06541.
- D. Budhadev, E. Poole, I. Nehlmeier, Y. Liu, J. Hooper, E. Kalverda, U. S. Akshath, N. Hondow, W. B. Turnbull, S. Pöhlmann, Y. Guo and D. Zhou, Glycan-Gold Nanoparticles as Multifunctional Probes for Multivalent Lectin–Carbohydrate Binding: Implications for Blocking Virus Infection and Nanoparticle Assembly, J. Am. Chem. Soc., 2020, 142, 18022–18034, DOI:10.1021/jacs.0c06793.
- Y. Guo, H. Feinberg, E. Conroy, D. A. Mitchell, R. Alvarez, O. Blixt, M. E. Taylor, W. I. Weis and K. Drickamer, Structural basis for distinct ligand-binding and targeting properties of the receptors DC-SIGN and DC-SIGNR, Nat. Struct. Mol. Biol., 2004, 11, 591–598, DOI:10.1038/nsmb784.
- S. Pöhlmann, E. J. Soilleux, F. Baribaud, G. J. Leslie, L. S. Morris, J. Trowsdale, B. Lee, N. Coleman and R. W. Doms, DC-SIGNR, a DC-SIGN homologue expressed in endothelial cells, binds to human and simian immunodeficiency viruses and activates infection in trans, Proc. Natl. Acad. Sci. U. S. A., 2001, 98, 2670–2675, DOI:10.1073/pnas.051631398.
- E. J. Soilleux, DC-SIGN (dendritic cell-specific ICAM-grabbing non-integrin) and DC-SIGN-related (DC-SIGNR): friend or foe, Clin. Sci., 2003, 104, 437–446, DOI:10.1042/cs1040437.
- H. Feinberg, C. K. W. Tso, M. E. Taylor, K. Drickamer and W. I. Weis, Segmented Helical Structure of the Neck Region of the Glycan-Binding Receptor DC-SIGNR, J. Mol. Biol., 2009, 394, 613–620, DOI:10.1016/j.jmb.2009.10.006.
- C. W. Davis, H.-Y. Nguyen, S. L. Hanna, M. D. Sánchez, R. W. Doms and T. C. Pierson, West Nile Virus Discriminates between DC-SIGN and DC-SIGNR for Cellular Attachment and Infection, J. Virol., 2006, 80, 1290–1301, DOI:10.1128/JVI.80.3.1290-1301.2006.
- S. Pöhlmann, G. J. Leslie, T. G. Edwards, T. Macfarlan, J. D. Reeves, K. Hiebenthal-Millow, F. Kirchhoff, F. Baribaud and R. W. Doms, DC-SIGN Interactions with Human Immunodeficiency Virus: Virus Binding and Transfer Are Dissociable Functions, J. Virol., 2001, 75, 10523–10526, DOI:10.1128/JVI.75.21.10523-10526.2001.
- N. P. Y. Chung, S. K. J. Breun, A. Bashirova, J. G. Baumann, T. D. Martin, J. M. Karamchandani, J. W. Rausch, S. F. J. Le Grice, L. Wu, M. Carrington and V. N. KewalRamani, HIV-1 Transmission by Dendritic Cell-specific ICAM-3-grabbing Nonintegrin (DC-SIGN) Is Regulated by Determinants in the Carbohydrate Recognition Domain That Are Absent in Liver/Lymph Node-SIGN (L-SIGN), J. Biol. Chem., 2010, 285, 2100–2112, DOI:10.1074/jbc.M109.030619.
- Y. Guo, I. Nehlmeier, E. Poole, C. Sakonsinsiri, N. Hondow, A. Brown, Q. Li, S. Li, J. Whitworth, Z. Li, A. Yu, R. Brydson, W. B. Turnbull, S. Pöhlmann and D. Zhou, Dissecting Multivalent Lectin–Carbohydrate Recognition Using Polyvalent Multifunctional Glycan-Quantum Dots, J. Am. Chem. Soc., 2017, 139, 11833–11844, DOI:10.1021/jacs.7b05104.
- Y. Guo, C. Sakonsinsiri, I. Nehlmeier, M. A. Fascione, H. Zhang, W. Wang, S. Pöhlmann, W. B. Turnbull and D. Zhou, Compact, Polyvalent Mannose Quantum Dots as Sensitive, Ratiometric FRET Probes for Multivalent Protein-Ligand Interactions, Angew. Chem., Int. Ed., 2016, 55, 4738–4742, DOI:10.1002/anie.201600593.
-
J. Hooper, Y. Guo and D. Zhou, Glycan-Based Cellular Communication: Techniques for Carbohydrate-Protein Interactions, American Chemical Society, 2020, vol. 1346, ch. 4, pp. 47–66 Search PubMed.
- J. Hooper, Y. Liu, D. Budhadev, D. Fernandez Ainaga, N. Hondow, D. Zhou and Y. Guo, Polyvalent Glycan Quantum Dots as a Multifunctional Tool for Revealing Thermodynamic, Kinetic, and Structural Details of Multivalent Lectin–Glycan Interactions, ACS Appl. Mater. Interfaces, 2022, 14, 47385–47396, DOI:10.1021/acsami.2c11111.
- S. H. Rouhanifard, R. Xie, G. Zhang, X. Sun, X. Chen and P. Wu, Detection and Isolation of Dendritic Cells Using Lewis X-Functionalized Magnetic Nanoparticles, Biomacromolecules, 2012, 13, 3039–3045, DOI:10.1021/bm3007506.
- S. Park, G.-H. Kim, S.-H. Park, J. Pai, D. Rathwell, J.-Y. Park, Y.-S. Kang and I. Shin, Probing Cell-Surface Carbohydrate Binding Proteins with Dual-Modal Glycan-Conjugated Nanoparticles, J. Am. Chem. Soc., 2015, 137, 5961–5968, DOI:10.1021/jacs.5b00592.
- H. Bavireddi, V. R. Murthy, M. Gade, S. Sangabathuni, P. M. Chaudhary, C. Alex, B. Lepenies and R. Kikkeri, Understanding carbohydrate–protein interactions using homologous supramolecular chiral Ru(II)-glyconanoclusters, Nanoscale, 2016, 8, 19696–19702, 10.1039/C6NR06431K.
- M. M. Alam, C. M. Jarvis, R. Hincapie, C. S. McKay, J. Schimer, C. A. Sanhueza, K. Xu, R. C. Diehl, M. G. Finn and L. L. Kiessling, Glycan-Modified Virus-like Particles Evoke T Helper Type 1-like Immune Responses, ACS Nano, 2021, 15, 309–321, DOI:10.1021/acsnano.0c03023.
- R. Wawrzinek, E.-C. Wamhoff, J. Lefebre, M. Rentzsch, G. Bachem, G. Domeniconi, J. Schulze, F. F. Fuchsberger, H. Zhang, C. Modenutti, L. Schnirch, M. A. Marti, O. Schwardt, M. Bräutigam, M. Guberman, D. Hauck, P. H. Seeberger, O. Seitz, A. Titz, B. Ernst and C. Rademacher, A Remote Secondary Binding Pocket Promotes Heteromultivalent Targeting of DC-SIGN, J. Am. Chem. Soc., 2021, 143, 18977–18988, DOI:10.1021/jacs.1c07235.
- T. Ito, M. Inaba, K. Inaba, J. Toki, S. Sogo, T. Iguchi, Y. Adachi, K. Yamaguchi, R. Amakawa, J. Valladeau, S. Saeland, S. Fukuhara and S. Ikehara, A CD1a+/CD11c+ Subset of Human Blood Dendritic Cells Is a Direct Precursor of Langerhans Cells, J. Immunol., 1999, 163, 1409–1419 CAS.
- N. S. Stambach and M. E. Taylor, Characterization of carbohydrate recognition by langerin, a C-type lectin of Langerhans cells, Glycobiology, 2003, 13, 401–410, DOI:10.1093/glycob/cwg045.
- H. Feinberg, A. S. Powlesland, M. E. Taylor and W. I. Weis, Trimeric Structure of Langerin, J. Biol. Chem., 2010, 285, 13285–13293, DOI:10.1074/jbc.M109.086058.
- H. Feinberg, M. E. Taylor, N. Razi, R. McBride, Y. A. Knirel, S. A. Graham, K. Drickamer and W. I. Weis, Structural Basis for Langerin Recognition of Diverse Pathogen and Mammalian Glycans through a Single Binding Site, J. Mol. Biol., 2011, 405, 1027–1039, DOI:10.1016/j.jmb.2010.11.039.
- E. Chabrol, A. Nurisso, A. Daina, E. Vassal-Stermann, M. Thepaut, E. Girard, R. R. Vivès and F. Fieschi, Glycosaminoglycans Are Interactants of Langerin: Comparison with gp120 Highlights an Unexpected Calcium-Independent Binding Mode, PLoS One, 2012, 7, e50722, DOI:10.1371/journal.pone.0050722.
- J. C. Muñoz-García, E. Chabrol, R. R. Vivès, A. Thomas, J. L. de Paz, J. Rojo, A. Imberty, F. Fieschi, P. M. Nieto and J. Angulo, Langerin–Heparin Interaction: Two Binding Sites for Small and Large Ligands As Revealed by a Combination of NMR Spectroscopy and Cross-Linking Mapping Experiments, J. Am. Chem. Soc., 2015, 137, 4100–4110, DOI:10.1021/ja511529x.
- J. Hanske, R. Wawrzinek, A. Geissner, E.-C. Wamhoff, K. Sellrie, H. Schmidt, P. H. Seeberger and C. Rademacher, Calcium-Independent Activation of an Allosteric Network in Langerin by Heparin Oligosaccharides, ChemBioChem, 2017, 18, 1183–1187, DOI:10.1002/cbic.201700027.
- R. E. Hunger, P. A. Sieling, M. T. Ochoa, M. Sugaya, A. E. Burdick, T. H. Rea, P. J. Brennan, J. T. Belisle, A. Blauvelt, S. A. Porcelli and R. L. Modlin, Langerhans cells utilize CD1a and langerin to efficiently present nonpeptide antigens to T cells, J. Clin. Invest., 2004, 113, 701–708, DOI:10.1172/JCI19655.
- M. A. W. P. de Jong, L. E. M. Vriend, B. Theelen, M. E. Taylor, D. Fluitsma, T. Boekhout and T. B. H. Geijtenbeek, C-type lectin Langerin is a β-glucan receptor on human Langerhans cells that recognizes opportunistic and pathogenic fungi, Mol. Immunol., 2010, 47, 1216–1225, DOI:10.1016/j.molimm.2009.12.016.
- H. Tateno, K. Ohnishi, R. Yabe, N. Hayatsu, T. Sato, M. Takeya, H. Narimatsu and J. Hirabayashi, Dual Specificity of Langerin to Sulfated and Mannosylated Glycans via a Single C-type Carbohydrate Recognition Domain, J. Biol. Chem., 2010, 285, 6390–6400, DOI:10.1074/jbc.M109.041863.
- N. Nasr, J. Lai, R. A. Botting, S. K. Mercier, A. N. Harman, M. Kim, S. Turville, R. J. Center, T. Domagala, P. R. Gorry, N. Olbourne and A. L. Cunningham, Inhibition of Two Temporal Phases of HIV-1 Transfer from Primary Langerhans Cells to T Cells: The Role of Langerin, J. Immunol., 2014, 193, 2554–2564, DOI:10.4049/jimmunol.1400630.
- P. Domínguez-Rodríguez, C. Vivès, M. Thepaut, F. Fieschi, P. M. Nieto, J. L. de Paz and J. Rojo, Second-Generation Dendrimers with Chondroitin Sulfate Type-E Disaccharides as Multivalent Ligands for Langerin, Biomacromolecules, 2020, 21, 2726–2734, DOI:10.1021/acs.biomac.0c00476.
- F. Ota, T. Hirayama, Y. Kizuka, Y. Yamaguchi, R. Fujinawa, M. Nagata, H. S. Ismanto, B. Lepenies, J. Aretz, C. Rademacher, P. H. Seeberger, T. Angata, S. Kitazume, K. Yoshida, T. Betsuyaku, K. Kida, S. Yamasaki and N. Taniguchi, High affinity sugar ligands of C-type lectin receptor langerin, Biochim. Biophys. Acta, Gen. Subj., 2018, 1862, 1592–1601, DOI:10.1016/j.bbagen.2018.04.004.
- J. Rojo, P. M. Nieto and J. L. de Paz, GAG Multivalent Systems to Interact with Langerin, Curr. Med. Chem., 2022, 29, 1173–1192, DOI:10.2174/0929867328666210705143102.
- P. Domínguez-Rodríguez, J. J. Reina, S. Gil-Caballero, P. M. Nieto, J. L. de Paz and J. Rojo, Glycodendrimers as Chondroitin Sulfate Mimetics: Synthesis and Binding to Growth Factor Midkine, Chem. – Eur. J., 2017, 23, 11338–11345, DOI:10.1002/chem.201701890.
- K. Neuhaus, E.-C. Wamhoff, T. Freichel, A. Grafmüller, C. Rademacher and L. Hartmann, Asymmetrically Branched Precision Glycooligomers Targeting Langerin, Biomacromolecules, 2019, 20, 4088–4095, DOI:10.1021/acs.biomac.9b00906.
- C. Fasting, C. A. Schalley, M. Weber, O. Seitz, S. Hecht, B. Koksch, J. Dernedde, C. Graf, E.-W. Knapp and R. Haag, Multivalency as a Chemical Organization and Action Principle, Angew. Chem., Int. Ed., 2012, 51, 10472–10498, DOI:10.1002/anie.201201114.
- S. Mayer, M.-K. Raulf and B. Lepenies, C-type lectins: their network and roles in pathogen recognition and immunity, Histochem. Cell Biol., 2017, 147, 223–237, DOI:10.1007/s00418-016-1523-7.
- R. C. Preston, S. Rabbani, F. P. C. Binder, S. Moes, J. L. Magnani and B. Ernst, Implications of the E-selectin S128R mutation for drug discovery, Glycobiology, 2014, 24, 592–601, DOI:10.1093/glycob/cwu026.
- S. Bhatia, M. Dimde and R. Haag, Multivalent glycoconjugates as vaccines and potential drug candidates, MedChemComm, 2014, 5, 862–878, 10.1039/C4MD00143E.
- A. Restuccia, M. M. Fettis and G. A. Hudalla, Glycomaterials for immunomodulation, immunotherapy,
and infection prophylaxis, J. Mater. Chem. B, 2016, 4, 1569–1585, 10.1039/c5tb01780g.
- F. J. Barkalow, K. L. Barkalow and T. N. Mayadas, Dimerization of P-selectin in platelets and endothelial cells, Blood, 2000, 96, 3070–3077, DOI:10.1182/blood.V96.9.3070.
- E. E. Simanek, G. J. McGarvey, J. A. Jablonowski and C.-H. Wong, Selectin-Carbohydrate Interactions: From Natural Ligands to Designed Mimics, Chem. Rev., 1998, 98, 833–862, DOI:10.1021/cr940226i.
- L. L. Kiessling and J. C. Grim, Glycopolymer probes of signal transduction, Chem. Soc. Rev., 2013, 42, 4476–4491, 10.1039/c3cs60097a.
- J. Salta, J. Dernedde and H.-U. Reissig, Synthesis of multivalent carbohydrate mimetics with aminopolyol end groups and their evaluation as L-selectin inhibitors, Beilstein J. Org. Chem., 2015, 11, 638–646, DOI:10.3762/bjoc.11.72.
- J. Salta and H.-U. Reissig, Divalent Triazole-Linked Carbohydrate Mimetics: Synthesis by Click Chemistry and Evaluation as Selectin Ligands, Eur. J. Org. Chem., 2020, 4361–4370, DOI:10.1002/ejoc.202000618.
- J. Salta, F. F. Arp, C. Kühne and H.-U. Reissig, Multivalent 1,2,3-Triazole-Linked Carbohydrate Mimetics by Huisgen–Meldal-Sharpless Cycloadditions of an Azidopyran, Eur. J. Org. Chem., 2020, 7333–7342, DOI:10.1002/ejoc.202001389.
- I. Tavernaro, A. M. Rodrigo, M. Kandziora, S. Kuntz, J. Dernedde, C. Trautwein, F. Tacke, A. Blas-Garcia and M. Bartneck, Modulating Myeloid Immune Cell Migration Using Multivalently Presented Monosaccharide Ligands for Advanced Immunotherapy, Adv. Theory, 2019, 2, 1900145, DOI:10.1002/adtp.201900145.
- C. Scheibe, S. Wedepohl, S. B. Riese, J. Dernedde and O. Seitz, Carbohydrate–PNA and Aptamer–PNA Conjugates for the Spatial Screening of Lectins and Lectin Assemblies, ChemBioChem, 2013, 14, 236–250, DOI:10.1002/cbic.201200618.
- C. Chantarasrivong, A. Ueki, R. Ohyama, J. Unga, S. Nakamura, I. Nakanishi, Y. Higuchi, S. Kawakami, H. Ando, A. Imamura, H. Ishida, F. Yamashita, M. Kiso and M. Hashida, Synthesis and Functional Characterization of Novel Sialyl LewisX Mimic-Decorated Liposomes for E-selectin-Mediated Targeting to Inflamed Endothelial Cells, Mol. Pharmaceutics, 2017, 14, 1528–1537, DOI:10.1021/acs.molpharmaceut.6b00982.
- A. Alekseeva, M. Kapkaeva, O. Shcheglovitova, I. Boldyrev, G. Pazynina, N. Bovin and E. Vodovozova, Interactions of antitumour Sialyl Lewis X liposomes with vascular endothelial cells, Biochim. Biophys. Acta, Biomembr., 2015, 1848, 1099–1110, DOI:10.1016/j.bbamem.2015.01.016.
- E. Jubeli, L. Moine, V. Nicolas and G. Barratt, Preparation of E-selectin-targeting nanoparticles and preliminary in vitro evaluation, Int. J. Pharm., 2012, 426, 291–301, DOI:10.1016/j.ijpharm.2012.01.029.
- K. E. Moog, M. Barz, M. Bartneck, F. Beceren-Braun, N. Mohr, Z. Wu, L. Braun, J. Dernedde, E. A. Liehn, F. Tacke, T. Lammers, H. Kunz and R. Zentel, Polymeric Selectin Ligands Mimicking Complex Carbohydrates: From Selectin Binders to Modifiers of Macrophage Migration, Angew. Chem., Int. Ed., 2017, 56, 1416–1421, DOI:10.1002/anie.201610395.
- M. Bartneck, C. T. Schlößer, M. Barz, R. Zentel, C. Trautwein, T. Lammers and F. Tacke, Immunomodulatory Therapy of Inflammatory Liver Disease Using Selectin-Binding Glycopolymers, ACS Nano, 2017, 11, 9689–9700, DOI:10.1021/acsnano.7b04630.
- G. A. Rabinovich, M. A. Toscano, S. S. Jackson and G. R. Vasta, Functions of cell surface galectin-glycoprotein lattices, Curr. Opin. Struct. Biol., 2007, 17, 513–520, DOI:10.1016/j.sbi.2007.09.002.
-
R. D. Cummings, F.-T. Liu, G. A. Rabinovich, S. R. Stowell and G. R. Vasta, in Essentials of Glycobiology, ed. A. Varki, R. D. Cummings, J. D. Esko, P. Stanley, G. W. Hart, M. Aebi, D. Mohnen, T. Kinoshita, N. H. Packer, J. J. Prestegard, R. L. Schnaar and P. H. Seeberger, Cold Spring Harbor, New York, 2022 Search PubMed.
- D. Laaf, P. Bojarová, L. Elling and V. Křen, Galectin–Carbohydrate Interactions in Biomedicine and Biotechnology, Trends Biotechnol., 2019, 37, 402–415, DOI:10.1016/j.tibtech.2018.10.001.
- P. Nangia-Makker, T. Raz, L. Tait, V. Hogan, R. Fridman and A. Raz, Galectin-3 Cleavage: A Novel Surrogate Marker for Matrix Metalloproteinase Activity in Growing Breast Cancers, Cancer Res., 2007, 67, 11760–11768, DOI:10.1158/0008-5472.CAN-07-3233.
- S. H. Barondes, V. Castronovo, D. N. W. Cooper, R. D. Cummings, K. Drickamer, T. Felzi, M. A. Gitt, J. Hirabayashi, C. Hughes, K.-I. Kasai, H. Leffler, F.-T. Liu, R. Lotan, A. M. Mercurio, M. Monsigny, S. Pillai, F. Poirer, A. Raz, P. W. J. Rigby, J. M. Rini and J. L. Wang, Galectins: A family of animal β-galactoside-binding lectins, Cell, 1994, 76, 597–598, DOI:10.1016/0092-8674(94)90498-7.
- S. H. Barondes, D. N. W. Cooper, M. A. Gitt and H. Leffler, Galectins. Structure and function of a large family of animal lectins, J. Biol. Chem., 1994, 269, 20807–20810 CrossRef CAS PubMed.
- J. M. Cousin and M. J. Cloninger, The Role of Galectin-1 in Cancer Progression, and Synthetic Multivalent Systems for the Study of Galectin-1, Int. J. Mol. Sci., 2016, 17, 1566, DOI:10.3390/ijms17091566.
- L. Astorgues-Xerri, M. E. Riveiro, A. Tijeras-Raballand, M. Serova, C. Neuzillet, S. Albert, E. Raymond and S. Faivre, Unraveling galectin-1 as a novel therapeutic target for cancer, Cancer Treat. Rev., 2014, 40, 307–319, DOI:10.1016/j.ctrv.2013.07.007.
- I. Camby, M. Le Mercier, F. Lefranc and R. Kiss, Galectin-1: a small protein with major functions, Glycobiology, 2006, 16, 137R–157R, DOI:10.1093/glycob/cwl025.
- F.-T. Liu and G. A. Rabinovich, Galectins as modulators of tumour progression, Nat. Rev. Cancer, 2005, 5, 29–41, DOI:10.1038/nrc1527.
- G. A. Rabinovich, Galectin-1 as a potential cancer target, Br. J. Cancer, 2005, 92, 1188–1192, DOI:10.1038/sj.bjc.6602493.
- S. André, K. E. Kövér, H.-J. Gabius and L. Szilágyi, Thio- and selenoglycosides as ligands for biomedically relevant lectins: Valency–activity correlations for benzene-based dithiogalactoside clusters and first assessment for (di)selenodigalactosides, Bioorg. Med. Chem. Lett., 2015, 25, 931–935, DOI:10.1016/j.bmcl.2014.12.049.
- J. Mackeviča, P. Ostrovskis, H. Leffler, U. J. Nilsson, V. Rudovica, A. Viksna, S. Belyakov and M. Turks, Synthesis of 1,2,3-triazolelinked galactohybrids and their inhibitory activities on galectins, ARKIVOC, 2014, 2014, 90–112, DOI:10.3998/ark.5550190.p008.402.
- S. Mercier, C. St-Pierre, I. Pelletier, M. Ouellet, M. J. Tremblay and S. Sato, Galectin-1 promotes HIV-1 infectivity in macrophages through stabilization of viral adsorption, Virology, 2008, 371, 121–129, DOI:10.1016/j.virol.2007.09.034.
- M. Ouellet, S. Mercier, I. Pelletier, S. Bounou, J. Roy, J. Hirabayashi, S. Sato and M. J. Tremblay, Galectin-1 Acts as a Soluble Host Factor That
Promotes HIV-1 Infectivity through Stabilization of Virus Attachment to Host Cells, J. Immunol., 2005, 174, 4120–4126, DOI:10.4049/jimmunol.174.7.4120.
- C. St-Pierre, H. Manya, M. Ouellet, G. F. Clark, T. Endo, M. J. Tremblay and S. Sato, Host-Soluble Galectin-1 Promotes HIV-1 Replication through a Direct Interaction with Glycans of Viral gp120 and Host CD4, J. Virol., 2011, 85, 11742–11751, DOI:10.1128/JVI.05351-11.
- C. St-Pierre, M. Ouellet, D. Giguère, R. Ohtake, R. Roy, S. Sato and M. J. Tremblay, Galectin-1-Specific Inhibitors as a New Class of Compounds To Treat HIV-1 Infection, Antimicrob. Agents Chemother., 2012, 56, 154–162, DOI:10.1128/AAC.05595-11.
- S. Cecioni, S. E. Matthews, H. Blanchard, J.-P. Praly, A. Imberty and S. Vidal, Synthesis of lactosylated glycoclusters and inhibition studies with plant and human lectins, Carbohydr. Res., 2012, 356, 132–141, DOI:10.1016/j.carres.2012.02.006.
- S. Velasco, N. Díez-Revuelta, T. Hernández-Iglesias, H. Kaltner, S. André, H.-J. Gabius and J. Abad-Rodríguez, Neuronal Galectin-4 is required for axon growth and for the organization of axonal membrane L1 delivery and clustering, J. Neurochem., 2013, 125, 49–62, DOI:10.1111/jnc.12148.
- S. André, G.-N. Wang, H.-J. Gabius and P. V. Murphy, Combining glycocluster synthesis with protein engineering: an approach to probe into the significance of linker length in a tandem-repeat-type lectin (galectin-4), Carbohydr. Res., 2014, 389, 25–38, DOI:10.1016/j.carres.2013.12.024.
- G.-N. Wang, S. André, H.-J. Gabius and P. V. Murphy, Bi- to tetravalent glycoclusters: synthesis, structure–activity profiles as lectin inhibitors and impact of combining both valency and headgroup tailoring on selectivity, Org. Biomol. Chem., 2012, 10, 6893–6907, 10.1039/c2ob25870f.
- R. Roy, Y. Cao, H. Kaltner, N. Kottari, T. C. Shiao, K. Belkhadem, S. André, J. C. Manning, P. V. Murphy and H.-J. Gabius, Teaming up synthetic chemistry and histochemistry for activity screening in galectin-directed inhibitor design, Histochem. Cell Biol., 2017, 147, 285–301, DOI:10.1007/s00418-016-1525-5.
- S. Ramaswamy, M. H. Sleiman, G. Masuyer, C. Arbez-Gindre, M. Micha-Screttas, T. Calogeropoulou, B. R. Steele and K. R. Acharya, Structural basis of multivalent galactose-based dendrimer recognition by human galectin-7, FEBS J., 2015, 282, 372–387, DOI:10.1111/febs.13140.
- V. L. Campo, M. F. Marchiori, L. C. Rodrigues and M. Dias-Baruffi, Synthetic glycoconjugates inhibitors of tumor-related galectin-3: an update, Glycoconjugate J., 2016, 33, 853–876, DOI:10.1007/s10719-016-9721-z.
- W. Jia, H. Kidoya, D. Yamakawa, H. Naito and N. Takakura, Galectin-3 Accelerates M2 Macrophage Infiltration and Angiogenesis in Tumors, Am. J. Pathol., 2013, 182, 1821–1831, DOI:10.1016/j.ajpath.2013.01.017.
- J. J. L. P. Voss, C. A. Ford, S. Petrova, L. Melville, M. Paterson, J. D. Pound, P. Holland, B. Giotti, T. C. Freeman and C. D. Gregory, Modulation of macrophage antitumor potential by apoptotic lymphoma cells, Cell Death Differ., 2017, 24, 971–983, DOI:10.1038/cdd.2016.132.
- A. C. MacKinnon, S. L. Farnworth, P. S. Hodkinson, N. C. Henderson, K. M. Atkinson, H. Leffler, U. J. Nilsson, C. Haslett, S. J. Forbes and T. Sethi, Regulation of Alternative Macrophage Activation by Galectin-3, J. Immunol., 2008, 180, 2650–2658, DOI:10.4049/jimmunol.180.4.2650.
- W. Peng, H. Y. Wang, Y. Miyahara, G. Peng and R.-F. Wang, Tumor-Associated Galectin-3 Modulates the Function of Tumor-Reactive T Cells, Cancer Res., 2008, 68, 7228–7236, DOI:10.1158/0008-5472.CAN-08-1245.
- Y. Honjo, P. Nangia-Makker, H. Inohara and A. Raz, Down-Regulation of Galectin-3 Suppresses Tumorigenicity of Human Breast Carcinoma Cells, Clin. Cancer Res., 2001, 7, 661–668 CAS.
- H. Ahmed and D. M. M. AlSadek, Galectin-3 as a Potential Target to Prevent Cancer Metastasis, Clin. Med. Insights: Oncol., 2015, 9, 113–121, DOI:10.4137/CMO.S29462.
- L. O'Driscoll, R. Linehan, Y. H. Liang, H. Joyce, I. Oglesby and M. Clynes, Galectin-3 expression alters adhesion, motility and invasion in a lung cell line (DLKP), in vitro, Anticancer Res., 2002, 22, 3117–3125 Search PubMed.
- N. Ahmad, H.-J. Gabius, S. André, H. Kaltner, S. Sabesan, R. Roy, B. Liu, F. Macaluso and C. F. Brewer, Galectin-3 Precipitates as a Pentamer with Synthetic Multivalent Carbohydrates and Forms Heterogeneous Cross-linked Complexes, J. Biol. Chem., 2004, 279, 10841–10847, DOI:10.1074/jbc.M312834200.
- J. Nieminen, A. Kuno, J. Hirabayashi and S. Sato, Visualization of Galectin-3 Oligomerization on the Surface of Neutrophils and Endothelial Cells Using Fluorescence Resonance Energy Transfer, J. Biol. Chem., 2007, 282, 1374–1383, DOI:10.1074/jbc.M604506200.
- J. Ochieng, B. Green, S. Evans, O. James and P. Warfield, Modulation of the biological functions of galectin-3 by matrix metalloproteinases, Biochim. Biophys. Acta, Gen. Subj., 1998, 1379, 97–106, DOI:10.1016/s0304-4165(97)00086-x.
- S. André, P. J. Cejas Ortega, M. Alamino Perez, R. Roy and H.-J. Gabius, Lactose-containing starburst dendrimers: influence of dendrimer generation and binding-site orientation of receptors (plant/animal lectins and immunoglobulins) on binding properties, Glycobiology, 1999, 9, 1253–1261, DOI:10.1093/glycob/9.11.1253.
- A. K. Michel, P. Nangia-Makker, A. Raz and M. J. Cloninger, Lactose-Functionalized Dendrimers Arbitrate the Interaction of Galectin-3/MUC1 Mediated Cancer Cellular Aggregation, ChemBioChem, 2014, 15, 2106–2112, DOI:10.1002/cbic.201402134.
- J. H. Ennist, H. R. Termuehlen, S. P. Bernhard, M. S. Fricke and M. J. Cloninger, Chemoenzymatic Synthesis of Galectin Binding Glycopolymers, Bioconjugate Chem., 2018, 29, 4030–4039, DOI:10.1021/acs.bioconjchem.8b00599.
- S. P. Bernhard, M. S. Fricke, R. Haag and M. J. Cloninger, Protein aggregation nucleated by functionalized dendritic polyglycerols, Polym. Chem., 2020, 11, 3849–3862, 10.1039/d0py00667j.
- C. K. Goodman, M. L. Wolfenden, P. Nangia-Makker, A. K. Michel, A. Raz and M. J. Cloninger, Multivalent scaffolds induce galectin-3 aggregation into nanoparticles, Beilstein J. Org. Chem., 2014, 10, 1570–1577, DOI:10.3762/bjoc.10.162.
- J. M. Cousin and M. J. Cloninger, Glycodendrimers: tools to explore multivalent galectin-1 interactions, Beilstein J. Org. Chem., 2015, 11, 739–747, DOI:10.3762/bjoc.11.84.
- M. Wolfenden, J. Cousin, P. Nangia-Makker, A. Raz and M. Cloninger, Glycodendrimers and Modified ELISAs: Tools to Elucidate Multivalent Interactions of Galectins 1 and 3, Molecules, 2015, 20, 7059–7096, DOI:10.3390/molecules20047059.
- M. Gade, C. Alex, S. Leviatan Ben-Arye, J. T. Monteiro, S. Yehuda, B. Lepenies, V. Padler-Karavani and R. Kikkeri, Microarray Analysis of Oligosaccharide-Mediated Multivalent Carbohydrate–Protein Interactions and Their Heterogeneity, ChemBioChem, 2018, 19, 1170–1177, DOI:10.1002/cbic.201800037.
- L. Abbassi, Y. M. Chabre, N. Kottari, A. A. Arnold, S. André, J. Josserand, H.-J. Gabius and R. Roy, Multifaceted glycodendrimers with programmable bioactivity through convergent, divergent, and accelerated approaches using polyfunctional cyclotriphosphazenes, Polym. Chem., 2015, 6, 7666–7683, 10.1039/C5PY01283J.
- P. Bojarová, M. R. Tavares, D. Laaf, L. Bumba, L. Petrásková, R. Konefał, M. Bláhová, H. Pelantová, L. Elling, T. Etrych, P. Chytil and V. Křen, Biocompatible glyconanomaterials based on HPMA-copolymer for specific targeting of galectin-3, J. Nanobiotechnol., 2018, 16, 73, DOI:10.1186/s12951-018-0399-1.
- C. Zhou, H. L. Reesink and D. A. Putnam, Selective and Tunable Galectin Binding of Glycopolymers Synthesized by a Generalizable Conjugation Method, Biomacromolecules, 2019, 20, 3704–3712, DOI:10.1021/acs.biomac.9b00759.
- J. Mauris, F. Mantelli, A. M. Woodward, Z. Cao, C. R. Bertozzi, N. Panjwani, K. Godula and P. Argüeso, Modulation of Ocular Surface Glycocalyx Barrier Function by a Galectin-3 N-terminal Deletion Mutant and Membrane-Anchored Synthetic Glycopolymers, PLoS One, 2013, 8, e72304, DOI:10.1371/journal.pone.0072304.
- S. Rosencrantz, J. S. J. Tang, C. Schulte-Osseili, A. Böker and R. R. Rosencrantz, Glycopolymers by RAFT Polymerization as Functional Surfaces for Galectin-3, Macromol. Chem. Phys., 2019, 220, 1900293, DOI:10.1002/macp.201900293.
- K. Ulbrich and V. Šubr, Structural and chemical aspects of HPMA copolymers as drug carriers, Adv. Drug Delivery Rev., 2010, 62, 150–166, DOI:10.1016/j.addr.2009.10.007.
- M. R. Tavares, M. Bláhová, L. Sedláková, L. Elling, H. Pelantová, R. Konefał, T. Etrych, V. Křen, P. Bojarová and P. Chytil, High-Affinity N-(2-Hydroxypropyl)methacrylamide Copolymers with Tailored N-Acetyllactosamine Presentation Discriminate between Galectins, Biomacromolecules, 2020, 21, 641–652, DOI:10.1021/acs.biomac.9b01370.
- M. Filipová, P. Bojarová, M. Rodriguez Tavares, L. Bumba, L. Elling, P. Chytil, K. Gunár, V. Křen, T. Etrych and O. Janoušková, Glycopolymers for Efficient Inhibition of Galectin-3: In Vitro Proof of Efficacy Using Suppression of T Lymphocyte Apoptosis and Tumor Cell Migration, Biomacromolecules, 2020, 21, 3122–3133, DOI:10.1021/acs.biomac.0c00515.
- S. Bertuzzi, A. Gimeno, A. Martinez-Castillo, M. G. Lete, S. Delgado, C. Airoldi, M. Rodrigues Tavares, M. Bláhová, P. Chytil, V. Křen, N. G. A. Abrescia, A. Ardá, P. Bojarová and J. Jiménez-Barbero, Cross-Linking Effects Dictate the Preference of Galectins to Bind LacNAc-Decorated HPMA Copolymers, Int. J. Mol. Sci., 2021, 22, 6000, DOI:10.3390/ijms22116000.
- A. J. Cagnoni, J. Kovensky and M. L. Uhrig, Design and Synthesis of Hydrolytically Stable Multivalent Ligands Bearing Thiodigalactoside Analogues for Peanut Lectin and Human Galectin-3 Binding, J. Org. Chem., 2014, 79, 6456–6467, DOI:10.1021/jo500883v.
- D. Vrbata, M. Filipová, M. R. Tavares, J. Červený, M. Vlachová, M. Šírová, H. Pelantová, L. Petrásková, L. Bumba, R. Konefał, T. Etrych, V. Křen, P. Chytil and P. Bojarová, Glycopolymers Decorated with 3-O-Substituted Thiodigalactosides as Potent Multivalent Inhibitors of Galectin-3, J. Med. Chem., 2022, 65, 3866–3878, DOI:10.1021/acs.jmedchem.1c01625.
- O. Suzuki and M. Abe, Galectin-1-mediated cell adhesion, invasion and cell death in human anaplastic large cell lymphoma: Regulatory roles of cell surface glycans, Int. J. Oncol., 2014, 44, 1433–1442, DOI:10.3892/ijo.2014.2319.
- N. D'Haene, S. Sauvage, C. Maris, I. Adanja, M. Le Mercier, C. Decaestecker, L. Baum and I. Salmon, VEGFR1 and VEGFR2 Involvement in Extracellular Galectin-1- and Galectin-3-Induced Angiogenesis, PLoS One, 2013, 8, e67029, DOI:10.1371/journal.pone.0067029.
- Y. C. Lee and R. T. Lee, Carbohydrate-Protein Interactions: Basis of Glycobiology, Acc. Chem. Res., 1995, 28, 321–327, DOI:10.1021/ar00056a001.
- J. Tejler, E. Tullberg, T. Frejd, H. Leffler and U. J. Nilsson, Synthesis of multivalent lactose derivatives by 1,3-dipolar cycloadditions: selective galectin-1 inhibition, Carbohydr. Res., 2006, 341, 1353–1362, DOI:10.1016/j.carres.2006.04.028.
- B. Belardi, G. P. O'Donoghue, A. W. Smith, J. T. Groves and C. R. Bertozzi, Investigating Cell Surface Galectin-Mediated Cross-Linking on Glycoengineered Cells, J. Am. Chem. Soc., 2012, 134, 9549–9552, DOI:10.1021/ja301694s.
- C. Bonduelle and S. Lecommandoux, Synthetic Glycopolypeptides as Biomimetic Analogues of Natural Glycoproteins, Biomacromolecules, 2013, 14, 2973–2983, DOI:10.1021/bm4008088.
- C. Lavilla, G. Yilmaz, V. Uzunova, R. Napier, C. R. Becer and A. Heise, Block-Sequence-Specific Glycopolypeptides with Selective Lectin Binding Properties, Biomacromolecules, 2017, 18, 1928–1936, DOI:10.1021/acs.biomac.7b00356.
- Y. Hou, S. Cao, X. Li, B. Wang, Y. Pei, L. Wang and Z. Pei, One-Step Synthesis of Dual Clickable Nanospheres via Ultrasonic-Assisted Click Polymerization for Biological Applications, ACS Appl. Mater. Interfaces, 2014, 6, 16909–16917, DOI:10.1021/am504479w.
- M. F. Troncoso, M. T. Elola, D. O. Croci and G. A. Rabinovich, Integrating structure and function of ‘tandem-repeat’ galectins, Front. Biosci., Scholar Ed., 2012, 4, 864–887, DOI:10.2741/s305.
- C. Shao, X. Li, Z. Pei, D. Liu, L. Wang, H. Dong and Y. Pei, Facile fabrication of glycopolymer-based iron oxide nanoparticles and their applications in the carbohydrate–lectin interaction and targeted cell imaging, Polym. Chem., 2016, 7, 1337–1344, 10.1039/C5PY01954K.
- A. Aykaç, M. C. Martos-Maldonado, J. M. Casas-Solvas, I. Quesada-Soriano, F. García-Maroto, L. García-Fuentes and A. Vargas-Berenguel, β-Cyclodextrin-Bearing Gold Glyconanoparticles for the Development of Site Specific Drug Delivery Systems, Langmuir, 2014, 30, 234–242, DOI:10.1021/la403454p.
- Q. Liu, P. Sacco, E. Marsich, F. Furlani, C. Arib, N. Djaker, M. Lamy de la Chapelle, I. Donati and J. Spadavecchia, Lactose-Modified Chitosan Gold(III)-PEGylated Complex-Bioconjugates: From Synthesis to Interaction with Targeted Galectin-1 Protein, Bioconjugate Chem., 2018, 29, 3352–3361, DOI:10.1021/acs.bioconjchem.8b00520.
- M. C. Miller, I. V. Nesmelova, D. Platt, A. Klyosov and K. H. Mayo, The carbohydrate-binding domain on galectin-1 is more extensive for a complex glycan than for simple saccharides: implications for galectin–glycan interactions at the cell surface, Biochem. J., 2009, 421, 211–221, DOI:10.1042/BJ20090265.
- Y. Yang, X.-C. Xue, X.-F. Jin, L.-J. Wang, Y.-L. Sha and Z.-J. Li, Synthesis of multivalent N-acetyl lactosamine modified quantum dots for the study of carbohydrate and galectin-3 interactions, Tetrahedron, 2012, 68, 7148–7154, DOI:10.1016/j.tet.2012.06.035.
- I. García, A. Sánchez-Iglesias, M. Henriksen-Lacey, M. Grzelczak, S. Penadés and L. M. Liz-Marzán, Glycans as Biofunctional Ligands for Gold Nanorods: Stability and Targeting in Protein-Rich Media, J. Am. Chem. Soc., 2015, 137, 3686–3692, DOI:10.1021/jacs.5b01001.
- A. Restuccia, Y. F. Tian, J. H. Collier and G. A. Hudalla, Self-Assembled Glycopeptide Nanofibers as Modulators of Galectin-1 Bioactivity, Cell. Mol. Bioeng., 2015, 8, 471–487, DOI:10.1007/s12195-015-0399-2.
- A. Restuccia, M. M. Fettis, S. A. Farhadi, M. D. Molinaro, B. Kane and G. A. Hudalla, Evaluation of Self-Assembled Glycopeptide Nanofibers Modified with N,N′-Diacetyllactosamine for Selective Galectin-3 Recognition and Inhibition, ACS Biomater. Sci. Eng., 2018, 4, 3451–3459, DOI:10.1021/acsbiomaterials.8b00611.
- T. K. van den Berg, H. Honing, N. Franke, A. van Remoortere, W. E. C. M. Schiphorst, F.-T. Liu, A. M. Deelder, R. D. Cummings, C. H. Hokke and I. van Die, LacdiNAc-Glycans Constitute a Parasite Pattern for Galectin-3-Mediated Immune Recognition, J. Immunol., 2004, 173, 1902–1907, DOI:10.4049/jimmunol.173.3.1902.
- P. F. Blackmore and S. Eisoldt, The neoglycoprotein mannose–bovine serum albumin, but not progesterone, activates T-type calcium channels in human spermatozoa, Mol. Hum. Reprod., 1999, 5, 498–506, DOI:10.1093/molehr/5.6.498.
- Y. S. Oh, H. S. Ahn and M. C. Gye, Fucosyl neoglycoprotein binds to mouse epididymal spermatozoa and inhibits sperm binding to the egg zona pellucida, Andrologia, 2013, 45, 363–368, DOI:10.1111/and.12024.
- B. X. Huang, H.-Y. Kim and C. Dass, Probing three-dimensional structure of bovine serum albumin by chemical cross-linking and mass spectrometry, J. Am. Soc. Mass Spectrom., 2004, 15, 1237–1247, DOI:10.1016/j.jasms.2004.05.004.
- A. Šimonová, C. E. Kupper, S. Böcker, A. Müller, K. Hofbauerová, H. Pelantová, L. Elling, V. Krěn and P. Bojarová, Chemo-enzymatic synthesis of LacdiNAc dimers of varying length as novel galectin ligands, J. Mol. Catal. B: Enzym., 2014, 101, 47–55, DOI:10.1016/j.molcatb.2013.12.018.
- C. E. Kupper, S. Böcker, H. Liu, C. Adamzyk, J. van de Kamp, T. Recker, B. Lethaus, W. Jahnen-Dechent, S. Neuss, G. Müller-Newen and L. Elling, Fluorescent SNAP-Tag Galectin Fusion Proteins as Novel Tools in Glycobiology, Curr. Pharm. Des., 2013, 19, 5457–5467, DOI:10.2174/1381612811319300017.
- S. Böcker, D. Laaf and L. Elling, Galectin Binding to Neo-Glycoproteins: LacDiNAc Conjugated BSA as Ligand for Human Galectin-3, Biomolecules, 2015, 5, 1671–1696, DOI:10.3390/biom5031671.
- L. Bumba, D. Laaf, V. Spiwok, L. Elling, V. Křen and P. Bojarová, Poly-N-Acetyllactosamine Neo-Glycoproteins as Nanomolar Ligands of Human Galectin-3: Binding Kinetics and Modeling, Int. J. Mol. Sci., 2018, 19, 372, DOI:10.3390/ijms19020372.
- S. Böcker and L. Elling, Biotinylated N-Acetyllactosamine- and N,N-Diacetyllactosamine-Based Oligosaccharides as Novel Ligands for Human Galectin-3, Bioengineering, 2017, 4, 31, DOI:10.3390/bioengineering4020031.
- D. Laaf, P. Bojarová, H. Pelantová, V. Křen and L. Elling, Tailored Multivalent Neo-Glycoproteins: Synthesis, Evaluation, and Application of a Library of Galectin-3-Binding Glycan Ligands, Bioconjugate Chem., 2017, 28, 2832–2840, DOI:10.1021/acs.bioconjchem.7b00520.
- D. Laaf, P. Bojarová, B. Mikulová, H. Pelantová, V. Křen and L. Elling, Two-Step Enzymatic Synthesis of β-D-N-Acetylgalactosamine-(1 → 4)-D-N-acetylglucosamine (LacdiNAc) Chitooligomers for Deciphering Galectin Binding Behavior, Adv. Synth. Catal., 2017, 359, 2101–2108, DOI:10.1002/adsc.201700331.
- T. Fischöder, D. Laaf, C. Dey and L. Elling, Enzymatic Synthesis of N-Acetyllactosamine (LacNAc) Type 1 Oligomers and Characterization as Multivalent Galectin Ligands, Molecules, 2017, 22, 1320, DOI:10.3390/molecules22081320.
- H. Zhang, D. Laaf, L. Elling and R. J. Pieters, Thiodigalactoside–Bovine Serum Albumin Conjugates as High-Potency Inhibitors of Galectin-3: An Outstanding Example of Multivalent Presentation of Small Molecule Inhibitors, Bioconjugate Chem., 2018, 29, 1266–1275, DOI:10.1021/acs.bioconjchem.8b00047.
- M. Hoffmann, M. R. Hayes, J. Pietruszka and L. Elling, Synthesis of the Thomsen-Friedenreich-antigen (TF-antigen) and binding of Galectin-3 to TF-antigen presenting neo-glycoproteins, Glycoconjugate J., 2020, 37, 457–470, DOI:10.1007/s10719-020-09926-y.
- H. Wang, W. Huang, J. Orwenyo, A. Banerjee, G. R. Vasta and L.-X. Wang, Design and synthesis of glycoprotein-based multivalent glyco-ligands for influenza hemagglutinin and human galectin-3, Bioorg. Med. Chem., 2013, 21, 2037–2044, DOI:10.1016/j.bmc.2013.01.028.
- V. Heine, M. Hovorková, M. Vlachová, M. Filipová, L. Bumba, O. Janoušková, M. Hubálek, J. Cvačka, L. Petrásková, H. Pelantová, V. Křen, L. Elling and P. Bojarová, Immunoprotective neo-glycoproteins: Chemoenzymatic synthesis of multivalent glycomimetics for inhibition of cancer-related galectin-3, Eur. J. Med. Chem., 2021, 220, 113500, DOI:10.1016/j.ejmech.2021.113500.
- C. Bonduelle, H. Oliveira, C. Gauche, J. Huang, A. Heise and S. Lecommandoux, Multivalent effect of glycopolypeptide based nanoparticles for galectin binding, Chem. Commun., 2016, 52, 11251–11254, 10.1039/c6cc06437j.
- T. Freichel, D. Laaf, M. Hoffmann, P. B. Konietzny, V. Heine, R. Wawrzinek, C. Rademacher, N. L. Snyder, L. Elling and L. Hartmann, Effects of linker and liposome anchoring on lactose-functionalized glycomacromolecules as multivalent ligands for binding galectin-3, RSC Adv., 2019, 9, 23484–23497, 10.1039/c9ra05497a.
- T. Freichel, V. Heine, D. Laaf, E. E. Mackintosh, S. Sarafova, L. Elling, N. L. Snyder and L. Hartmann, Sequence-Defined Heteromultivalent Precision Glycomacromolecules Bearing Sulfonated/Sulfated Nonglycosidic Moieties Preferentially Bind Galectin-3 and Delay Wound Healing of a Galectin-3 Positive Tumor Cell Line in an In Vitro Wound Scratch Assay, Macromol. Biosci., 2020, 20, 2000163, DOI:10.1002/mabi.202000163.
- S. E. Sherman, Q. Xiao and V. Percec, Mimicking Complex Biological Membranes and Their Programmable Glycan Ligands with Dendrimersomes and Glycodendrimersomes, Chem. Rev., 2017, 117, 6538–6631, DOI:10.1021/acs.chemrev.7b00097.
- V. Percec, P. Leowanawat, H.-J. Sun, O. Kulikov, C. D. Nusbaum, T. M. Tran, A. Bertin, D. A. Wilson, M. Peterca, S. Zhang, N. P. Kamat, K. Vargo, D. Moock, E. D. Johnston, D. A. Hammer, D. J. Pochan, Y. Chen, Y. M. Chabre, T. C. Shiao, M. Bergeron-Brlek, S. André, R. Roy, H.-J. Gabius and P. A. Heiney, Modular Synthesis of Amphiphilic Janus Glycodendrimers and Their Self-Assembly into Glycodendrimersomes and Other Complex Architectures with Bioactivity to Biomedically Relevant Lectins, J. Am. Chem. Soc., 2013, 135, 9055–9077, DOI:10.1021/ja403323y.
- S. Zhang, R.-O. Moussodia, H.-J. Sun, P. Leowanawat, A. Muncan, C. D. Nusbaum, K. M. Chelling, P. A. Heiney, M. L. Klein, S. André, R. Roy, H.-J. Gabius and V. Percec, Mimicking Biological Membranes with Programmable Glycan Ligands Self-Assembled from Amphiphilic Janus Glycodendrimers, Angew. Chem., Int. Ed., 2014, 53, 10899–10903, DOI:10.1002/anie.201403186.
- S. Zhang, R.-O. Moussodia, S. Vértesy, S. André, M. L. Klein, H.-J. Gabius and V. Percec, Unraveling functional significance of natural variations of a human galectin by glycodendrimersomes with programmable
glycan surface, Proc. Natl. Acad. Sci. U. S. A., 2015, 112, 5585–5590, DOI:10.1073/pnas.1506220112.
- S. Zhang, Q. Xiao, S. E. Sherman, A. Muncan, A. D. M. Ramos Vicente, Z. Wang, D. A. Hammer, D. Williams, Y. Chen, D. J. Pochan, S. Vértesy, S. André, M. L. Klein, H.-J. Gabius and V. Percec, Glycodendrimersomes from Sequence-Defined Janus Glycodendrimers Reveal High Activity and Sensor Capacity for the Agglutination by Natural Variants of Human Lectins, J. Am. Chem. Soc., 2015, 137, 13334–13344, DOI:10.1021/jacs.5b08844.
- S. Zhang, R.-O. Moussodia, C. Murzeau, H.-J. Sun, M. L. Klein, S. Vértesy, S. André, R. Roy, H.-J. Gabius and V. Percec, Dissecting Molecular Aspects of Cell Interactions Using Glycodendrimersomes with Programmable Glycan Presentation and Engineered Human Lectins, Angew. Chem., Int. Ed., 2015, 54, 4036–4040, DOI:10.1002/anie.201410882.
- J. Kopitz, Q. Xiao, A.-K. Ludwig, A. Romero, M. Michalak, S. E. Sherman, X. Zhou, C. Dazen, S. Vértesy, H. Kaltner, M. L. Klein, H.-J. Gabius and V. Percec, Reaction of a Programmable Glycan Presentation of Glycodendrimersomes and Cells with Engineered Human Lectins To Show the Sugar Functionality of the Cell Surface, Angew. Chem., Int. Ed., 2017, 56, 14677–14681, DOI:10.1002/anie.201708237.
- Q. Xiao, A.-K. Ludwig, C. Romanò, I. Buzzacchera, S. E. Sherman, M. Vetro, S. Vértesy, H. Kaltner, E. H. Reed, M. Möller, C. J. Wilson, D. A. Hammer, S. Oscarson, M. L. Klein, H.-J. Gabius and V. Percec, Exploring functional pairing between surface glycoconjugates and human galectins using programmable glycodendrimersomes, Proc. Natl. Acad. Sci. U. S. A., 2018, 115, E2509–E2518, DOI:10.1073/pnas.1720055115.
- A.-K. Ludwig, M. Michalak, Q. Xiao, U. Gilles, F. J. Medrano, H. Ma, F. G. FitzGerald, W. D. Hasley, A. Melendez-Davila, M. Liu, K. Rahimi, N. Y. Kostina, C. Rodriguez-Emmenegger, M. Möller, I. Lindner, H. Kaltner, M. Cudic, D. Reusch, J. Kopitz, A. Romero, S. Oscarson, M. L. Klein, H.-J. Gabius and V. Percec, Design–functionality relationships for adhesion/growth-regulatory galectins, Proc. Natl. Acad. Sci. U. S. A., 2019, 116, 2837–2842, DOI:10.1073/pnas.1813515116.
- C. Rodriguez-Emmenegger, Q. Xiao, N. Y. Kostina, S. E. Sherman, K. Rahimi, B. E. Partridge, S. Li, D. Sahoo, A. M. Reveron Perez, I. Buzzacchera, H. Han, M. Kerzner, I. Malhotra, M. Möller, C. J. Wilson, M. C. Good, M. Goulian, T. Baumgart, M. L. Klein and V. Percec, Encoding biological recognition in a bicomponent cell-membrane mimic, Proc. Natl. Acad. Sci. U. S. A., 2019, 116, 5376–5382, DOI:10.1073/pnas.1821924116.
- Q. Xiao, S. S. Yadavalli, S. Zhang, S. E. Sherman, E. Fiorin, L. da Silva, D. A. Wilson, D. A. Hammer, S. André, H.-J. Gabius, M. L. Klein, M. Goulian and V. Percec, Bioactive cell-like hybrids coassembled from (glyco)dendrimersomes with bacterial membranes, Proc. Natl. Acad. Sci. U. S. A., 2016, 113, E1134–E1141, DOI:10.1073/pnas.1525589113.
- P. R. Crocker, J. C. Paulson and A. Varki, Siglecs and their roles in the immune system, Nat. Rev. Immunol., 2007, 7, 255–266, DOI:10.1038/nri2056.
- A. Varki, Glycan-based interactions involving vertebrate sialic-acid-recognizing proteins, Nature, 2007, 446, 1023–1029, DOI:10.1038/nature05816.
- M. S. Macauley, P. R. Crocker and J. C. Paulson, Siglec-mediated regulation of immune cell function in disease, Nat. Rev. Immunol., 2014, 14, 653–666, DOI:10.1038/nri3737.
- N. Kawasaki, C. Rademacher and J. C. Paulson, CD22 Regulates Adaptive and Innate Immune Responses of B Cells, J. Innate Immun., 2011, 3, 411–419, DOI:10.1159/000322375.
- V. S. Mahajan and S. Pillai, Sialic acids and autoimmune disease, Immunol. Rev., 2016, 269, 145–161, DOI:10.1111/imr.12344.
- S. S. Siddiqui, R. Matar, M. Merheb, R. Hodeify, C. G. Vazhappilly, J. Marton, S. A. Shamsuddin and H. Al Zouabi, Siglecs in Brain Function and Neurological Disorders, Cells, 2019, 8, 1125, DOI:10.3390/cells8101125.
- J. Munkley and E. Scott, Targeting Aberrant Sialylation to Treat Cancer, Medicines, 2019, 6, 102, DOI:10.3390/medicines6040102.
- V. Almeida-Marrero, F. Bethlehem, S. Longo, M. C. Bertolino, T. Torres, J. Huskens and A. de la Escosura, Tailored Multivalent Targeting of Siglecs with Photosensitizing Liposome Nanocarriers, Angew. Chem., Int. Ed., 2022, 61, e202206900, DOI:10.1002/anie.202206900.
- G.-j Liu, Y. Zhang, L. Zhou, L.-y Jia, G. Jiang, G.-w Xing and S. Wang, A water-soluble AIE-active polyvalent glycocluster: design, synthesis and studies on carbohydrate–lectin interactions for visualization of Siglec distributions in living cell membranes, Chem. Commun., 2019, 55, 9869–9872, 10.1039/C9CC05008F.
- G. St-Pierre, S. Pal, M. E. Østergaard, T. Zhou, J. Yu, M. Tanowitz, P. P. Seth and S. Hanessian, Synthesis and biological evaluation of sialyl-oligonucleotide conjugates targeting leukocyte B trans-membranal receptor CD22 as delivery agents for nucleic acid drugs, Bioorg. Med. Chem., 2016, 24, 2397–2409, DOI:10.1016/j.bmc.2016.03.047.
- J. E. Hudak, S. M. Canham and C. R. Bertozzi, Glycocalyx engineering reveals a Siglec-based mechanism for NK cell immunoevasion, Nat. Chem. Biol., 2014, 10, 69–75, DOI:10.1038/nchembio.1388.
- T. Yamaji, T. Teranishi, M. S. Alphey, P. R. Crocker and Y. Hashimoto, A Small Region of the Natural Killer Cell Receptor, Siglec-7, Is Responsible for Its Preferred Binding to α2,8-Disialyl and Branched α2,6-Sialyl Residues. A COMPARISON WITH Siglec-9, J. Biol. Chem., 2002, 277, 6324–6332, DOI:10.1074/jbc.M110146200.
- S. Ohira, Y. Yasuda, I. Tomita, K. Kitajima, T. Takahashi, C. Sato and H. Tanaka, Synthesis of end-functionalized glycopolymers containing α(2,8) disialic acids via π-allyl nickel catalyzed coordinating polymerization and their interaction with Siglec-7, Chem. Commun., 2017, 53, 553–556, 10.1039/C6CC07115E.
- S. Yamaguchi, A. Yoshimura, Y. Yasuda, A. Mori, H. Tanaka, T. Takahashi, K. Kitajima and C. Sato, Chemical Synthesis and Evaluation of a Disialic Acid-Containing Dextran Polymer as an Inhibitor for the Interaction between Siglec 7 and Its Ligand, ChemBioChem, 2017, 18, 1194–1203, DOI:10.1002/cbic.201600694.
- C. S. Delaveris, A. J. Wilk, N. M. Riley, J. C. Stark, S. S. Yang, A. J. Rogers, T. Ranganath, K. C. Nadeau, C. A. Blish and C. R. Bertozzi, Synthetic Siglec-9 Agonists Inhibit Neutrophil Activation Associated with COVID-19, ACS Cent. Sci., 2021, 7, 650–657, DOI:10.1021/acscentsci.0c01669.
- C. S. Delaveris, S. H. Chiu, N. M. Riley and C. R. Bertozzi, Modulation of immune cell reactivity with cis-binding Siglec agonists, Proc. Natl. Acad. Sci. U. S. A., 2021, 118, e2012408118, DOI:10.1073/pnas.2012408118.
- W. Peng and J. C. Paulson, CD22 Ligands on a Natural N-Glycan Scaffold Efficiently Deliver Toxins to B-Lymphoma Cells, J. Am. Chem. Soc., 2017, 139, 12450–12458, DOI:10.1021/jacs.7b03208.
- J.-K. Rhee, M. Baksh, C. Nycholat, J. C. Paulson, H. Kitagishi and M. G. Finn, Glycan-Targeted Virus-like Nanoparticles for Photodynamic Therapy, Biomacromolecules, 2012, 13, 2333–2338, DOI:10.1021/bm300578p.
- T. Angata, C. M. Nycholat and M. S. Macauley, Therapeutic Targeting of Siglecs using Antibody- and Glycan-Based Approaches, Trends Pharmacol. Sci., 2015, 36, 645–660, DOI:10.1016/j.tips.2015.06.008.
- C. D. Rillahan, M. S. Macauley, E. Schwartz, Y. He, R. McBride, B. M. Arlian, J. Rangarajan, V. V. Fokin and J. C. Paulson, Disubstituted sialic acid ligands targeting siglecs CD33 and CD22 associated with myeloid leukaemias and B cell lymphomas, Chem. Sci., 2014, 5, 2398–2406, 10.1039/C4SC00451E.
- F. Pfrengle, M. S. Macauley, N. Kawasaki and J. C. Paulson, Copresentation of Antigen and Ligands of Siglec-G Induces B Cell Tolerance Independent of CD22, J. Immunol., 2013, 191, 1724–1731, DOI:10.4049/jimmunol.1300921.
- M. S. Macauley, F. Pfrengle, C. Rademacher, C. M. Nycholat, A. J. Gale, A. von Drygalski and J. C. Paulson, Antigenic liposomes displaying CD22 ligands induce antigen-specific B cell apoptosis, J. Clin. Invest., 2013, 123, 3074–3083, DOI:10.1172/JCI69187.
- Y. Sun, S. Hong, R. Xie, R. Huang, R. Lei, B. Cheng, D.-E. Sun, Y. Du, C. M. Nycholat, J. C. Paulson and X. Chen, Mechanistic Investigation and Multiplexing of Liposome-Assisted Metabolic Glycan Labeling, J. Am. Chem. Soc., 2018, 140, 3592–3602, DOI:10.1021/jacs.7b10990.
- C. M. Nycholat, C. Rademacher, N. Kawasaki and J. C. Paulson, In Silico-Aided Design of a Glycan Ligand of Sialoadhesin for in Vivo Targeting of Macrophages, J. Am. Chem. Soc., 2012, 134, 15696–15699, DOI:10.1021/ja307501e.
- A. Srivastava, B. M. Arlian, L. Pang, T. K. Kishimoto and J. C. Paulson, Tolerogenic Nanoparticles Impacting B and T Lymphocyte Responses Delay Autoimmune Arthritis in K/BxN Mice, ACS Chem. Biol., 2021, 16, 1985–1993, DOI:10.1021/acschembio.1c00212.
- S. Duan, B. M. Arlian, C. M. Nycholat, Y. Wei, H. Tateno, S. A. Smith, M. S. Macauley, Z. Zhu, B. S. Bochner and J. C. Paulson, Nanoparticles Displaying Allergen and Siglec-8 Ligands Suppress IgE-FcεRI-Mediated Anaphylaxis and Desensitize Mast Cells to Subsequent Antigen Challenge, J. Immunol., 2021, 206, 2290–2300, DOI:10.4049/jimmunol.1901212.
- K. J. Bednar, C. M. Nycholat, T. S. Rao, J. C. Paulson, W.-P. Fung-Leung and M. S. Macauley, Exploiting CD22 To Selectively Tolerize Autoantibody Producing B-Cells in Rheumatoid Arthritis, ACS Chem. Biol., 2019, 14, 644–654, DOI:10.1021/acschembio.8b01018.
- C. M. Nycholat, S. Duan, E. Knuplez, C. Worth, M. Elich, A. Yao, J. O’Sullivan, R. McBride, Y. Wei, S. M. Fernandes, Z. Zhu, R. L. Schnaar, B. S. Bochner and J. C. Paulson, A Sulfonamide Sialoside Analogue for Targeting Siglec-8 and -F on Immune Cells, J. Am. Chem. Soc., 2019, 141, 14032–14037, DOI:10.1021/jacs.9b05769.
- A. J. Affandi, J. Grabowska, K. Olesek, M. Lopez Venegas, A. Barbaria, E. Rodríguez, P. P. G. Mulder, H. J. Pijffers, M. Ambrosini, H. Kalay, T. O’Toole, E. S. Zwart, G. Kazemier, K. Nazmi, F. J. Bikker, J. Stöckl, A. J. M. van den Eertwegh, T. D. de Gruijl, G. Storm, Y. van Kooyk and J. M. M. den Haan, Selective tumor antigen vaccine delivery to human CD169+ antigen-presenting cells using ganglioside-liposomes, Proc. Natl. Acad. Sci. U. S. A., 2020, 117, 27528–27539, DOI:10.1073/pnas.2006186117.
- F. Xu, A. Bandara, H. Akiyama, B. Eshaghi, D. Stelter, T. Keyes, J. E. Straub, S. Gummuluru and B. M. Reinhard, Membrane-wrapped nanoparticles probe divergent roles of GM3 and phosphatidylserine in lipid-mediated viral entry pathways, Proc. Natl. Acad. Sci. U. S. A., 2018, 115, E9041–E9050, DOI:10.1073/pnas.1804292115.
- X. Yu, A. Feizpour, N.-G. P. Ramirez, L. Wu, H. Akiyama, F. Xu, S. Gummuluru and B. M. Reinhard, Glycosphingolipid-functionalized nanoparticles recapitulate CD169-dependent HIV-1 uptake and trafficking in dendritic cells, Nat. Commun., 2014, 5, 4136, DOI:10.1038/ncomms5136.
|
This journal is © The Royal Society of Chemistry 2023 |