DOI:
10.1039/D2CC06957A
(Feature Article)
Chem. Commun., 2023,
59, 2726-2738
Visible-light-induced reactions of methylenecyclopropanes (MCPs)
Received
22nd December 2022
, Accepted 1st February 2023
First published on 1st February 2023
Abstract
Diverse, visible-light-induced transformations of methylenecyclopropanes (MCPs) have been reported in recent years, attracting significant attention from synthetic chemists. As readily accessible strained molecules, MCPs have sufficient reactivity to selectively generate different target products, through reactions with various radical species upon visible-light irradiation under regulated reaction conditions. These transformations can be classified into three subcategories of reaction pathway, forming ring-opened products, cyclopropane derivatives, and alkynes. These products include pharmaceutical intermediates and polycyclic/heterocyclic compounds that are challenging to obtain using traditional methods. This review summarizes the recent advancements in this field.
Introduction
Cyclopropane is strained because the “bent” carbon–carbon bonds overlap poorly (Scheme 1). The total ring strain in cyclopropane is 27.5 kcal mol−1, in which about three-quarters of the strain is the bond angle strain. Introducing a nominally trigonal carbon center into a three-membered ring system increases its ring strain. The SE (strain energy) for methylenecyclopropane is 41.0 kcal mol−1, while that for 1-methylcyclopropene is 53.1 kcal mol−1.1 Wiberg suggested that each additional sp2 center in a three-membered ring increases the SE by 12–14 kcal mol−1 and recognized that part of the reason is due to the enlarged strain connected with the sp2 carbon center. Afterwards, Borden concluded that the loss of a firm tert-C–H bond upon introducing the sp2 carbon in methylenecyclopropane is the primary source of the additional strain energy.1,2 As a class of highly strained but readily accessible and sufficiently reactive molecules, methylenecyclopropanes (MCPs) can undergo a range of ring-opening reactions in which the released strain provides a thermodynamic driving force.3
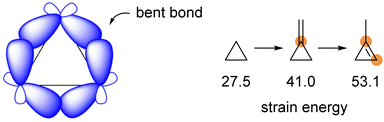 |
| Scheme 1 Three-membered rings with high strain (the SE is given in kcal mol−1). | |
MCPs exhibit preeminent physical and chemical properties, which offer opportunities to participate in various attractive chemical transformations. These reactions can access cyclopropane-containing frameworks and other ring-opened compounds that are challenging to obtain conventionally.4 These transformations usually occur smoothly under mild reaction conditions such as with heating,5 Lewis/Brønsted acids,6 transition metal catalysis,7 radical-promoted processes,8 and nucleophilic species-promoted ring-opening reactions,9 giving tremendous potential in organic synthesis (Scheme 2).
 |
| Scheme 2 Transformations of MCPs. | |
In most radical reactions of MCPs and vinylcyclopropanes (VCPs), the dominant contributor to reactivity is the formation of 1, the cyclopropylcarbinyl radical species (Scheme 3). The structure of 1, deduced from its electronic spin resonance spectrum10 and in agreement with calculations (STO-3G basis set),11 is in the bisected conformation shown, and is predicted to be 1.4 kcal mol−1 more stable than its perpendicularly oriented counterpart. The opening of the cyclopropylcarbinyl radical to the butenyl radical 2 is among the fastest radical processes known, and has been widely designed as a radical clock to conduct mechanistic studies for probing radical processes.12
 |
| Scheme 3 Cyclopropylcarbinyl radical species. | |
Precursors to cyclopropylcarbinyl radicals mainly cover VCPs and MCPs. Over the past three decades, many radical reactions of MCPs have been established, providing effective routes to access polycyclic and heterocyclic compounds, including bioactive drugs.13a–am,8a In the early 1990s, synthetic applications of radical reactions involving MCPs appeared. For example, in 1991, Priestley's group reported that readily available methylenecyclopropanes 3 efficiently undergo annulation with inactivated and electron-rich alkenes 4 to provide methylenecyclopentanes 5via thiyl-radical-catalyzed chain cyclization (Scheme 4).14
 |
| Scheme 4 Thiyl-radical-catalyzed chain cyclization. | |
Afterwards, a brilliant and efficient method was established to access methylenecyclohexyl radicals from cyclopropylcarbinyl radicals. In this method, the (methylenecyclopropyl)propyl radical 6, which is generated from the precursor, undergoes initial 5-exo cyclization, leading to the cyclopropylcarbinyl radical 7, and then 7 undergoes ring-opening to afford the more stable methylenecyclohexyl radical 8.14,15 For instance, in 1998, the Kilburn group reported the SmI2-mediated cascade reaction of methylenecyclopropyl ketone 9, providing a short route to the natural product paeonilactone B (Scheme 5).13e
 |
| Scheme 5 Synthesis of paeonilactone B from MCPs. | |
In 2004, Huang and co-workers reported the tandem radical cyclization reactions of MCPs for the first time (Scheme 6).13n In this protocol, MCP 10 reacted with Mn(OAc)3·2H2O and malonic acid diethyl ester 11 in HOAc/Ac2O (9
:
1) via two SET processes, providing 2-(3,4-dihydronaphthalen-2-yl)malonic acid diethyl ester 12 in 68% yield. In 2005, our group also reported a radical-promoted cyclization reaction using Mn(OAc)3·2H2O (Scheme 6, bottom). In this protocol, MCPs were used as the substrates to react with 2,4-pentanedione or 1,3-cyclohexanedione, which was applied for α-carbonyl radical generation, producing 4,5-dihydrofuran derivatives in moderate to good yields.13o After that, tandem radical cyclization reactions of MCPs involving various radicals were abundantly developed, involving a variety of radicals, such as perfluoroalkyl radicals,13q phosphite radicals,13p,13an α-carbonyl radicals,13r,13u thiyl radicals,13t selenium radicals,13s trifluoromethyl radicals,8a,16 and N-centered radicals.13y
 |
| Scheme 6 Radical-promoted tandem cyclization reactions. | |
Although radicals are highly reactive species, manipulating radical reactions to undergo selective processes is highly significant. One of the most rapidly increasing areas of radical chemistry in organic synthesis is photoredox catalysis, and it has been a helpful tool for chemists to achieve unique chemical reactivity, especially with the breakthrough merger of organocatalysis and photocatalysis.17 Based on the catalytic system employed, this field, in principle, can be classified into three subcategories: proceeding through a single electron transfer (SET) process, an energy transfer (EnT) process, or an atom transfer (AT) process.
In recent years, visible-light-induced organic reactions of MCPs have become well established, consisting of ring-opening reactions, cyclopropane-producing reactions, and ring-opening reactions via a non-classical radical clock. This feature article aims to introduce the recent developments in these reactions, from our group and other researchers.
Ring-opening reactions via cyclopropylcarbinyl radical intermediates
In 2006, the Huang's group reported the ring-opening process for MCPs under irradiation with a tungsten lamp (300 W) (Scheme 7).13s In this reaction, when MCP 13 and diphenyl diselenide were used as the substrates, with toluene as the solvent, the ring-opened product 14 was obtained under a nitrogen atmosphere. If N2 protection was not applied and EtOH was used as the solvent, the cyclobutanol derivative 15 was generated in 37% yield, along with 14 in 25% yield. Subsequently, they found that adding dibenzoyl peroxide avoided the formation of 14, and the yield of 15 was improved to 66%. This report demonstrated the following reaction process: diphenyl diselenide is first oxidized by oxygen or dibenzoyl peroxide to benzeneselenenic anhydride 16. Then, cyclopropyl cationic intermediates 17-A and 17-B are generated upon the introduction of 13. After rearrangement, SN1 substitution, and alcoholysis, the final product 15 is generated. However, catalytic reactions of MCPs induced by visible-light irradiation were not established during the subsequent decade.
 |
| Scheme 7 Difunctionalization of MCPs with diphenyl diselenide. | |
Fluorinated compounds are essential in functional materials,18 pharmaceuticals,19 and agrochemicals.20 Trifluoromethylation21 and difluoroalkylation22 are considered to be significant features in the preparation of fluorinated compounds because integrating these groups brings enhanced biological and pharmaceutical properties to the molecules, such as better metabolic stability, higher bioavailability, and greater lipophilicity.
Imidoyl radicals are vital synthetic intermediates that undergo intermolecular and intramolecular radical addition to olefins, alkynes, aromatics, etc.23 They are often utilized to synthesize nitrogen-containing heterocycles such as indoles, pyrrolidines, and quinolines. One of the known methods to engender imidoyl radicals is the addition of radicals to isonitriles and isothiocyanates. In 2016, inspired by the previous work of Studer and co-workers,24 our group disclosed the first protocol that applied photoredox catalysis for MCP transformation reactions (Scheme 8).25 In this protocol, [Ir(ppy)2(dtbbpy)]PF6 is employed as the photocatalyst to enable the photocatalytic trifluoromethylation of isonitrile-tethered MCPs 18 with the Togni reagent. Two six-membered rings and three new C–C bonds were formed in a one-pot reaction, showing the high efficiency of the protocol. Moreover, these products can be easily transformed into 6-(trifluoromethyl)-benzo[k]phenanthridine derivatives. In the proposed mechanism, initially, the excited state (E1/2 = −0.96 V vs. SCE) of the Ir catalyst reduces the Togni reagent (E1/2 = −1.34 V vs. SCE)26 to the trifluoromethyl radical through an SET process. It should be noted that the values of the redox potentials do not support a valid spontaneous SET process, although this is not impossible. Sharma et al. proposed an alternative mechanism in which the trifluoromethyl radical is generated via photo-induced fission without the intervention of the catalyst,23b which is conceivable because hypervalent iodine(III) reagents readily undergo photodecomposition upon exposure to weak UV light or visible-light to provide radicals.27 Subsequently, in the reaction process, addition of the CF3 radical to the isonitrile group in substrate 20 generates the imidoyl radical. Subsequent attack at the MCP and its ring-opening provide intermediate 21, and 21 undergoes direct radical cyclization with its arene moiety to give the cyclohexadienyl radical. After SET oxidation and deprotonation, the final product 22 is afforded. To illustrate the synthetic utility of the products, in the presence of NBS and dibenzoyl peroxide, 22 can then be transformed to 23, which contains a larger delocalized framework.
 |
| Scheme 8 Trifluoromethylation of isonitrile-tethered MCPs. | |
In the same year, Li and co-workers reported the catalytic difluoroalkylation/C–H cascade annulation reactions of cyclopropyl olefins, including VCPs 24 and MCP 26, induced by visible-light irradiation for the synthesis of partially hydrogenated naphthalene and quinoline derivatives (25, 27) with various difluorinated side chains (Scheme 9).28 In this protocol, ethyl bromodifluoroacetate (or other brominated compounds as shown in Scheme 9) was used as the radical precursor to react with MCPs or VCPs, providing similar radical intermediates 29 and 30 after radical addition, as was described before. This protocol will provide access to other functionalized bicyclic compounds if further derivatization can be developed.
 |
| Scheme 9 Difluoroalkylation of MCPs and VCPs. | |
To realize the CF2H functionalization of MCPs, Wu's group recently applied easily prepared [Ph3PCF2H]+Br− (32)29 for CF2H radical generation, synthesizing CF2H functionalized partially hydrogenated naphthalenes 33 from 31 (Scheme 10).30 [Ph3PCF2H]+Br− provides simple and practical access to CF2H radicals for hydrodifluoromethylation,31 compared with other CF2H radical sources32 such as HCF2SO2Cl, its derivatives and difuoromethylsulfinate salts which are prepared from gaseous HCF2Cl through multiple steps.32a Interestingly, if MCP compounds are tethered with two phenyl rings (13) in this protocol, the difunctionalized product 34 will be obtained without ring-closing of the radical to the aryl moiety. In this report, Wu and co-workers also tested other halides 35 such as α-bromoketones and aryl sulfonyl chlorides 39 for MCPs (31, 37), and found that various functionalized products (36, 38, 40) were obtained in moderate to excellent yields. Synthetic transformations, including epoxidation and cyclization, highlight the potential applications of this methodology in organic synthesis and the synthesis of pharmaceutical compounds (Scheme 10, bottom).
 |
| Scheme 10 CF2H functionalization, bromination and chlorination of MCPs. | |
In recent years, N-functionalized pyridinium salts have been employed as versatile reagents for the redox-triggered release of various radical species for organic synthesis.33 In 2017, Xu and co-workers used N-protected 1-aminopyridinium 42 to produce sulfamide radicals via reduction of the photoexcited catalyst *Ir(ppy)3 (Scheme 11).34 In this reaction, after addition of the N-centered radical to MCP 13, SET oxidation by Ir(IV) and aza-pinacol rearrangement, the strained cyclobutanimine derivative 44 was ultimately afforded. After reduction or oxidation, the formed motifs can easily be transformed into valuable groups (45, 46) in natural products and bioactive molecules. In organic synthetic methodologies, pinacol and semi-pinacol rearrangement reactions are essential to construct all-carbon quaternary centers, although the aza-pinacol rearrangement has been less reported (Scheme 12).35 Therefore, this novel visible-light-induced aza-pinacol rearrangement reaction provides a supplement to the ring-expansion reactions of MCPs with nitrogen compounds.36
 |
| Scheme 11 Visible-light-induced aza-pinacol rearrangement reaction. | |
 |
| Scheme 12 Semi-pinacol rearrangement and aza-pinacol rearrangement. | |
The sulfone moiety is also an essential motif in pharmaceuticals,37 agrochemicals,38 natural products,39 and organic materials.40 Among the synthetic routes to install the sulfonyl group, sulfonylation starting from stable and environmentally friendly sulfonyl sources is promising and attractive.41 In 2019, Tang's group developed the visible-light-catalyzed sulfonylation of MCPs 47 with sulfonyl chlorides to synthesize 3-sulfonylated 1,2-dihydronaphthalene derivatives 48 (Scheme 13).42 Compared with the work of Wu and co-workers as discussed above,30 after sulfonyl radical-promoted ring-opening of the MCP, the carbon radical finally preferentially undergoes a ring-closing reaction instead of combining with a nucleophilic species after SET oxidation. It should be noted that the reactions were carried out at a higher temperature (100 °C); thus, the ring-closing reaction can take place by overcoming the high energy barrier at high temperature.
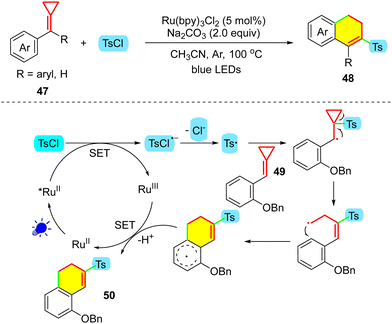 |
| Scheme 13 Sulfonyl radical-promoted MCP ring-opening. | |
Following this work, in 2020, Tang's group developed a new sulfonylation method for MCPs 41 using a cycloketone oxime 51 as the radical precursor (Scheme 14).8d The cycloketone oxime and its derivatives are both stable and easily prepared from cycloketones with hydroxylamine derivatives, and they are endowed with redox-active properties as they can not only accept an electron but also donate an electron in the SET mode.43 Formation of the sulfonyl-radical-bearing alkylnitrile occurs via the SET reduction of 53, β-scission of the iminyl radical intermediate 54, and trapping of sulfur dioxide. Both the cyanoalkyl radical and the cyanoalkylsulfonyl radical were trapped using radical inhibitors. This protocol provides a facile and convenient route to access diverse 2-cyanoalkylsulfonated 3,4-dihydronaphthalenes 52.
 |
| Scheme 14 Sulfonylation of MCPs with cycloketone oxime as the radical precursor. | |
The radical-promoted ring-opening of MCPs can be extended to synthesize phosphine-containing compounds. Huang and co-workers proved that MCP is a suitable acceptor of P-centered radicals.13p In 2020, the group of Hirano and Miura developed the ring-opening diphosphination of MCPs 56 and VCPs 68 with tetraaryldiphosphines 57 to efficiently synthesize the corresponding 1,3-diphenylphosphinopropane- and 1,5-diphenylphosphinopentane-type bidentate ligands 58 and 59, respectively (Scheme 15).13an The ligands 58 and 59 can be transformed to 58-S and 59-S, respectively, by reacting with S8. The addition of BrPPh2 can improve the product yield, and BrPPh2 can be luminescence quenched by Ir(ppy)3, indicating that the excited species Ir(III)* undergoes an SET process to reduce BrPPh2. Since 58-S can be obtained under additive-free conditions, the pathway in which 61 can be directly generated from 60via an energy transfer (EnT) process might be competitive. After attack of the radical to afford 63 and SET oxidation by Ir(IV), the formed cationic species 64 can be trapped by 60 to afford 65, and the diarylphosphine radical 61 is regenerated after SET reduction of the phosphonium species 65, providing 67. This method can readily prepare electronically diverse substituted bidentate ligands.
 |
| Scheme 15 Diarylphosphine-promoted difunctionalization of MCPs and VCPs. | |
The generation of α-carbonyl radicals from the direct oxidization of an enolate usually needs stoichiometric oxidants,44 in which the most frequently used reagent is Mn(OAc)3. However, the debromination of α-carbonyl alkyl bromides45 or radical conjugate addition to electron-deficient olefins17e,46 can provide more atom-economic and environmentally friendly synthetic routes.
In 2019, our group developed a visible-light-induced photocatalytic cascade cyclization reaction of MCPs 69 and 71 to rapidly construct seven- and eight-membered ring-containing polycyclic lactams and lactones 70 and 72 (Scheme 16).8c In this reaction, substrate 69 or 71 produces an α-carbonyl radical through SET reduction, and subsequent cascade cyclization and SET oxidation enable the formation of polycyclic products. In addition, an intermolecular version of the reaction using MCP 31 and BrCH2COOEt as the reactants was also realized but with a lower productivity compared with BrCF2COOEt,28 of which the products 73 can be used as precursors of many valuable compounds. For example, naproxen (a non-steroidal anti-inflammatory drug) was synthesized from 74 through dehydrogenation, methylation and hydrolysis.
 |
| Scheme 16 α-Carbonyl-radical-promoted tandem cyclization of MCPs under visible-light irradiation. | |
Halogen atom transfer (XAT)47 is also an alternative means of realizing the debromination of α-carbonyl alkyl bromides. However, traditional reagents that enable XAT, which mainly contain organotin species, are usually toxic and hazardous. Therefore, “Flight from the Tyranny of Tin” has driven a quest for alternative reagents for the homolytic activation of C–halogen bonds.48 For achieving XAT, silicon reagents have become competitive players.49 Moreover, more efficient XAT processes under mild conditions have attracted the attention of researchers.50 Inspired by the report of Leonori and co-workers, our group recently developed a metal-free version of the cascade cyclization that employs the α-aminoalkyl radical as the XAT species (Scheme 17).51 In this reaction, initially, excited 4CzIPN undergoes SET oxidation with Et3N to afford the α-aminoalkyl radical 78, and subsequent XAT enables the generation of the α-carbonyl radical 80. Then, similar cascade cyclization occurs, but the final oxidation can involve SET and XAT upon visible-light irradiation. This novel transformation of MCPs features a broad substrate scope and good functional group tolerance under mild conditions.
 |
| Scheme 17 α-Carbonyl-radical-promoted tandem cyclization under metal-free conditions. | |
Radical addition to α,β-unsaturated compounds provides a convenient method to access α-carbonyl radicals, which have been used widely in visible-light-catalyzed reactions. In 2021, our group reported multiple-step catalysis to realize the tandem cyclization of MCPs tethered with acrylamide 81 (or vinyl sulfonamide) under visible-light irradiation (Scheme 19).52 Multicatalysis has conspicuously contributed to the field of catalysis in synthetic chemistry, but the complicated details remain underinvestigated.53 Clearly, governing several perfectly cooperative active catalysts in one pot without considerable entropic penalties is challenging when attempting to achieve mechanistic insight (Scheme 18). In our recent work, four catalysts – consisting of 4CzIPN, Co(dmgH)2PyCl, silane 82 (TTMSS), and quinuclidine – are used to bring about cascade catalysis upon visible-light irradiation for the synthesis of polycyclic compounds 83. We proposed a plausible mechanism through a series of mechanistic studies, including DFT calculations, radical trapping, and dark–light experiments. Under irradiation with blue light, the excited photosensitizer 4CzIPN* reduces CoIII(dmgH)2PyCl to afford CoII species. Subsequently, the oxidized photosensitizer oxidizes quinuclidine to generate the quinuclidium cation. This is a reactive HAT species that abstracts the hydrogen atom of TTMSS to generate the corresponding silyl radical 90. Addition of the silyl radical to a C–C double bond results in the α-carbonyl radical 85, which then undergoes cyclization to give the silylated intermediate 86, and intermediate 86 can be captured by CoII to form intermediate 87. The alkyl radical 88, resulting from the photolysis of 87, attacks the carbon atom connecting with the silyl unit, delivering the final product 89, upon releasing the silyl radical. The silyl radical is then trapped by CoII to afford the silyl–cobalt complex, the protonation of which regenerates CoIII and TTMSS (82) to complete the catalytic cycle. This protocol effectively synthesizes lactam- and sultam-containing polycyclic derivatives in moderate to good yields with a broad substrate scope and good functional group tolerance. In addition, this reaction can be achieved on a gram scale, and the corresponding products can be functionalized further to afford interesting compounds through hydrogenation or epoxidation.
 |
| Scheme 18 The concept of multicatalysis. | |
 |
| Scheme 19 Tandem cyclization of MCPs catalyzed by four species. | |
The generation of cyclopropylcarbinyl radicals usually needs exogenous radicals to attack the MCP group, but research on other reaction pathways, e.g., direct SET oxidation, has rarely been reported. In 2001, Beck proved the possibility of an MCP oxidation pathway via SET because MCP 13 is oxidized by ozone to afford cyclobutanone, peroxide, and ketone derivatives.54 To extend the reactivity of MCPs, in 2021, our group reported the cascade cyclization of MCPs through the direct SET oxidation the MCPs 91 by merging photocatalysis and cobalt catalysis for the synthesis of 4-aryl-1,2-dihydronaphthalene derivatives 92 and 93 (Scheme 20).55 In this report, two different oxidation pathways were proposed. In the formation of 96, MCP 13 was initially oxidized by the excited photocatalyst to radical cation 94, which was attacked by a nucleophilic fluorine anion to generate the cyclopropylcarbinyl radical intermediate 95. As for forming the hydrogen-atom-substituted product 98, we suggested an MHAT process (or HAT from a metal hydride). The CoI species was initially generated by the catalytic cycle, and it was then transformed into the CoIII–H species through combining a proton. Subsequently, an MHAT process occurred between CoIII–H and 13 to form the cyclopropylcarbinyl radical intermediate 97, regenerating the CoII species. This protocol demonstrates a robust synthetic strategy beyond photoredox catalysis to integrate single-electron oxidation and an MHAT process into MCP chemistry.
 |
| Scheme 20 Other pathways to generate cyclopropylcarbinyl radicals from MCPs. | |
Visible-light-induced synthesis of cyclopropane derivatives from MCPs
As described above, although MCPs 47 can react with TsCl under visible-light irradiation to provide cascade-cyclized42 or difunctionalized30 products, Wang's group found that if H2O is present in the reaction system, the cyclopropane ring-preserved products 100 will be obtained from MCPs 99 (Scheme 21).56 The X-ray diffraction analysis of 100 reveals that this different reaction tendency is due to intramolecular hydrogen bonding between the hydroxyl and sulfonyl groups in 100, which increases the stability. When MCPs 101, derived from acetophenones, were used without additional water, an elimination process occurred, providing the cyclopropyl styrene products 102 in moderate to good yields. The driving force might cause results that are different from the previously reported sulfonylation reactions of MCPs,8d,30,42 probably because the cyclopropylcarbinyl radical intermediate 103 is consumed rapidly through other pathways, such as SN1 nucleophilic attack by H2O or elimination via deprotonation, after SET oxidation (Scheme 21).
 |
| Scheme 21 Sulfonyl-radical-promoted cyclopropane ring-preserved reactions of MCPs. | |
In 2021, our group developed a visible-light photoredox-catalyzed intramolecular difunctionalization of MCPs to access spiro[cyclopropane-1,2-indan]ones 105 from easily prepared MCPs 104 tethered with a carboxylic acid (Scheme 22).8e If 104, DMDC (dimethyl dicarbonate), and Ir(ppy)3 are employed under the irradiation of 5 W blue LEDs, with 1,4-dioxane as the solvent, the cyclopropyl-containing cyclized products 105 were obtained in high yields. It should be noted that a base is unnecessary for the cyclization reaction, which differs from the previously reported protocol that generates acyl radicals from carboxylic acid and DMDC.57 In our reported reaction, 106 reacts with DMDC to generate 107, which can be reduced by an excited Ir catalyst to 108. Intermediate 108 is then transformed into two fragments, 109 and 110. The cyclopropyl group can be preserved in this case, which is mainly attributed to the high stabilization of the cationic intermediate 112. The cationic intermediate 112 is formed from the cyclization of 110 and the subsequent SET oxidation of 111. The two aryl groups and the cyclopropyl moiety make the cation very stable, and next SN1 attack by 109 provides 113 in excellent yield. If the cyclopropyl group adjacent to the cation is absent, control experiments indicated that the cyclization cannot proceed via the same pathway (Scheme 22, bottom). Besides DMDC, triphenylphosphine is an effective reagent for deoxygenation under visible-light irradiation,58 by which a similar cyclization reaction can proceed smoothly. In addition, product 113 can undergo nucleophilic substitution with indole or pyrrole, affording the corresponding indolyl and pyrrolyl derivatives. Moreover, product 113 can be transformed into a ring-opened product, which features a 1-indanone structural motif in the presence of TsOH.
 |
| Scheme 22 Visible-light-induced intramolecular difunctionalization of MCPs. | |
Visible-light-induced cycloaddition is also an important topic in organic synthesis. In 2021, our group reported a visible-light-induced intramolecular [2+2] cycloaddition of MCPs 114 for the construction of polysubstituted cyclic frameworks 115 (Scheme 23).59 In this reaction, the triplet excited state 117 is produced through energy transfer (EnT) between the excited state of *Ir(III) and 116, in addition to subsequent intersystem crossing (ISC). Intermediate 117 undergoes intramolecular C–C bond formation with a closed-shell carbon–carbon double bond, yielding the 1,4-triplet biradical species 118. The subsequent ISC then takes place to give a 1,4-singlet biradical species, through which subsequent C–C bond formation yields the desired [2+2] cycloaddition product 119.
 |
| Scheme 23 Visible-light-induced intramolecular [2+2] cycloaddition of MCPs. | |
Ring-opening reaction via homopropargyl radical intermediates
Most MCP reactions involving radicals depend on the classical cyclopropylcarbinyl radical clock, and other potential reaction pathways deserve to be explored, e.g., rearrangements such as β-scission of the ketiminyl radical.60 In 2021, our group reported a non-classical ring-opening radical clock reaction of MCPs 120 tethered with NHPI ester, providing a direct method to prepare a variety of alkenyl derivatives 122 (Scheme 24).61 NHPI esters have been used widely as alkyl radical precursors for photoredox or transition-metal-catalyzed coupling reactions.62 In this protocol, readily available NHPI esters 120 and radical acceptors 121 are employed as substrates for the coupling reactions. We proposed a plausible catalytic cycle based on DFT calculations, Stern–Volmer quenching, and control experiments. The initial excitation of Ru(bpy)3(PF6)2 produces excited-state *Ru(bpy)3(PF6)2, which undergoes SET oxidation with iPr2NEt or a Hantzsch ester to generate Ru(I) and a amine radical cation or Hantzsch ester radical cation. The N-(acyloxy) phthalimide 123 receives an electron from Ru(I), to transiently form the radical anion intermediate 124. Next, entropically favored decarboxylation can proceed via two plausible pathways to generate the desired radical intermediate 127, as shown in Scheme 24. In fact, the putative radical intermediate 125 or 126 will undergo a non-classical ring-opening radical clock rearrangement to afford the key intermediate 127. The addition of this nucleophilic radical to the conjugate acceptor 128 generates the stabilized radical 129, which then undergoes an SET or HAT process to form the functionalized alkyne 130 successfully. In addition, we used the products to synthesize the various synthetic intermediates 131–135 on a gram scale, which could be further transformed into functional molecules that are useful in biochemistry and medicinal chemistry. Among these molecules, the drug fingolimod can be used for the treatment of multiple sclerosis (MS), the novel tetrahydrocannabinol/anandamide (THC/AEA) hybrid ligand can significantly improve its binding affinity to the CB1 receptor in biological research, and tramadol can be used as a painkiller by serving as a weak μ-opioid receptor agonist. Besides, products 134 and 135 can be further transformed into bioactive compounds that are exceptionally active against the reverse transcriptase of HIV.
 |
| Scheme 24 Synthesizing alkenyl derivatives from MCPs under visible-light irradiation. | |
Summary
In recent years, various visible-light-induced transformations of MCPs have been developed, the majority of which enable the rapid construction of polycyclic or heterocyclic frameworks in a simple operation. The target products can be obtained selectively via different terminal pathways by regulating the reaction conditions. These transformations are being exploited towards green chemistry, such as in applying more efficient catalytic systems and metal-free conditions, and it is expected that further developments will be continuously reported in due course. Moreover, the products provided by these reactions can be further transformed into bioactive molecules used for the treatment of Multiple Sclerosis, relieving pain, inhibiting the reverse transcriptase of HIV, and so on; therefore, their synthetic applications deserve further exploration.
Author contributions
M. Shi and Y. Wei directed the perspective and revised the manuscript. H.-Z. Wei carried out the literature collection, organization, and wrote the manuscript. H.-Z. Wei drew the Schemes and checked over them.
Conflicts of interest
There are no conflicts to declare.
Acknowledgements
We are grateful for the financial support from the National Key R&D Program of China (2022YFC2303100), the National Natural Science Foundation of China (21372250, 21121062, 21302203, 20732008, 21772037, 21772226, 21861132014, 91956115 and 22171078) and the Fundamental Research Funds for the Central Universities (222201717003).
Notes and references
- K. B. Wiberg and R. A. Fenoglio, J. Am. Chem. Soc., 1968, 90, 3395–3397 CrossRef CAS.
- R. D. Bach and O. Dmitrenko, J. Am. Chem. Soc., 2004, 126, 4444–4452 CrossRef CAS PubMed.
-
(a) A. Brandi, S. Cicchi, F. M. Cordero and A. Goti, Chem. Rev., 2014, 114, 7317–7420 CrossRef CAS PubMed;
(b) L. Yu, M. Liu, F. Chen and Q. Xu, Org. Biomol. Chem., 2015, 13, 8379–8392 RSC;
(c) G. Fumagalli, S. Stanton and J. F. Bower, Chem. Rev., 2017, 117, 9404–9432 CrossRef CAS PubMed;
(d) H. Cao, F. Chen, C. Su and L. Yu, Adv. Synth. Catal., 2019, 362, 438–461 CrossRef;
(e) J. Liu, R. Liu, Y. Wei and M. Shi, Trends Chem., 2019, 1, 779–793 CrossRef CAS.
- L. Z. Yu and M. Shi, Chem. – Eur. J., 2019, 25, 7591–7606 CrossRef CAS PubMed.
-
(a) L.-Z. Yu, X.-B. Hu, Q. Xu and M. Shi, Chem. Commun., 2016, 52, 2701–2704 RSC;
(b) H.-Z. Wei, Q.-Z. Li, Y. Wei and M. Shi, Org. Biomol. Chem., 2020, 18, 7396–7400 RSC;
(c) H.-Z. Wei, L.-Z. Yu and M. Shi, Org. Biomol. Chem., 2020, 18, 135–139 RSC.
-
(a) B. Xu and M. Shi, Org. Lett., 2003, 5, 1415–1418 CrossRef CAS PubMed;
(b) L.-P. Liu and M. Shi, J. Org. Chem., 2004, 69, 2805–2808 CrossRef CAS PubMed;
(c) M. Shi, M. Jiang and L.-P. Liu, Org. Biomol. Chem., 2007, 5, 438–440 RSC;
(d) L. Ackermann, S. Kozhushkov, D. Yufit and I. Marek, Synlett, 2011, 1515–1518 CrossRef CAS.
-
(a) Y. Liu, S. Feng and X. Bao, ChemCatChem, 2018, 10, 2817–2825 CrossRef CAS;
(b) Y. Liu, A. A. Ogunlana and X. Bao, Dalton Trans., 2018, 47, 5660–5669 RSC;
(c) H. Z. Gui, Y. Wei and M. Shi, Chem. – Asian J., 2020, 15, 1225–1233 CrossRef CAS PubMed;
(d) D. Li, W. Zang, M. J. Bird, C. J. T. Hyland and M. Shi, Chem. Rev., 2020, 121, 8685–8755 CrossRef PubMed;
(e) J. Yao, Z. Chen, L. Yu, L. Lv, D. Cao and C.-J. Li, Chem. Sci., 2020, 11, 10759–10763 RSC;
(f) N. Kimura, S. Katta, Y. Kitazawa, T. Kochi and F. Kakiuchi, J. Am. Chem. Soc., 2021, 143, 4543–4549 CrossRef CAS PubMed;
(g) B. Niu, Y. Wei and M. Shi, Org. Chem. Front., 2021, 8, 3475–3501 RSC.
-
(a) Z.-Z. Zhu, K. Chen, L.-Z. Yu, X.-Y. Tang and M. Shi, Org. Lett., 2015, 17, 5994–5997 CrossRef CAS PubMed;
(b) X.-Y. Zhang, P.-H. Li and M. Shi, Asian J. Org. Chem., 2018, 7, 1924–1933 CrossRef CAS;
(c) M. Chen, Y. Wei and M. Shi, Org. Chem. Front., 2020, 7, 374–379 RSC;
(d) Y. Liu, Q.-L. Wang, Z. Chen, H. Li, B.-Q. Xiong, P.-L. Zhang and K.-W. Tang, Chem. Commun., 2020, 56, 3011–3014 RSC;
(e) H.-Z. Wei, Y. Wei and M. Shi, Org. Chem. Front., 2021, 8, 4527–4532 RSC.
- H.-Z. Wei, Y. Wei and M. Shi, Chem. Commun., 2021, 57, 11201–11204 RSC.
-
(a) J. K. Kochi, P. J. Krusic and D. R. Eaton, J. Am. Chem. Soc., 1969, 91, 1877–1879 CrossRef CAS;
(b) K. S. Chen, D. J. Edge and J. K. Kochi, J. Am. Chem. Soc., 1973, 95, 7036–7043 CrossRef CAS.
- W. J. Hehre, J. Am. Chem. Soc., 1973, 95, 2643–2646 CrossRef CAS.
-
(a) W. Zhang, F. Wang, S. D. McCann, D. Wang, P. Chen, S. S. Stahl and G. Liu, Science, 2016, 353, 1014–1018 CrossRef CAS PubMed;
(b) L. Huang, A. M. Olivares and D. J. Weix, Angew. Chem., Int. Ed., 2017, 56, 11901–11905 CrossRef CAS PubMed;
(c) W. Zhao, R. P. Wurz, J. C. Peters and G. C. Fu, J. Am. Chem. Soc., 2017, 139, 12153–12156 CrossRef CAS PubMed;
(d) Q. Fu, Z.-Y. Bo, J.-H. Ye, T. Ju, H. Huang, L.-L. Liao and D.-G. Yu, Nat. Commun., 2019, 10, 3592 CrossRef PubMed;
(e) J. H. Blackwell, R. Kumar and M. J. Gaunt, J. Am. Chem. Soc., 2021, 143, 1598–1609 CrossRef CAS PubMed.
-
(a) C. Destabel, J. D. Kilburn and J. Knight, Tetrahedron, 1994, 50, 11267–11288 CrossRef CAS;
(b) C. Destabel, J. D. Kilburn and J. Knight, Tetrahedron, 1994, 50, 11289–11302 CrossRef CAS;
(c) M. Santagostino and J. D. Kilburn, Tetrahedron Lett., 1994, 35, 8863–8866 CrossRef CAS;
(d) M. Santagostino and J. D. Kilburn, Tetrahedron Lett., 1995, 36, 1365–1368 CrossRef CAS;
(e) R. J. Boffey, M. Santagostino, J. D. Kilburn, R. J. Boffey and W. G. Whittingham, Chem. Commun., 1998, 1875–1876 RSC;
(f) K. G. Pike, C. Destabel, M. Anson and J. D. Kilburn, Tetrahedron Lett., 1998, 39, 5877–5880 CrossRef CAS;
(g) R. J. Boffey, W. G. Whittingham and J. D. Kilburn, Tetrahedron Lett., 1999, 40, 5625–5628 CrossRef CAS;
(h) D. J. Penfold, K. Pike, A. Genge, M. Anson, J. Kitteringham and J. D. Kilburn, Tetrahedron Lett., 2000, 41, 10347–10351 CrossRef CAS;
(i) F. C. Watson and J. D. Kilburn, Tetrahedron Lett., 2000, 41, 10341–10345 CrossRef CAS;
(j) R. J. Boffey, W. G. Whittingham and J. D. Kilburn, J. Chem. Soc., Perkin Trans. 1, 2001, 487–496 RSC;
(k) A. C. Saint-Dizier and J. D. Kilburn, Tetrahedron Lett., 2002, 43, 6201–6203 CrossRef CAS;
(l) L.-P. Liu and M. Shi, Chem. Commun., 2004, 2878 RSC;
(m) J. J. Underwood, G. J. Hollingworth, P. N. Horton, M. B. Hursthouse and J. D. Kilburn, Tetrahedron Lett., 2004, 45, 2223–2225 CrossRef CAS;
(n) H. Zhou, X. Huang and W. Chen, J. Org. Chem., 2004, 69, 5471–5472 CrossRef CAS PubMed;
(o) J.-W. Huang and M. Shi, J. Org. Chem., 2005, 70, 3859–3863 CrossRef CAS PubMed;
(p) X. Huang and L. Yu, Synlett, 2005, 2953–2957 CrossRef CAS;
(q) M. Shi and J.-W. Huang, J. Fluorine Chem., 2005, 126, 809–817 CrossRef CAS;
(r) X. Huang, W. Chen, H. Zhou and L. Ren, Synthesis, 2006, 609–614 CrossRef;
(s) X. Huang and L. Yu, Synlett, 2006, 2136–2138 CrossRef;
(t) X. Huang, L. Yu and M. Xie, Synlett, 2006, 0423–0426 CrossRef;
(u) W.-J. Fu and X. Huang, J. Organomet. Chem., 2007, 692, 740–745 CrossRef CAS;
(v) L. Yu, B. Chen, X. Huang and L. L. Wu, Chin. Chem. Lett., 2007, 18, 121–123 CrossRef CAS;
(w) N. Hayashi, Y. Hirokawa, I. Shibata, M. Yasuda and A. Baba, J. Am. Chem. Soc., 2008, 130, 2912–2913 CrossRef CAS PubMed;
(x) J. Sheng, C. Fan, Y. Ding, X. Fan and J. Wu, Chem. Commun., 2014, 50, 4188 RSC;
(y) K. Chen, Z.-Z. Zhu, J.-X. Liu, X.-Y. Tang, Y. Wei and M. Shi, Chem. Commun., 2016, 52, 350–353 RSC;
(z) H. Xie and B. Xu, Eur. J. Org. Chem., 2016, 2594–2598 CrossRef CAS;
(a
a) M.-T. Chen, X.-Y. Tang and M. Shi, Org. Chem. Front., 2017, 4, 86–90 RSC;
(a
b) X. Fan, L.-Z. Yu, Y. Wei and M. Shi, Org. Lett., 2017, 19, 4476–4479 CrossRef CAS PubMed;
(a
c) Y. Liu, Q.-L. Wang, C.-S. Zhou, B.-Q. Xiong, P.-L. Zhang, C.-A. Yang and K.-W. Tang, J. Org. Chem., 2017, 82, 7394–7401 CrossRef CAS PubMed;
(a
d) M. Chen, Y. Wei and M. Shi, Org. Chem. Front., 2018, 5, 2030–2034 RSC;
(a
e) Y. Liu, Q.-L. Wang, C.-S. Zhou, B.-Q. Xiong, P.-L. Zhang, C.-A. Yang and K.-W. Tang, J. Org. Chem., 2018, 83, 4657–4664 CrossRef CAS PubMed;
(a
f) C. Liu, Y.-J. Yang, J.-Y. Dong, M.-D. Zhou, L. Li and H. Wang, J. Org. Chem., 2019, 84, 9937–9945 CrossRef CAS PubMed;
(a
g) Y. Liu, Z. Chen, Q.-L. Wang, C.-S. Zhou, B.-Q. Xiong, C.-A. Yang and K.-W. Tang, J. Org. Chem., 2019, 84, 9984–9994 CrossRef CAS PubMed;
(a
h) Y. Liu, Q.-L. Wang, Z. Chen, Q. Zhou, H. Li, W.-Y. Xu, B.-Q. Xiong and K.-W. Tang, J. Org. Chem., 2019, 84, 5413–5424 CrossRef CAS PubMed;
(a
i) Y. Liu, Q.-L. Wang, Z. Chen, Q. Zhou, C.-S. Zhou, B.-Q. Xiong, P.-L. Zhang, C.-A. Yang and K.-W. Tang, Org. Biomol. Chem., 2019, 17, 1365–1369 RSC;
(a
j) T. E. Anderson and K. A. Woerpel, Org. Lett., 2020, 22, 5690–5694 CrossRef CAS PubMed;
(a
k) Y. Yuan, S. Zhang, Z. Sun, Y. Su, Q. Ma, Y. Yuan and X. Jia, Org. Lett., 2020, 22, 6294–6298 CrossRef CAS PubMed;
(a
l) Y. Su, S. Zhang, Y. Yuan, Q. Ma, Z. Sun, Y. Yuan and X. Jia, Chem. Commun., 2021, 57, 9878–9881 RSC;
(a
m) Y. Nie, L. Cheng, L. Chen, Z. Li, Y. Leng and J. Wu, Eur. J. Org. Chem., 2022, e202101406 CAS;
(a
n) Y. Kato, N. Otomura, K. Hirano and M. Miura, J. Org. Chem., 2020, 85, 5981–5994 CrossRef CAS PubMed.
- D. A. Singleton, C. C. Huval, K. M. Church and E. S. Priestley, Tetrahedron Lett., 1991, 32, 5765–5768 CrossRef CAS.
- C. Destabel, J. D. Kilburn and J. Knight, Tetrahedron Lett., 1993, 34, 3151–3154 CrossRef CAS.
- L.-Z. Yu, Q. Xu, X.-Y. Tang and M. Shi, ACS Catal., 2015, 6, 526–531 CrossRef.
-
(a) J. M. R. Narayanam and C. R. J. Stephenson, Chem. Soc. Rev., 2011, 40, 102–113 RSC;
(b) J. Xuan and W.-J. Xiao, Angew. Chem., Int. Ed., 2012, 51, 6828–6838 CrossRef CAS PubMed;
(c) C. K. Prier, D. A. Rankic and D. W. C. MacMillan, Chem. Rev., 2013, 113, 5322–5363 CrossRef CAS PubMed;
(d) N. A. Romero and D. A. Nicewicz, Chem. Rev., 2016, 116, 10075–10166 CrossRef CAS PubMed;
(e) A. Y. Chan, I. B. Perry, N. B. Bissonnette, B. F. Buksh, G. A. Edwards, L. I. Frye, O. L. Garry, M. N. Lavagnino, B. X. Li, Y. Liang, E. Mao, A. Millet, J. V. Oakley, N. L. Reed, H. A. Sakai, C. P. Seath and D. W. C. MacMillan, Chem. Rev., 2021, 122, 1485–1542 CrossRef PubMed.
-
(a) T. Okazoe, Proc. Jpn. Acad., Ser. B, 2009, 85, 276–289 CrossRef CAS PubMed;
(b) J. Gardiner, Aust. J. Chem., 2015, 68, 13–22 CrossRef CAS.
-
(a) J. Wang, M. Sánchez-Roselló, J. L. Aceña, C. del Pozo, A. E. Sorochinsky, S. Fustero, V. A. Soloshonok and H. Liu, Chem. Rev., 2013, 114, 2432–2506 CrossRef PubMed;
(b) J. Han, A. M. Remete, L. S. Dobson, L. Kiss, K. Izawa, H. Moriwaki, V. A. Soloshonok and D. O’Hagan, J. Fluorine Chem., 2020, 239, 109639 CrossRef CAS;
(c) M. Inoue, Y. Sumii and N. Shibata, ACS Omega, 2020, 5, 10633–10640 CrossRef CAS PubMed.
-
(a) T. Fujiwara and D. O’Hagan, J. Fluorine Chem., 2014, 167, 16–29 CrossRef CAS;
(b) Y. Ogawa, E. Tokunaga, O. Kobayashi, K. Hirai and N. Shibata, iScience, 2020, 23, 101467 CrossRef CAS PubMed.
-
(a) L. M. Yagupolskii, I. I. Maletina, N. V. Kondratenko and V. V. Orda, Synthesis, 1978, 835–837 CrossRef CAS;
(b) T. Umemoto, Chem. Rev., 1996, 96, 1757–1778 CrossRef CAS PubMed;
(c) R. P. Singh and J. N. M. Shreeve, Tetrahedron, 2000, 56, 7613–7632 CrossRef CAS;
(d) G. K. S. Prakash and M. Mandal, J. Fluorine Chem., 2001, 112, 123–131 CrossRef CAS;
(e) P. Eisenberger, S. Gischig and A. Togni, Chem. –
Eur. J., 2006, 12, 2579–2586 CrossRef CAS PubMed;
(f) I. Kieltsch, P. Eisenberger and A. Togni, Angew. Chem., Int. Ed., 2007, 46, 754–757 CrossRef CAS PubMed;
(g) T. Umemoto, K. Adachi and S. Ishihara, J. Org. Chem., 2007, 72, 6905–6917 CrossRef CAS PubMed;
(h) S. Noritake, N. Shibata, S. Nakamura, T. Toru and M. Shiro, Eur. J. Org. Chem., 2008, 3465–3468 CrossRef CAS;
(i) Y. Nomura, E. Tokunaga and N. Shibata, Angew. Chem., Int. Ed., 2010, 50, 1885–1889 CrossRef PubMed;
(j) C. Urban, F. Cadoret, J. C. Blazejewski and E. Magnier, Eur. J. Org. Chem., 2011, 4862–4867 CAS;
(k) H. Liu, Z. Gu and X. Jiang, Adv. Synth. Catal., 2013, 355, 617–626 CrossRef CAS;
(l) X. Liu, C. Xu, M. Wang and Q. Liu, Chem. Rev., 2014, 115, 683–730 CrossRef PubMed.
-
(a) Z. Feng, Q.-Q. Min, Y.-L. Xiao, B. Zhang and X. Zhang, Angew. Chem., Int. Ed., 2014, 53, 1669–1673 CrossRef CAS PubMed;
(b) J.-Y. Wang, Y.-M. Su, F. Yin, Y. Bao, X. Zhang, Y.-M. Xu and X.-S. Wang, Chem. Commun., 2014, 50, 4108–4111 RSC;
(c) J.-Y. Wang, X. Zhang, Y. Bao, Y.-M. Xu, X.-F. Cheng and X.-S. Wang, Org. Biomol. Chem., 2014, 12, 5582–5585 RSC;
(d) J.-W. Gu, Q.-Q. Min, L.-C. Yu and X. Zhang, Angew. Chem., Int. Ed., 2016, 55, 12270–12274 CrossRef CAS PubMed;
(e) G. Li, T. Wang, F. Fei, Y.-M. Su, Y. Li, Q. Lan and X.-S. Wang, Angew. Chem., Int. Ed., 2016, 55, 3491–3495 CrossRef CAS PubMed;
(f) Y. Wu, H.-R. Zhang, Y.-X. Cao, Q. Lan and X.-S. Wang, Org. Lett., 2016, 18, 5564–5567 CrossRef CAS PubMed;
(g) T. Xia, L. He, Y. A. Liu, J. F. Hartwig and X. Liao, Org. Lett., 2017, 19, 2610–2613 CrossRef CAS PubMed;
(h) D. Li, T. Mao, J. Huang and Q. Zhu, J. Org. Chem., 2018, 83, 10445–10452 CrossRef CAS PubMed;
(i) S. Tian, X. Song, D. Zhu and M. Wang, Adv. Synth. Catal., 2018, 360, 1414–1419 CrossRef CAS;
(j) Y. Chen, L. Li, Y. Ma and Z. Li, J. Org. Chem., 2019, 84, 5328–5338 CrossRef CAS PubMed.
-
(a) J. Lei, J. Huang and Q. Zhu, Org. Biomol. Chem., 2016, 14, 2593–2602 RSC;
(b) S. Sharma, A. P. Pandey and A. Sharma, Adv. Synth. Catal., 2020, 362, 5196–5218 CrossRef CAS.
- B. Zhang, C. Mück-Lichtenfeld, C. G. Daniliuc and A. Studer, Angew. Chem., Int. Ed., 2013, 52, 10792–10795 CrossRef CAS PubMed.
- Y.-C. Yuan, H.-L. Liu, X.-B. Hu, Y. Wei and M. Shi, Chem. – Eur. J., 2016, 22, 13059–13063 CrossRef CAS PubMed.
- S. P. Pitre, C. D. McTiernan, H. Ismaili and J. C. Scaiano, ACS Catal., 2014, 4, 2530–2535 CrossRef CAS.
-
(a) H. Togo, M. Aoki and M. Yokoyama, Tetrahedron Lett., 1991, 32, 6559–6562 CrossRef CAS;
(b) H. Togo, M. Aoki and M. Yokoyama, Tetrahedron, 1993, 49, 8241–8256 CrossRef CAS;
(c) A. De Mico, R. Margarita and G. Piancatelli, Tetrahedron Lett., 1995, 36, 3553–3556 CrossRef CAS;
(d) S. A. Moteki, A. Usui, S. Selvakumar, T. Zhang and K. Maruoka, Angew. Chem., Int. Ed., 2014, 53, 11060–11064 CrossRef CAS PubMed;
(e) H. Tan, H. Li, W. Ji and L. Wang, Angew. Chem., Int. Ed., 2015, 54, 8374–8377 CrossRef CAS PubMed;
(f) L. Wang and J. Liu, Eur. J. Org. Chem., 2016, 1813–1824 CrossRef CAS.
- J. Li, J. Chen, W. Jiao, G. Wang, Y. Li, X. Cheng and G. Li, J. Org. Chem., 2016, 81, 9992–10001 CrossRef CAS PubMed.
- D. J. Burton and D. G. Naae, J. Am. Chem. Soc., 1973, 95, 8467–8468 CrossRef CAS.
- B. Zhu, Z. Wang, H. Xi, Z. Feng, B. Wang, W. Jiao, Z. Li, Z. Wang and J. Wu, Org. Chem. Front., 2021, 8, 6300–6308 RSC.
- Q.-Y. Lin, X.-H. Xu, K. Zhang and F.-L. Qing, Angew. Chem., Int. Ed., 2015, 55, 1479–1483 CrossRef PubMed.
-
(a) Y. Fujiwara, J. A. Dixon, R. A. Rodriguez, R. D. Baxter, D. D. Dixon, M. R. Collins, D. G. Blackmond and P. S. Baran, J. Am. Chem. Soc., 2012, 134, 1494–1497 CrossRef CAS PubMed;
(b) Z. Li, Z. Cui and Z.-Q. Liu, Org. Lett., 2013, 15, 406–409 CrossRef CAS PubMed;
(c) X.-J. Tang, C. S. Thomoson and W. R. Dolbier, Org. Lett., 2014, 16, 4594–4597 CrossRef CAS PubMed;
(d) C. S. Thomoson, X.-J. Tang and W. R. Dolbier, J. Org. Chem., 2014, 80, 1264–1268 CrossRef PubMed;
(e) Z. He, P. Tan, C. Ni and J. Hu, Org. Lett., 2015, 17, 1838–1841 CrossRef CAS PubMed;
(f) X.-J. Tang and W. R. Dolbier, Angew. Chem., Int. Ed., 2015, 54, 4246–4249 CrossRef CAS PubMed;
(g) Z. Zhang, X. Tang, C. S. Thomoson and W. R. Dolbier, Org. Lett., 2015, 17, 3528–3531 CrossRef CAS PubMed.
-
(a) S. L. Rössler, B. J. Jelier, E. Magnier, G. Dagousset, E. M. Carreira and A. Togni, Angew. Chem., Int. Ed., 2020, 59, 9264–9280 CrossRef PubMed;
(b) A. A. Volkov, Chem. Heterocycl. Compd., 2020, 56, 1149–1151 CrossRef CAS;
(c) Y. N. Li, F. Xiao, Y. Guo and Y. F. Zeng, Eur. J. Org. Chem., 2021, 1215–1228 CrossRef;
(d) D. A. Shabalin, Org. Biomol. Chem., 2021, 19, 8184–8204 RSC;
(e) M. Hromadová and P. P. Lainé, Curr. Opin. Electrochem., 2022, 34, 100996 CrossRef;
(f) C.-Y. Huang, J. Li and C.-J. Li, Chem. Sci., 2022, 13, 5465–5504 RSC;
(g) Y. Wang, Y. Bao, M. Tang, Z. Ye, Z. Yuan and G. Zhu, Chem. Commun., 2022, 58, 3847–3864 RSC.
- W.-D. Liu, G.-Q. Xu, X.-Q. Hu and P.-F. Xu, Org. Lett., 2017, 19, 6288–6291 CrossRef CAS PubMed.
- O. O. Sokolova and J. F. Bower, Chem. Rev., 2020, 121, 80–109 CrossRef PubMed.
-
(a) Y. Liang, L. Jiao, Y. Wang, Y. Chen, L. Ma, J. Xu, S. Zhang and Z.-X. Yu, Org. Lett., 2006, 8, 5877–5879 CrossRef CAS PubMed;
(b) W. Li and M. Shi, Tetrahedron, 2007, 63, 11016–11020 CrossRef CAS;
(c) D. H. Zhang, Y. Wei and M. Shi, Eur. J. Org. Chem., 2011, 4940–4944 CAS.
-
(a) M. Teall, P. Oakley, T. Harrison, D. Shaw, E. Kay, J. Elliott, U. Gerhard, J. L. Castro, M. Shearman, R. G. Ball and N. N. Tsou, Bioorg. Med. Chem. Lett., 2005, 15, 2685–2688 CrossRef CAS PubMed;
(b) Y. Harrak, G. Casula, J. Basset, G. Rosell, S. Plescia, D. Raffa, M. G. Cusimano, R. Pouplana and M. D. Pujol, J. Med. Chem., 2010, 53, 6560–6571 CrossRef CAS PubMed;
(c) M. Feng, B. Tang, S. H. Liang and X. Jiang, Curr. Top. Med. Chem., 2016, 16, 1200–1216 CrossRef CAS PubMed;
(d) K. A. Scott and J. T. Njardarson, Top. Curr. Chem., 2018, 376, 5 CrossRef PubMed.
-
(a) K. Grossmann and T. Ehrhardt, Pest Manage. Sci., 2007, 63, 429–439 CrossRef CAS PubMed;
(b) Y. Noutoshi, M. Ikeda, T. Saito, H. Osada and K. Shirasu, Front. Recent Dev. Plant Sci., 2012, 3, 245 Search PubMed;
(c) W.-M. Xu, F.-F. Han, M. He, D.-Y. Hu, J. He, S. Yang and B.-A. Song, J. Agric. Food Chem., 2012, 60, 1036–1041 CrossRef CAS PubMed;
(d) P. Devendar and G.-F. Yang, Top. Curr. Chem., 2017, 375, 82 CrossRef PubMed.
-
(a) Y. Takaishi, Y. Murakami, M. Uda, T. Ohashi, N. Hamamura, M. Kidota and S. Kadota, Phytochemistry, 1997, 45, 997–1001 CrossRef CAS;
(b) J. J. Petkowski, W. Bains and S. Seager, J. Nat. Prod., 2018, 81, 423–446 CrossRef CAS PubMed;
(c) N. Wang, P. Saidhareddy and X. Jiang, Nat. Prod. Rep., 2020, 37, 246–275 RSC.
-
(a) M.-a Kakimoto, S. J. Grunzinger and T. Hayakawa, Polym. J., 2010, 42, 697–705 CrossRef CAS;
(b) H. Sasabe, Y. Seino, M. Kimura and J. Kido, Chem. Mater., 2012, 24, 1404–1406 CrossRef CAS;
(c) G. Turkoglu, M. E. Cinar and T. Ozturk, Top. Curr. Chem., 2017, 375, 84 CrossRef PubMed.
-
(a) Y. Li and Y. Fan, Synth. Commun., 2019, 49, 3227–3264 CrossRef CAS;
(b) D. Q. Dong, Q. Q. Han, S. H. Yang, J. C. Song, N. Li, Z. L. Wang and X. M. Xu, ChemistrySelect, 2020, 5, 13103–13134 CrossRef CAS.
- Y. Liu, Q.-L. Wang, Z. Chen, Q. Zhou, H. Li, C.-S. Zhou, B.-Q. Xiong, P.-L. Zhang and K.-W. Tang, J. Org. Chem., 2019, 84, 2829–2839 CrossRef CAS PubMed.
-
(a) F. Xiao, Y. Guo and Y. F. Zeng, Adv. Synth. Catal., 2020, 363, 120–143 CrossRef;
(b) I. B. Krylov, O. O. Segida, A. S. Budnikov and A. O. Terent'ev, Adv. Synth. Catal., 2021, 363, 2502–2528 CrossRef CAS;
(c) M. Latrache and N. Hoffmann, Chem. Soc. Rev., 2021, 50, 7418–7435 RSC;
(d) R. I. Patel, S. Sharma and A. Sharma, Org. Chem. Front., 2021, 8, 3166–3200 RSC.
-
(a) M. A. Dombroski, S. A. Kates and B. B. Snider, J. Am. Chem. Soc., 1990, 112, 2759–2767 CrossRef CAS;
(b) G. G. Melikyan, Synthesis, 1993, 833–850 CrossRef CAS;
(c) J. Iqbal, B. Bhatia and N. K. Nayyar, Chem. Rev., 1994, 94, 519–564 CrossRef CAS;
(d) P. I. Dalko, Tetrahedron, 1995, 51, 7579–7653 CrossRef CAS;
(e)
G. G. Melikyan, Organic Reactions, John Wiley & Sons, Inc., 1996, pp. 427–675 Search PubMed;
(f) B. B. Snider, Chem. Rev., 1996, 96, 339–364 CrossRef CAS PubMed;
(g) T. Linker, J. Prakt. Chem./Chem.-Ztg., 1997, 339, 488–492 CrossRef CAS;
(h) V. Nair, J. Mathew and J. Prabhakaran, Chem. Soc. Rev., 1997, 26, 127–132 RSC;
(i) G. A. Molander and C. R. Harris, Tetrahedron, 1998, 54, 3321–3354 CrossRef CAS;
(j) T. Sommermann, Synlett, 1999, 834 CrossRef;
(k) X.-Q. Pan, J.-P. Zou and W. Zhang, Mol. Diversity, 2009, 13, 421–438 CrossRef CAS PubMed;
(l) M. Mondal and U. Bora, RSC Adv., 2013, 3, 18716 RSC.
-
(a) X.-H. Ouyang, R.-J. Song and J.-H. Li, Chem. – Asian J., 2018, 13, 2316–2332 CrossRef CAS PubMed;
(b) K. Matsuo, T. Kondo, E. Yamaguchi and A. Itoh, Chem. Pharm. Bull., 2021, 69, 796–801 CrossRef CAS PubMed.
-
(a) A. L. Gant Kanegusuku and J. L. Roizen, Angew. Chem., Int. Ed., 2021, 60, 21116–21149 CrossRef CAS PubMed;
(b) S. Zhu, X. Zhao, H. Li and L. Chu, Chem. Soc. Rev., 2021, 50, 10836–10856 RSC;
(c) D. M. Kitcatt, S. Nicolle and A.-L. Lee, Chem. Soc. Rev., 2022, 51, 1415–1453 RSC.
- F. Juliá, T. Constantin and D. Leonori, Chem. Rev., 2021, 122, 2292–2352 CrossRef PubMed.
- P. A. Baguley and J. C. Walton, Angew. Chem., Int. Ed., 1998, 37, 3072–3082 CrossRef CAS PubMed.
-
(a) I. M. T. Davidson, Q. Rev., Chem. Soc., 1971, 25, 111 RSC;
(b) C. Chatgilialoglu, Chem. Rev., 1995, 95, 1229–1251 CrossRef CAS;
(c) C. Chatgilialoglu, C. Ferreri, Y. Landais and V. I. Timokhin, Chem. Rev., 2018, 118, 6516–6572 CrossRef CAS PubMed.
-
(a) T. Constantin, F. Juliá, N. S. Sheikh and D. Leonori, Chem. Sci., 2020, 11, 12822–12828 RSC;
(b) T. Constantin, M. Zanini, A. Regni, N. S. Sheikh, F. Juliá and D. Leonori, Science, 2020, 367, 1021–1026 CrossRef CAS PubMed;
(c) B. Górski, A.-L. Barthelemy, J. J. Douglas, F. Juliá and D. Leonori, Nat. Catal., 2021, 4, 623–630 CrossRef;
(d) H. Zhao, A. J. McMillan, T. Constantin, R. C. Mykura, F. Juliá and D. Leonori, J. Am. Chem. Soc., 2021, 143, 14806–14813 CrossRef CAS PubMed.
- B. Mao, X.-Y. Zhang, Y. Wei and M. Shi, Chem. Commun., 2022, 58, 3653–3656 RSC.
- X.-Y. Zhang, X.-Y. Wu, B. Zhang, Y. Wei and M. Shi, ACS Catal., 2021, 11, 4372–4380 CrossRef CAS.
-
(a) L. M. Ambrosini and T. H. Lambert, ChemCatChem, 2010, 2, 1373–1380 CrossRef CAS;
(b) S. P. Sancheti, Urvashi, M. P. Shah and N. T. Patil, ACS Catal., 2020, 10, 3462–3489 CrossRef CAS.
- R. F. Langler, R. K. Raheja, K. Schank and H. Beck, Helv. Chim. Acta, 2001, 84, 1943–1951 CrossRef CAS.
- J. Liu, Y. Wei and M. Shi, Org. Chem. Front., 2021, 8, 94–100 RSC.
- C. Liu, Y.-J. Yang, J.-Y. Dong, M.-D. Zhou, L. Li and H. Wang, Org. Chem. Front., 2019, 6, 3944–3949 RSC.
- G. Bergonzini, C. Cassani and C.-J. Wallentin, Angew. Chem., Int. Ed., 2015, 54, 14066–14069 CrossRef CAS PubMed.
-
(a) E. E. Stache, A. B. Ertel, T. Rovis and A. G. Doyle, ACS Catal., 2018, 8, 11134–11139 CrossRef CAS PubMed;
(b) M. Zhang, J. Xie and C. Zhu, Nat. Commun., 2018, 9, 3517 CrossRef PubMed;
(c) M. Zhang, X.-A. Yuan, C. Zhu and J. Xie, Angew. Chem., Int. Ed., 2018, 58, 312–316 CrossRef PubMed;
(d) J. I. Martinez Alvarado, A. B. Ertel, A. Stegner, E. E. Stache and A. G. Doyle, Org. Lett., 2019, 21, 9940–9944 CrossRef CAS PubMed;
(e) X.-Q. Hu, Y.-X. Hou, Z.-K. Liu and Y. Gao, Org. Chem. Front., 2020, 7, 2319–2324 RSC.
- X. Gu, Y. Wei and M. Shi, Org. Chem. Front., 2021, 8, 6823–6829 RSC.
- D. Griller and K. U. Ingold, Acc. Chem. Res., 1980, 13, 317–323 CrossRef CAS.
- X. Y. Zhang, C. Ning, B. Mao, Y. Wei and M. Shi, Chem. Sci., 2021, 12, 9088–9095 RSC.
- S. Murarka, Adv. Synth. Catal., 2018, 360, 1735–1753 CrossRef CAS.
|
This journal is © The Royal Society of Chemistry 2023 |
Click here to see how this site uses Cookies. View our privacy policy here.