DOI:
10.1039/D3CB00052D
(Review Article)
RSC Chem. Biol., 2023,
4, 548-563
Transition metal ions and neurotransmitters: coordination chemistry and implications for neurodegeneration
Received
9th April 2023
, Accepted 26th June 2023
First published on 27th June 2023
Abstract
Neurodegeneration is characterized by a disturbance in neurotransmitter-mediated signaling pathways. Recent studies have highlighted the significant role of transition metal ions, including Cu(I/II), Zn(II), and Fe(II/III), in neurotransmission, thereby making the coordination chemistry of neurotransmitters a growing field of interest in understanding signal dysfunction. This review outlines the physiological functions of transition metal ions and neurotransmitters, with the metal-binding properties of small molecule-based neurotransmitters and neuropeptides. Additionally, we discuss the structural and conformational changes of neurotransmitters induced by redox-active metal ions, such as Cu(I/II) and Fe(II/III), and briefly describe the outcomes arising from their oxidation, polymerization, and aggregation. These observations have important implications for neurodegeneration and emphasize the need for further research to develop potential therapeutic strategies.
Introduction
Signal transmission is crucial to maintain homeostasis in central and peripheral nervous systems.1–3 This process relies on neurotransmitters to regulate membrane potentials, biological cascades, or gene expression, which ultimately controls neuronal growth, differentiation, and cell proliferation.1–3 The accuracy of synaptic functions depends on the precise release of neurotransmitters in response to neural activity, which is largely regulated by biochemical signaling for efficient information processing. Although the relationship between neurotransmitter dysregulation and neurodegeneration remains a controversial topic, some studies suggest that disrupting the production, concentration, and metabolism of neurotransmitters may contribute to the development of neurodegeneration.4–8
Extensive research has been conducted on the involvement of alkali and alkaline earth metal ions in signal transmission.9,10 Na+ and K+ regulate the electrochemical gradient across neuronal membranes to generate and propagate action potentials.9 In addition, Ca2+ serves as a second messenger in the phosphoinositol pathway to mediate stimulus-responsive reactions in cells.10 Transition metal ions, such as Cu(I/II), Zn(II), and Fe(II/III), also play a crucial role in physiological systems. These metal ions function as cofactors in the active sites of metalloenzymes and assist in the structural folding of proteins.11–15 Moreover, in neurotransmission, transition metal ions facilitate the biosynthesis of neurotransmitters, coordinate with biomolecules, and even act as neurotransmitters themselves.13,16–18 The colocalization between transition metal ions and neurotransmitters has led to significant interest in their potential inter-communication and impact on neurotransmission.13,16–23 This review overviews the physiological roles of transition metal ions, their coordination with neurotransmitters, and the subsequent structural or conformational changes in neurotransmitters, with particular emphasis on their potential implications in neurodegeneration.
Physiological roles of Cu(I/II)
Copper, which exists in two major oxidation states Cu(I) and Cu(II), has multiple coordination numbers and geometries.24 As a result, Cu(I/II) can serve as a catalyst in biological systems. The possible geometries for d10 Cu(I) are two-coordinate linear, three-coordinate trigonal planar, and four-coordinate tetrahedral. In the case of d9 Cu(II), four-coordinate square planar, five-coordinate trigonal bipyramidal, and six-coordinate octahedral geometries are observed, the latter of which is often distorted by Jahn–Teller distortion effects.25 The redox ability of copper changes its electronic configuration, which makes it have different coordination modes.24,25
In biological systems, the ligand environment of static Cu(I/II) pools varies depending on enzymatic functions, such as antioxidant defense and the synthesis of neurotransmitters.26–29 The concentration of Cu(I/II) has been reported to be ranging from 60 to 110 μM in human frontal lobes and cerebellums.30 As depicted in Fig. 1a, for instance, superoxide dismutase (SOD) coordinates Cu(II) with four His residues (His46, His48, His63, and His120) to form a distorted square planar geometry.26 This structural flexibility between square planar and tetrahedral geometries [for Cu(II) and Cu(I), respectively] allows for rapid redox reactions against superoxide anion radicals (O2−˙) with a minimal reorganization energy.26,29 Copper is also found in the active site of dopamine-β-monooxygenase (DβM), which is responsible for the biosynthesis of dopamine (DA).27,28 DβM comprises two copper centers, as described in Fig. 1b: (i) one sulfur (S) donor atom and two nitrogen (N) donor atoms from Met487, His412, and His414, respectively; (ii) three N donor atoms from His262, His263, and His333.27,28 Such differences in the coordination sphere can be correlated with distinct functions at each copper center. The formal one with 2N1S coordination initiates the oxidation of DA via reducing O2 to O2−˙, while another copper center provides an additional one electron to terminate the whole reaction.27,28 While the coordination chemistry of static copper sites in metalloenzymes has been extensively studied, the existence and functions of labile copper pools have been relatively less understood.31–34 Recent studies have shown the presence of endogenous labile copper pools in cultured hippocampal neurons and retinal tissue slices, suggesting the potential role of labile copper in regulating normal neuronal functions.32,34–36 Interestingly, labile copper can also behave as a neurotransmitter modulator. For example, copper can regulate neurotransmission by acting as an antagonist of γ-aminobutyric acid (GABA) receptors upon binding to the His residues located in the extracellular domain.20,37–39
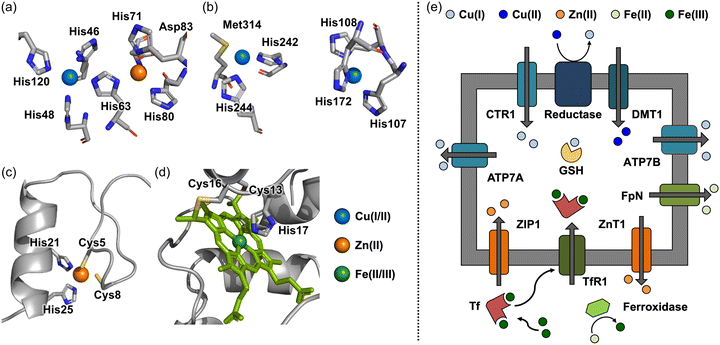 |
| Fig. 1 Coordination of metal ions found in metalloproteins and metal transport across cellular membranes. (a)–(d) Cu(II), Zn(II), and Fe(II/III) coordination found in Cu/Zn SOD (amino acid residues are numbered according to human cytoplasmic Cu/Zn SOD although the coordinates used were those of the bovine enzyme) (PDB 2SOD26), peptidylglycine α-hydroxylating monooxygenase (structural analog of DβM) (PDB 1PHM28), zinc fingers (PDB 1SP144), and cytochrome c (PDB 1C2R62). (e) Uptake and release of Cu(I/II), Zn(II), and Fe(II/III) across cellular membranes through metal transporters. Examples of metal transporters include CTR1, DMT1, ATP7A, and ATP7B for Cu(I/II) transport, ZIP1 and ZnT1 for Zn(II) transport, and TfR1 and FpN for Fe(II/III) transport. Copper, zinc, and iron are depicted in blue, orange, and green, respectively. | |
Physiological roles of Zn(II)
Zn(II) is found in biological systems (at approximately 150 μM in the brain).30 The coordination number varies from four to six with tetrahedral and octahedral geometries being the most common.40,41 Zn(II) has a closed d-shell, resulting in uniform ligand field stabilization energy (LFSE), making it thermodynamically stable even when it undergoes changes in the geometry or coordination number.42 The distinct d10 electron configuration of Zn(II) is explained for its role in the structural folding and stability of metalloproteins.42
Unlike Cu(I/II), which drives electron-transfer reactions (vide supra), Zn(II) primarily functions to organize and stabilize the structures of proteins, such as zinc finger proteins.43,44 As depicted in Fig. 1c, Zn(II) in zinc finger proteins is coordinated to four Cys or His residues in a tetrahedral geometry, forming Zn(II)(Cys)2(His)2, Zn(II)(Cys)3(His), and Zn(II)(Cys)4. It should be noted that Asp and Glu can also be part of the coordination spheres.43,44 Zn(II) binding to zinc finger proteins enables interactions with nucleic acids, which allows them to function as a transcription factor.45,46 In addition, Zn(II) can act as a Lewis acid that can activate nucleophiles.40,47,48 For instance, the Zn(II) center in carboxypeptidase A is coordinated with two N donor atoms and one O donor atom from His69, His196, and Glu72, respectively, which facilitates the deprotonation of a water molecule in the coordination sphere of carboxypeptidase A to catalytically hydrolyze amide bonds of peptides.49 In addition, Zn(II) has been reported to modulate neurotransmission, with one well-documented example being its inhibitory effect on the GABAA-activated current.38,50 Although the detailed mechanism remains unclear, it has been suggested that Zn(II) coordination to Glu137 and His141 in the α1 subunit, and Glu182, His267, and Glu270 in the β3 subunit may be involved.38,50 As a neurotransmitter itself, Zn(II) is co-released with glutamate to modulate the excitatory neurotransmission, and it is involved in learning and memory.51 For example, the lowered levels of Zn(II) can induce hippocampus-dependent memory deficits, mediated by decreasing the activation of extracellular signal-regulated kinases 1 and 2 (Erk1/2).51
Physiological roles of Fe(II/III)
Iron is the most available transition metal in biological systems (at approximately 720 μM in normal brains), and it participates in a wide range of enzymatic reactions.30,52,53 Iron can accommodate multiple oxidation states, such as Fe(II), Fe(III), and Fe(IV), under physiological conditions.54 The coordination number of Fe(II/III) is commonly observed to be six with an octahedral geometry, but three-coordinate trigonal planar, four-coordinate tetrahedral or square planar, and five-coordinate trigonal bipyramidal geometries are also found on the iron centers.55–57 As expected from various geometries and oxidation states of iron, it is utilized in a broad range of redox-mediated reactions, including oxygen transfer, electron transfer chain, and the biosynthesis of neurotransmitters.52,58 For instance, heme proteins are a prime example of iron-based electron transfer systems, where heme is the structural framework composed of iron coordinated to a porphyrin ligand with a 4N coordination mode.59 In cytochromes, heme is bound to a Cys-Xxx-Xxx-Cys-His motif, and the internal His residue is considered as an additional axial ligand with either Met or Tyr.60–62 As depicted in Fig. 1d, cytochrome c containing a heme moiety transfers an electron from complex III to complex IV, generating a proton gradient across the mitochondrial membrane and producing adenosine triphosphate (ATP).60–62 Iron–sulfur clusters represent another example of iron's use in electron transfer.58,63,64 By virtue of the redox properties of iron, thiolates are bridged to iron(s) with different ratios, producing 2Fe–2S, 3Fe–4S, and 4Fe–4S clusters that are associated with nitrogen fixation, oxidative phosphorylation, mitochondrial respiratory pathways, and ribosome assembly.58,63,64 Additionally, iron is involved in the production of neurotransmitters. In particular, the biosynthesis of catecholamine-based neurotransmitters is highly dependent on the reaction of iron-containing hydroxylase (e.g., phenylalanine hydroxylase that coordinates with Fe(II) through two His residues and one Glu residue).65 The role of iron as a neurotransmitter has not been well elucidated; however, several reports have indicated that iron is highly related to dopaminergic systems and anxiety-like behaviors.16,66,67 Moreover, ferroptosis caused by the imbalance of iron species is implicated to be the pathogenesis of neurodegeneration.16,66–71
Metal ion homeostasis
Transition metal ions, such as Cu(I/II), Zn(II), and Fe(II/III), are essential for physiological functions in the brain and neurotransmission (vide supra).13,16–23 The uptake and release of these elements are tightly regulated by metal transporters and metallochaperones, as illustrated in Fig. 1e. In this review, we briefly overview the influx and efflux of Cu(I/II), Zn(II), and Fe(II/III). Other review papers are available for detailed information.17,21,48,51,66,67,72–74
Copper uptake protein 1 (CTR1) is a representative transporter that involves almost 70% of total copper import into the brain.72 X-ray absorption spectroscopic studies revealed that the human CTR1 trimer binds two Cu(I) through three-coordinate Cu–S bonds within the Met-Xxx-Xxx-Xxx-Met motif, providing a copper channel.75,76 Intracellular copper is then appropriately delivered to target enzymes, such as cytochrome c oxidase (CcO) and SOD, by metallochaperones, including COX17 (for CcO) and CCS (for SOD), or stored in glutathione (GSH).72,77 Excess copper is removed from brain cells into the cerebrospinal fluid (CSF) followed by being stored in ATP7B for potential transport to the CSF or transported into the blood by ATP7A.72,78
Zn(II) homeostasis is maintained by several transporters, such as Zrt-, Irt-like protein (ZIP) and zinc transporter (ZnT) proteins.48,51,73 ZIP8, ZIP10, and ZIP14 are mainly linked to the uptake of Zn(II), while ZnT1 and ZnT10 manage its efflux. ZnT3 transports Zn(II) from the cytosol into synaptic vesicles.48,51,73 These transporters coordinate to Zn(II) via three oxygen (O) donor atoms and one N donor atom from three carboxylate groups in Asp and one imidazole group in His.73,79
In the case of iron, once exported from endothelial cells to the interstitial fluid through ferroportin (FpN), Fe(II) is transferred into neuronal cells through divalent metal transporter 1 (DMT1), or it is oxidized to Fe(III) by ceruloplasmin (Cp) to undergo a transferrin (Tf)-mediated pathway.53,67 Tf is composed of two domains and coordinates to two Fe(III).80 Fe(III) is coordinated to Tf through five O donor atoms from Asp63, Tyr95, Tyr188, and CO32−, and one additional N donor atom from His249, forming an octahedral geometry.80 The coordination of CO32− onto the Fe(III) center induces the conformational difference between apo-Tf and holo-Tf, which can be recognized by Tf receptor 1 (TfR1).80 Fe2Tf bound to TfR1 can be internalized via endocytosis.81 The activation of proton pumps can lower endosomal pH to around 5.6, and the reduction of Fe(III) to Fe(II) directs the dissociation of Fe(II) from Fe2Tf.81 The released Fe(II) is stored as its oxidized form, Fe(III), in ferritin for future use in biological systems.81
Small molecule-based neurotransmitters and their metal-mediated chemical transformations
Small molecule-based neurotransmitters are associated with the regulation of both physiological and behavioral processes through neurotransmission.82,83 These neurotransmitters can be derived from a single amino acid residue.83 Monoamine-based neurotransmitters synthesized from Tyr or Phe possess a distinct structure featuring an amino group linked to an aromatic ring by an ethylene linker.82,83 Interestingly, some amino acids themselves can act as neurotransmitters, such as Glu, Asp, Gly, Trp, and Phe, which are directly or indirectly involved in signaling pathways.83 This section illustrates the physiological functions of Tyr, Trp, and Phe-derived neurotransmitters that are known to interact with transition metal ions and, subsequently, undergo structural transformations.
Dopamine
Dopamine (DA) is a monoamine-based neurotransmitter categorized as a catecholamine, as presented in Fig. 2a.84 The gradual loss of DA-producing neurons in the substantia nigra is believed to contribute to Parkinson's disease (PD); thus, efforts have been made to elucidate the role of DA in neurodegenerative disorders.84–86 DA is primarily involved in four axonal pathways: nigrostriatal, mesolimbic, mesocortical, and tuberoinfundibular, initiating physiological responses for the control of movement, learning and memory, motivated behavior, attention, emotion, milk production, and lactotroph proliferation.84 Particularly in PD, however, the degeneration of dopaminergic neurons can reduce the production and release of DA, resulting in several characteristic motor symptoms, such as tremors, rigidity, bradykinesia, and balance instability.85–87
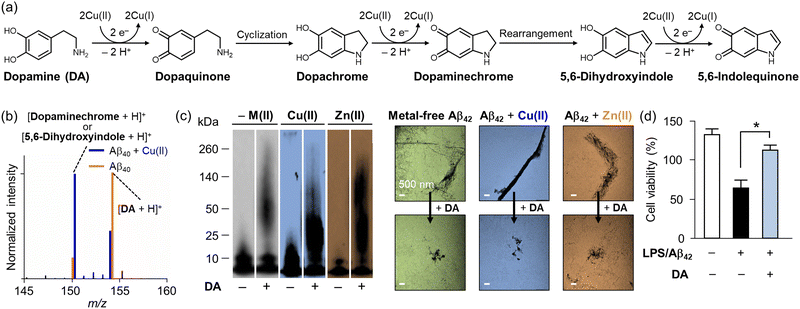 |
| Fig. 2 Impact of Cu(II) on the chemical transformation of DA with its effects on the aggregation and cytotoxicity of Aβ (a) Oxidative transformation of DA in the presence of Cu(II). (b) Analysis of DA transformation in the presence of Aβ40 with and without Cu(II) by mass spectrometry. (c) Inhibitory impact of DA on metal-free and metal-added Aβ aggregation in vitro. The size distribution and morphology of Aβ aggregates produced with and without DA and metal ions were investigated by gel/Western blot and TEM, respectively. (d) Improvement of cytotoxicity upon incubation with DA. Reproduced with permission from ref. 100 Copyright© 2018 American Chemical Society. | |
Under aerobic conditions, the catechol moiety shown in DA can be oxidized to quinone [E1/2 = 0.801 V versus standard hydrogen electrode (SHE)], as depicted in Fig. 2a.88 Waite and coworkers reported that the oxidation of DA is notably accelerated upon binding to Cu(II) via its catechol moiety.89 This copper–catechol chemistry results in electron transfer, producing Cu(I) and DA˙, with the latter reducing additional Cu(II) and transforming into dopaquinone.90 Dopaquinone readily undergoes cyclization to form dopachrome, which is oxidized to the relatively stable dopaminechrome, rearranged into 5,6-dihydroxyindole, and finally converted into 5,6-indolequinone.89 The oxidation process of DA is characterized by monitoring the absorbance at approximately 475–480 nm, indicating the presence of a dopaminechrome intermediate.89
The metal-mediated oxidation of DA summarized in Fig. 2a can induce toxicity. In detail, DA can generate reactive oxygen species (ROS) through Fenton-like reactions (redox reactions between Cu(I) and Cu(II) in the presence of DA and O2).91–93 Following similar mechanisms, Fe(II/III) can also generate quinone species and ROS via iron–catechol chemistry or Fenton chemistry.91–93 ROS can cause oxidative stress in cellular systems, resulting in lipid peroxidation and protein misfolding.93,94 Furthermore, unstable quinone intermediates, such as dopaquinone, dopaminechrome, and 5,6-indolequinone, may induce neurotoxicity via the stabilization of toxic oligomers and protofibrils of amyloidogenic proteins (e.g., α-synuclein, a pathological factor associated with PD), mitochondrial dysfunction mediated by adduct formation with complex I, III, and IV, and the disruption of proteasome and lysosomal systems by inactivating the parkin system or simply generating complexes with ubiquitin C-terminal hydrolase L1 (UCH-L1), α-tubulin, and β-tubulin.95–97 Such adduct formation was suggested to occur via Michael addition with polar side chains, such as His, Cys, and Lys.93 In addition, another DA metabolite, 3,4-dihydroxyphenylacetaldehyde (DOPAL), also stabilizes toxic oligomers and protofibrils of amyloidogenic peptides and proteins [e.g., amyloid-β (Aβ) and α-synuclein], inducing high toxicity.98
Interestingly, the neuroprotective roles of DA have continuously been reported by multiple groups.99–102 For instance, DA can alleviate inflammation and oxidative stress induced by Aβ, a pathological factor associated with Alzheimer's disease (AD).99,100 Govindaraju and coworkers suggested the neuroprotective properties of DA to mitigate Aβ-mediated toxicity by modulating its aggregation and exhibiting antioxidant activity.99 Lim and coworkers presented that DA undergoes oxidation when it is incubated with Cu(II) in both the absence and presence of Aβ.100 When DA is incubated with metal-free Aβ, the slow oxidation of DA was observed by mass spectrometry, as depicted in Fig. 2b. In the presence of Cu(II) and Aβ, however, the oxidation process of DA is facilitated.100 Notably, DA can also modulate the aggregation pathway of Aβ incubated with and without Cu(II) and Zn(II), as shown in Fig. 2c. The analysis by gel electrophoresis with Western blotting (gel/Western blot) revealed that DA could modify the aggregation of metal-free and metal-added Aβ, generating amorphous Aβ aggregates that were detected by transmission electron microscopy (TEM). Moreover, as illustrated in Fig. 2d, the treatment of DA alleviated the cytotoxicity triggered by Aβ.100 Taking account of the coordination mode of Cu(I)–Aβ complexes, His13 and His14 in Aβ have been suggested to provide a platform for Cu(I) coordination.101 The Cu(I)–Aβ complex interacts with the catechol group of DA and O2 to give a dopamine o-semiquinone radical and O2−˙.101 The dopamine o-semiquinone radical can disproportionate with a second semiquinone species, generating dopaquinone and DA.101 This process results in the oxidation of Aβ at Met35 and its covalent modification with quinone species, which alters Aβ aggregation pathways.100–102 In summary, both neurotoxic and neuroprotective effects of DA with transition metal ions have been suggested, and further research can provide a better understanding of the role of DA in neurodegeneration.
Tryptophan
Tryptophan (Trp) (Fig. 3a) is one of the 20 amino acids that make up proteins, which is not synthesized in humans but from bacteria, fungi, and plants.103 Trp is well documented that its supplementation improves sleep quality and maintenance.104,105 Although the spectroscopic evidence to support the binding of transition metal ions to Trp is very limited, DFT studies have suggested its possible coordination to divalent metal ions, such as Ni(II), Cu(II), and Zn(II), via the amine, carboxylate, and ring moieties.106,107 Interestingly, Trp undergoes several metabolic pathways under physiological conditions, generating essential neurotransmitters, such as 5-hydroxytryptamine (5-HT) and kynurenine (KYN).108–115 As illustrated in Fig. 3a, Trp can be oxidized to oxyindolylalanine, which is consecutively metabolized into N-formylkynurenine and KYN.114,115 KYN then follows a metabolic pathway, also known as the KYN pathway to generate NAD+.108–113 Such KYN pathways have been reported to modulate dopaminergic, nicotinergic, and glutaminergic neurotransmission, which may be related to numerous psychiatric and neurodegenerative symptoms.108–113,116
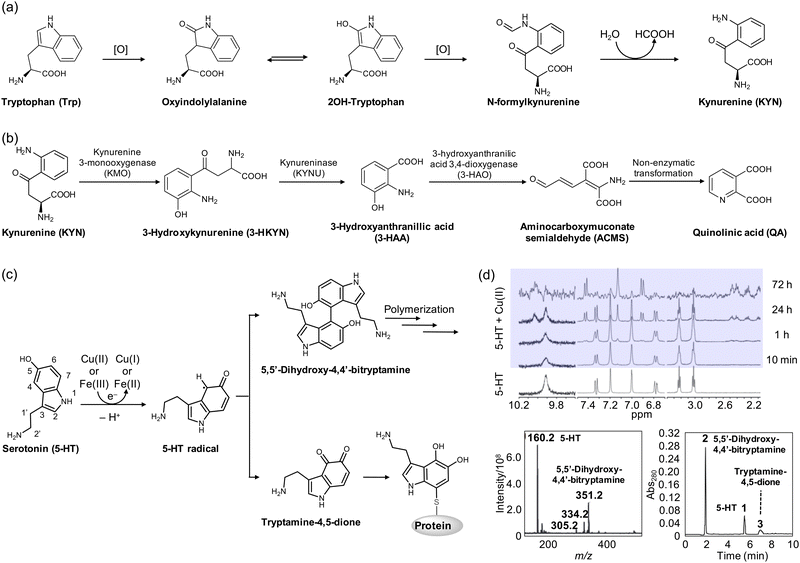 |
| Fig. 3 Structural transformations of Trp and 5-HT. (a) Metabolic pathway of Trp to KYN in the presence of ROS. (b) Metabolites from the KYN pathway. (c) Cu(I/II)-mediated oxidation and polymerization of 5-HT. (d) Oxidized products of 5-HT monitored by 1H NMR spectroscopy, mass spectrometry, and HPLC. Reproduced with permission from ref. 122 and 123 Copyright© 2007 John Wiley and Sons and 2014 Springer Nature. | |
In neurodegeneration, however, the oxidation process of Trp to KYN can be facilitated in the presence of redox-active transition metal ions producing ROS.114,115 The imbalance in the KYN concentration can accelerate the KYN pathway, upregulating the overall amount of KYN metabolites.108–115 Among various metabolites from the KYN pathway illustrated in Fig. 3b, 3-hydroxykynurenine (3-HKYN), 3-hydroxyanthranillic acid (3-HAA), and quinolinic acid (QA) have shown toxicity.108,109,112 To be specific, 3-HKYN elevates oxidative stress by blocking antioxidant catalases.108,109,112 QA, on the other hand, exhibits neurotoxicity by stimulating the release of glutamate and inhibiting the astroglial reuptake with the reduced activity of glutamine synthase.108,109,112 This elevates the extracellular concentration of glutamate and persistently activates excitatory neurons, leading to mitochondrial dysfunction, the release of cytochrome c, and the activation of proteases and caspases.108,109 Interestingly, 3-HAA and QA can generate ROS with the interactions between redox-active transition metal ions, such as Cu(I/II) and Fe(II/III), which promotes neurodegeneration through various effects, including lipid peroxidation.108–110,112
Serotonin
Serotonin, also known as 5-HT (Fig. 3c), is a monoamine-based neurotransmitter synthesized in serotonergic neurons in the central nervous system.117,118 5-HT is involved in cell growth and differentiation, smooth muscle activity, neuronal development, and signaling pathways.117,118 Due to these essential functions, it has been suggested that altered serotonergic neurotransmission may contribute to mood, motor, sensory, autonomic, cognitive, and sleep disorders that are commonly found in PD and other neurodegenerative disorders.3,119 Moreover, studies have shown that 5-HT receptors impact amyloid precursor protein processing into Aβ by activating these receptors, which increases non-amyloidogenic APP processing in vitro.120 Furthermore, the chronic treatment of selective 5-HT reuptake inhibitors, such as citalopram, reduces cerebral Aβ levels in mice, suggesting a potential therapeutic target for neurodegenerative diseases.121
In the presence of redox-active transition metal ions, which include Cu(I/II) and Fe(II/III), 5-HT is transformed through an electron-transfer process, as illustrated in Fig. 3c.122–124 At the initial stage of oxidation, 5-HT is oxidized by one electron to form a 5-HT radical intermediate, which can be detected at approximately 410 nm by electronic absorption spectroscopy.122 As depicted in Fig. 3d, the treatment of Cu(II) to 5-HT for 24 h resulted in the appearance of new peaks at 6.9, 7.1, and 7.5 ppm in 1H nuclear magnetic resonance (NMR) spectra.122 In particular, the singlet peak at 7.1 ppm suggested that only one proton exists, derived from either C2 or C4. The disappearance of the 1.9 Hz coupling between C4 and C6 clarified the dimerization of 5-HT via coupling of two species at the C4 position.122 The dehydrogenative coupling of two 5-HT molecules was also supported by mass spectrometry.122 Heme–Aβ complexes have been found to induce the same dimerization of the 5-HT radical intermediate, as observed by electronic absorption spectroscopy.123 Alternatively, the 5-HT radical intermediate can be oxidized to tryptamine-4,5-dione. As shown in Fig. 3d, investigations by high performance liquid chromatography (HPLC) showed a minor peak (peak 3), assigned to be tryptamine-4,5-dione produced by the oxidation of the 5-HT radical.123 Moreover, the 5-HT radical intermediate may subsequently form covalent adducts with thiol groups of proteins and enzymes, including tryptophan hydroxylase.125–127 This can direct the irreversible loss of function. While the impact of tryptamine-4,5-dione on cytotoxicity is still a topic of debate, some studies have reported that it may upregulate the mRNA and protein expression of NAD(P)H quinone dehydrogenase 1 (NQO1) and heme oxygenase-1 (HO-1) responsible for relieving the cytotoxicity induced by hydrogen peroxide (H2O2).128,129 The transformation of 5-HT in the presence of redox-active transition metal ions can lead to diverse outcomes that require further investigation.
Epinephrine
Epinephrine, also known as adrenaline, plays a key role in physiological muscle contraction, especially in the vascular smooth muscle and intestinal sphincter muscle, upon binding to α1 and α2 adrenergic receptors.130 The activation of β1 receptors by epinephrine contributes to the increase in heart rate, myocardial contractility, and renin release, while binding to β2 receptors provokes bronchodilation and glucose metabolism.130 Chronic stress can alter the level of epinephrine, disrupting the regulation of the stress response and potentially contributing to pathological conditions, such as cancer, cardiovascular dysfunction, and neurodegeneration.131 Thus, maintaining the proper levels of epinephrine is crucial for overall health and well-being.
The involvement of epinephrine's oxidation in neurodegeneration has received attention as a major contributing factor.132,133 The oxidation of catecholamines at physiological pH is generally very slow; however, it can be facilitated by enzymatic or metal-mediated catalysis.134 As illustrated in Fig. 4a, copper–catechol chemistry is involved in the oxidation process, where epinephrine reduces two Cu(II) to generate epinephrinequinone and two Cu(I).132,135 The structural instability of epinephrinequinone makes it readily cyclized to form leucoepinephrinechrome, or it can coordinate to GSH. Leucoepinephrinechrome, retaining the catechol moiety, undergoes another round of electron-transfer process, leading to epinephrinechrome, which can fall into three different fates: a reverse reaction to leucoepinephrinechrome accompanied by ROS generation, polymerization, or coordination to a thiol moiety of enzymes.132,135 In particular, the adduct formation between epinephrinequinone and GSH was observed to result in the overall depletion of GSH concentration, causing noxious effects on cellular function and defense.132 Moreover, 5-(glutathione-S-yl)epinephrine has been reported to induce toxicity by activating caspase-3 or oxidizing DNA bases.136 Rael and coworkers presented that chelating out copper by D-Asp–D-Ala–D-His–D-Lys (D-DAHK) could exert a protective effect from oxidative stress induced by the copper–epinephrine interaction,134 suggesting that redox-active metal ions are highly related to cytotoxicity in the progression of neurodegeneration.
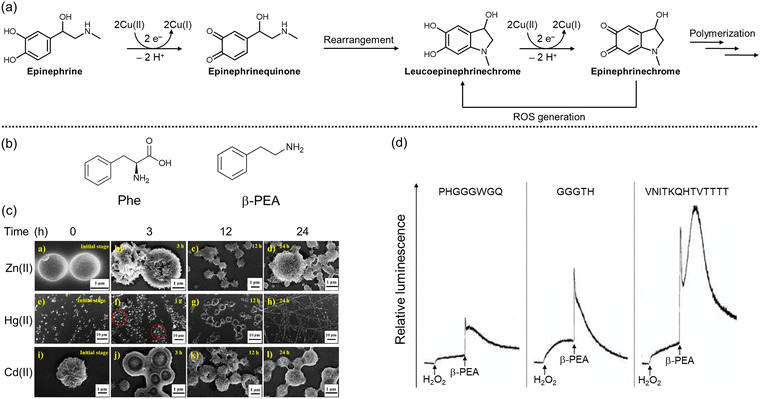 |
| Fig. 4 Structural changes of epinephrine as well as fibrilization and ROS generation of Phe and β-PEA in the presence of divalent metal ions. (a) Oxidation and polymerization of epinephrine in the presence of Cu(II). (b) Chemical structures of Phe and β-PEA. (c) Aggregation of Phe into fibrillar species in the presence of divalent metal ions, including Zn(II), Cd(II), and Hg(II). Reproduced with permission from ref. 141. Copyright© 2022 American Chemical Society. (d) Increased levels of O2−˙ by incubation of β-PEA, Cu(II), and fragments of the prion protein. Reproduced with permission from ref. 142. Copyright© 2006 Ivyspring International Publisher. | |
Phenylalanine & phenethylamine
Phenylalanine (Phe) (Fig. 4b) can be obtained from various food sources, including meat, wheat, and milk products.137 As a neurotransmitter, Phe is associated with controlling chronic pain, depression, and other disorders.137 Moreover, Phe is a precursor to monoamine-based neurotransmitters like DA and 5-HT, making its cellular levels indirectly related to signaling pathways.138 Interestingly, Phe can be decarboxylated by phenylalanine decarboxylase to generate phenethylamine (β-PEA; Fig. 4b),138 the specific role of which has not been fully elucidated. Several studies, however, suggest that β-PEA is involved in dopaminergic transmission.139,140 β-PEA inhibits the influx of DA, 5-HT, and norepinephrine by binding to amine-associated receptor 1,139 while the interaction with the DA receptor stimulates the release of DA in striatal brain slices.140
In the presence of metal ions, however, the aforementioned functions of Phe and β-PEA as neurotransmitters begin to diminish, and toxicity arises.141–144 In the case of Phe, Chakraborty and coworkers presented that divalent and trivalent metal ions, including Zn(II), Cd(II), Hg(II), Al(III), Ga(III), and In(III), could induce the self-aggregation of Phe.141 The impact of metal ions on Phe aggregation was investigated by confocal laser scanning microscopy and field emission scanning electron microscopy, as shown in Fig. 4c. It should be noted that the production of fibrillar species was monitored by the thioflavin-T (ThT) that is broadly used for quantitative analysis of β-sheet structures.145 In the presence of divalent metal ions, monomeric Phe rapidly generated microspheres that were fused into vesicular structures and transformed into mature fibrils over time. In particular, in the presence of Zn(II), the fibrils elongated in a dendritic fashion, orienting in one common center.141 On the other hand, β-PEA could increase the level of O2−˙ upon incubation with copper and copper-binding fragments of the prion protein, as presented in Fig. 4d.142 Alternatively, even in the absence of metal ions, β-PEA could aggravate oxidative stress by inhibiting the activity of NQO1 as well as mitochondrial complex I and complex III.143,144
Peptide-based neurotransmitters and their conformational and functional changes mediated by metal ions
Peptide-based neurotransmitters, referred to as neuropeptides, are involved in neuronal signaling associated with analgesia, reward, food intake, metabolism, social behaviors, learning, and memory.146 Recent advances in biotechnologies coupled with solid-phase peptide synthesis have opened up new avenues for developing peptide-based medicines, which are being actively explored in the therapeutic field against neurodegeneration.147 Notably, several studies have shown that transition metal ions interact with neuropeptides and distinctively affect signaling pathways, indicating the importance of elucidating how metal coordination influences the physiological and pathological functions involved in neurodegeneration caused by metal ion dyshomeostasis.148–154 Such a comprehensive understanding can pave the way for novel therapeutic strategies for neurodegeneration. The following section covers recent studies on the coordination chemistry of transition metal ions with neuropeptides, particularly focusing on those containing a disulfide bond: somatostatin (SST), vasopressin (AVP), and oxytocin (Oxt).
Somatostatin
Somatostatin (SST; Fig. 5a) is a naturally occurring neuropeptide composed of 14 amino acid residues (AGCKNFFWKTFTSC) with an intramolecular disulfide bond between Cys3 and Cys14.155 SST plays a vital role in nervous systems despite its short half-life (around 3 min), inhibiting the secretion of growth hormone (GH) mediated by the growth hormone-releasing hormone (GHRH) from anterior pituitary somatotrophs.156 In obese patients, GH secretion may be affected, with the subsequent responsivity of SST for suppressing insulin and glucagon.157 SST can also inhibit adenyl cyclase and Ca(II) channels, leading to the inhibition of cell proliferation,157 as well as olfactory systems.158,159 As the homeostasis of SST is impaired, it may behave differently, however.160,161 In PD patients, the concentration of SST decreases in frontal and temporal cortices, and its colocalization with Lewy bodies is observed, which is thought to be a cause of the disease impacting olfactory systems and nonmotor symptoms, such as tiredness, depression, and pain.162 In the case of AD, SST has been reported to specifically bind toxic Aβ42 oligomers.163,164
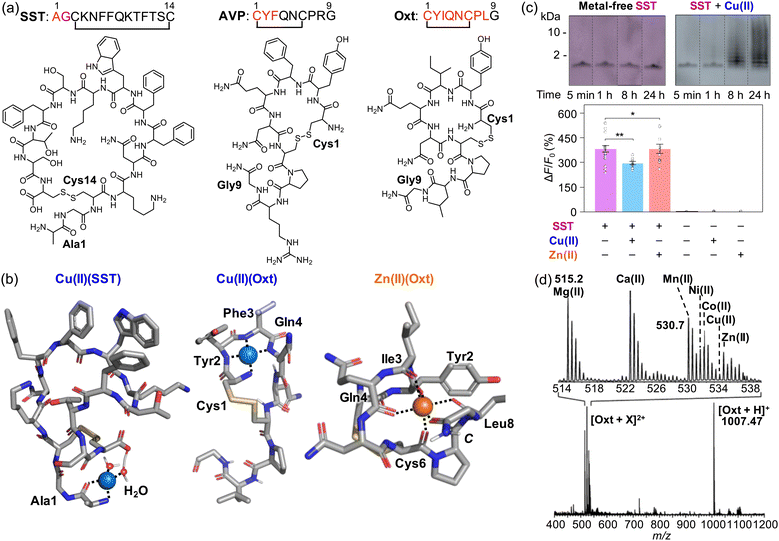 |
| Fig. 5 Interactions of SST, AVP, and Oxt with metal ions. (a) Amino acid sequences and chemical structures of SST, AVP, and Oxt. (b) Potential metal binding to SST and Oxt.148,152,153 (c) Aggregation and change in the receptor binding of SST in the presence of Cu(II). Reproduced with permission from ref. 148. Copyright© 2022 Springer Nature. (d) Adduct formation between Oxt and various divalent metal ions, including Mg(II), Ca(II), Mn(II), Ni(II), Co(II), Cu(II), and Zn(II). Reproduced with permission from ref. 152. Copyright© 2008 American Chemical Society. | |
Recently, Lim and coworkers have demonstrated that the presence of Cu(II) could result in the loss of function of SST as a neurotransmitter.148 Cu(II) was observed to coordinate to the N-terminal primary amine, the backbone carbonyl group between Ala1 and Gly2, and two water molecules, one of which is deprotonated by the C-terminal carboxylate group, as illustrated in Fig. 5b.148 Cu(II) binding to SST could partially fold the N-terminal region of the peptide, which may be a driving force for its self-assembly via hydrophobic interactions, antiparallel β-sheet formation, or the Cu(II)(SST)2 generation. It should be noted that other Cu(II)-binding sites, in addition to the N-terminal region, have also been suggested within the sidechain of Phe6 and Phe7 via cation–π interactions.165
The formation of SST aggregates upon incubation with Cu(II) was detected by the gel/Western blot, as shown in Fig. 5c.148 Moreover, SST species produced with Cu(II) were shown to have less binding properties to the receptor, confirmed by the studies employing the GPCR-activation-based sensor (GRABSST).148 It should be noted that such conformational and functional changes of SST were not observed in the presence of Zn(II).148 These findings suggested that Cu(II) interaction could cause the aggregation of SST and, consequently, inhibit its receptor binding properties critical for signaling.148 When SST was incubated with Aβ in both the absence and presence of Cu(II) and Zn(II), the aggregation of SST into oligomers was also observed, resulting in reduced interactions against cell membranes.148 The co-incubation between metal ions and SST has been shown to mitigate the cytotoxicity mediated by Aβ.148 Overall, these results and observations suggest the alteration of the structure and activity of SST under pathological conditions and provide broader insight into the new role of neuropeptides in the pathologies of neurodegenerative diseases.
Vasopressin
Vasopressin (Fig. 5a), also known as arginine vasopressin (AVP), is a nonapeptide neurotransmitter (CYFQNCPRG) that forms a cyclic structure through a disulfide bond between Cys1 and Cys6.166 AVP is importantly linked to osmoregulation.166–169 It is also involved in increasing the mean arterial blood pressure by binding to vasopressin type 1 (V1) receptors, which mediates vasoconstriction in the skin, skeletal muscle, and mesenteric blood vessels.166,170,171 Furthermore, AVP binding to V3 receptors can control thermoregulation, cognition, memory, and behavior regulation.172,173 The expression levels of AVP are decreased in patients with neurodegenerative disorders, such as Huntington's disease (HD).174 Interestingly, AVP(4-8) has been shown to improve cognitive function in an AD mouse model,175 suggesting the involvement of AVP metabolites in neurodegeneration.
An AVP mutant in which the side chain of Phe3 is substituted with naphthalene (Nal) has been found to bind divalent metal ions, including Cu(II), Zn(II), and Mn(II).149 A different AVP mutant with an additional Ala residue at the N-terminus has demonstrated the same result.150 Metal coordination to AVP could stabilize its active structure and potentiate its function.176 Cu(II)-binding residues in AVP have been proposed through CD, electron paramagnetic resonance, and electronic absorption spectroscopies.150 Cu(II) was observed to coordinate with four N donor atoms, including one from the N-terminal primary amine and three from the amide backbones between Cys1/Tyr2, Tyr2/Phe3, and Phe3/Gln4.151 Cu(II) coordination to AVP has been suggested to constrain the receptor-binding residues (e.g., Tyr2 and Phe3), which may alter its contractile activity;151 however, the exact mechanism is still unclear.
Oxytocin
Oxytocin (Oxt; Fig. 5a) is a nonapeptide (CYIQNCPLG) with a cyclic structure formed by the disulfide bond formation between Cys1 and Cys6.177 Notably, the sequence of Oxt resembles that of AVP, differing only in two amino acid residues (i.e., Ile3 and Leu8). Oxt plays diverse physiological roles in lactation, parturition, memory, recognition, affiliation, aggression, learning, stress, and depression.177 In addition, Oxt is also involved in cell proliferation, slowing the heart rate, and myoblast fusion with myotubule formation.178 Several studies have suggested that Oxt could have a neuroprotective role in neurodegeneration, as it could promote anti-inflammation, reduce ROS generation, and diminish pro-inflammation cascades in microglia.179–181 Such effects were also observed in the tMCAO stroke rat model.182
The interactions between Oxt and transition metal ions are an area of interest in bioinorganic chemistry.152–154 The coordination of Oxt to divalent metal ions, including Mg(II), Ca(II), Mn(II), Ni(II), Co(II), Cu(II), and Zn(II), has been identified, as represented in Fig. 5d.152 Bowers and coworkers reported two different binding modes of Cu(II) to Oxt by computational analysis (Fig. 5b).152 Both modes include the primary amine at the N-terminus and two N donor atoms from the amide backbones between Pro7/Leu8 and Leu8/Gly9. A fourth ligand differs depending on the mode. It is either one N donor atom from the amide backbone between Asn5 and Cys6 or the amidated C-terminus.152 Recent paramagnetic enhancement NMR studies reported another 4N coordination mode of Cu(II)(Oxt).153 Hurevich and coworkers illustrated the coordination sphere in Oxt composed of the N-terminal primary amine and three N donor atoms from amide backbones, including one between Cys1 and Tyr2 and two consecutive (Fig. 5b).153 The effect of Cu(II) complexation with Oxt on its receptor binding has not yet been fully elucidated, however. The disulfide bond in Oxt may be cleaved in the presence of ROS,154 which can affect normal functions of cyclic peptides (vide supra).37,72
In the case of Zn(II), computational studies showed two types of coordination modes to Oxt composed of either six or five O donor atoms.152 As depicted in Fig. 5b, the coordination sphere in a quasi-octahedral geometry contains the carbonyl group from the amidated C-terminus and the amide backbones between Tyr2/Ile3, Ile3/Gln4, Gln4/Asn5, Cys6/Pro7, and Leu8/Gly9.152 Another Zn(II)-binding mode is in a quasi-trigonal bipyramidal structure with the 1N4O coordination: the N donor atom from the N-terminal primary amine and four O donor atoms from the carbonyl groups of the amidated C-terminus and the amide backbones between Cys1/Tyr2, Gln4/Asn5, and Cys6/Pro7.152 Zn(II) binding of Oxt can align Ile3, Gln4, and Asn5 in the same plane, three of which are essential for the interaction with a hydrophobic pocket on Oxt receptors. Such conformational rigidity attributed to the complexation between Zn(II) and Oxt can facilitate the hormone–receptor binding.152
Conclusions and future perspective
Neurotransmitters are the fundamental building blocks of neuronal signaling pathways, which regulate the intricate homeostasis of our body system.13,16–18 Their vital roles in maintaining normal physiological functions have been documented in the literature.11–15 The binding of transition metal ions to neurotransmitters, however, can potentially disrupt their normal functions, thereby leading to the development of various neurodegenerative diseases, such as AD, PD, amyotrophic lateral sclerosis, and HD.100,127,142,147,148,154 Nonetheless, some interactions between metal ions and neurotransmitters have been reported to ameliorate the processes associated with neurodegeneration.100–102 In this review, we briefly introduce small molecule-based neurotransmitters and peptide-based neurotransmitters and summarize their metal-binding properties. Transition metal ions, such as Cu(I/II), Zn(II), and Fe(II/III), can directly or indirectly interfere with normal functions of neurotransmitters through multiple mechanisms, including coordination, conformational or structural changes, and ROS generation. It should be noted that other various metalloproteins exist in physiological systems exhibiting different functions,11–15 which was not covered in this review.
Despite significant progress in understanding the pathology of neurodegenerative diseases, the precise interplay between transition metal ions and neurotransmitters remains unclear.100,127,142,147,148,154 This highlights the urgent need for detailed investigations into the coordination chemistry of neurotransmitters. Such studies are expected to yield crucial insights into the influence of transition metal ions on the functions of neurotransmitters, thereby paving the way for the development of novel therapeutic strategies in the field of neurodegeneration. Thus, we anticipate that future research will focus on identifying new therapeutic targets and potential drug candidates against the inter-communication between transition metal ions and neurotransmitters.
Conflicts of interest
The authors declare no competing financial interests.
Acknowledgements
This work was supported by the National Research Foundation of Korea (NRF) grant funded by the Korean government [NRF-2022R1A3B1077319 (M. H. L.)] and the 2023 Research Fund of the University of Seoul (J. H.).
References
- D. A. Berg, L. Belnoue, H. Song and A. Simon, Neurotransmitter-mediated control of neurogenesis in the adult vertebrate brain, Development, 2013, 140, 2548–2561 CrossRef CAS PubMed.
- A. E. Pereda, Electrical synapses and their functional interactions with chemical synapses, Nat. Rev. Neurosci., 2014, 15, 250–263 CrossRef CAS PubMed.
- R. I. Teleanu, A. G. Niculescu, E. Roza, O. Vladâcenco, A. M. Grumezescu and D. M. Teleanu, Neurotransmitters-Key factors in neurological and neurodegenerative disorders of the central nervous system, Int. J. Mol. Sci., 2022, 23, 5954 CrossRef CAS PubMed.
-
J. Moini, J. Koenitzer and A. LoGalbo, Chapter 2—Brain neurotransmitters, Global Emergency of Mental Disorders, Academic Press, Cambridge, MA, U. S. A., 2021 Search PubMed.
- T. Hanada, Ionotropic glutamate receptors in epilepsy: A review focusing on AMPA and NMDA receptors, Biomolecules, 2020, 10, 464 CrossRef CAS PubMed.
- S. Banerjee, S. McCracken, M. F. Hossain and G. Slaughter, Electrochemical detection of neurotransmitters, Biosensors, 2020, 10, 101 CrossRef CAS.
- S. Bhat, A. El-Kasaby, M. Freissmuth and S. Sucic, Functional and biochemical consequences of disease variants in neurotransmitter transporters: A special emphasis on folding and trafficking deficits, Pharmacol. Ther., 2021, 225, 107816 CrossRef CAS PubMed.
- D. Yadav and P. Kumar, Restoration and targeting of aberrant neurotransmitters in Parkinson's disease therapeutics, Neurochem. Int., 2022, 156, 105327 CrossRef CAS PubMed.
- M. W. Barnett and P. M. Larkman, The action potential, Pract. Neurol., 2007, 7, 192–197 Search PubMed.
- M. Endo, Calcium ion as a second messenger with special reference to excitation-contraction coupling, J. Pharmacol. Sci., 2006, 100, 519–524 CrossRef CAS PubMed.
- M. G. Savelieff, G. Nam, J. Kang, H. J. Lee, M. Lee and M. H. Lim, Development of multifunctional molecules as potential therapeutic candidates for Alzheimer's disease, Parkinson's disease, and amyotrophic lateral sclerosis in the last decade, Chem. Rev., 2019, 119, 1221–1322 CrossRef CAS PubMed.
- K. P. Kepp, Bioinorganic chemistry of Alzheimer's disease, Chem. Rev., 2012, 112, 5193–5239 CrossRef CAS PubMed.
- C. M. Opazo, M. A. Greenough and A. I. Bush, Copper: From neurotransmission to neuroproteostasis, Front. Aging Neurosci., 2014, 6, 143 Search PubMed.
- C. Livingstone, Zinc: Physiology, deficiency, and parenteral nutrition, Nutr. Clin. Pract., 2015, 30, 371–382 CrossRef CAS PubMed.
- J. Kaplan and D. M. Ward, The essential nature of iron usage and regulation, Curr. Biol., 2013, 23, 2325 CrossRef CAS PubMed.
- D. Hare, S. Ayton, A. Bush and P. Lei, A delicate balance: Iron metabolism and diseases of the brain, Front. Aging Neurosci., 2013, 5, 34 Search PubMed.
- K. Tóth, Zinc in neurotransmission, Annu. Rev. Nutr., 2011, 31, 139–153 CrossRef PubMed.
- C. Marchetti, Interaction of metal ions with neurotransmitter receptors and potential role in neurodiseases, Biometals, 2014, 27, 1097–1113 CrossRef CAS PubMed.
- K. Acevedo, S. Masaldan, C. M. Opazo and A. I. Bush, Redox active metals in neurodegenerative diseases, J. Biol. Inorg. Chem., 2019, 24, 1141–1157 CrossRef CAS PubMed.
- M. Kawahara, K.-I. Tanaka and M. Kato-Negishi, Copper as a collaborative partner of zinc-induced neurotoxicity in the pathogenesis of vascular dementia, Int. J. Mol. Sci., 2021, 22, 7242 CrossRef CAS PubMed.
- K. Krzywoszyńska, D. Witkowska, J. Światek-Kozlowska, A. Szebesczyk and H. Kozłowski, General aspects of metal ions as signaling agents in health and disease, Biomolecules, 2020, 10, 1417 CrossRef PubMed.
- C. J. Chang, Bioinorganic life and neural activity: Toward a chemistry of consciousness?, Acc. Chem. Res., 2017, 50, 535–538 CrossRef CAS PubMed.
- R. C. Balachandran, S. Mukhopadhyay, D. McBride, J. Veevers, F. E. Harrison, M. Aschner, E. N. Haynes and A. B. Bowman, Brain manganese and the balance between essential roles and neurotoxicity, J. Biol. Chem., 2020, 295, 6312–6329 CrossRef CAS PubMed.
-
R. R. Conry, Encyclopedia of Inorganic Chemistry, ed. R. B. King, R. H. Crabtree, C. M. Lukehart, D. A. Atwood and R. A. Scott, John Wiley & Sons, 2006 Search PubMed.
- B. J. Charette, P. J. Griffin, C. M. Zimmerman and L. Olshansky, Conformationally dynamic copper coordination complexes, Dalton Trans., 2022, 51, 6212–6219 RSC.
- A. F. Miller, Superoxide dismutases: Active sites that save, but a protein that kills, Curr. Opin. Chem. Biol., 2004, 8, 162–168 CrossRef CAS PubMed.
- T. V. Vendelboe, P. Harris, Y. Zhao, T. S. Walter, K. Harlos, K. El Omari and H. E. Christensen, The crystal structure of human dopamine β-hydroxylase at 2.9 A resolution, Sci. Adv., 2016, 2, e1500980 CrossRef PubMed.
- S. Maheshwari, C. Shimokawa, K. Rudzka, C. D. Kline, B. A. Eipper, R. E. Mains, S. B. Gabelli, N. Blackburn and L. M. Amzel, Effects of copper occupancy on the conformational landscape of peptidylglycine α-hydroxylating monooxygenase, Commun. Biol., 2018, 1, 74 CrossRef PubMed.
- B. G. Trist, J. B. Hilton, D. J. Hare, P. J. Crouch and K. L. Double, Superoxide dismutase 1 in health and disease: How a frontline antioxidant becomes neurotoxic, Angew. Chem., Int. Ed., 2021, 60, 9215–9246 CrossRef CAS PubMed.
- L. Wang, Y.-L. Yin, X.-Z. Liu, P. Shen, Y.-G. Zheng, X.-R. Lan, C.-B. Lu and J.-Z. Wang, Current understanding of metal ions in the pathogenesis of Alzheimer's disease, Transl. Neurodegener., 2020, 9, 10 CrossRef PubMed.
- C. Shen and E. J. New, What has fluorescent sensing told us about copper and brain malfunction?, Metallomics, 2015, 7, 56–65 CrossRef CAS PubMed.
- S. C. Dodani, A. Firl, J. Chan, C. I. Nam, A. T. Aron, C. S. Onak, K. M. Ramos-Torres, J. Paek, C. M. Webster, M. B. Feller and C. J. Chang, Copper is an endogenous modulator of neural circuit spontaneous activity, Proc. Natl. Acad. Sci. U. S. A., 2014, 111, 16280–16285 CrossRef CAS PubMed.
- C. S. Lim, J. H. Han, C. W. Kim, M. Y. Kang, D. W. Kang and B. R. Cho, A copper(I)-ion selective two-photon fluorescent probe for in vivo imaging, Chem. Commun., 2011, 47, 7146–7148 RSC.
- E. Falcone, M. Okafor, N. Vitale, L. Raibaut, A. Sour and P. Faller, Extracellular Cu2+ pools and their detection: From current knowledge to next-generation probes, Coord. Chem. Rev., 2021, 433, 213727 CrossRef CAS.
- A. T. Aron, K. M. Ramos-Torres, J. A. Cotruvo, Jr. and C. J. Chang, Recognition- and reactivity-based fluorescent probes for studying transition metal signaling in living systems, Acc. Chem. Res., 2015, 48, 2434–2442 CrossRef CAS PubMed.
- J. A. Cotruvo, Jr., A. T. Aron, K. M. Ramos-Torres and C. J. Chang, Synthetic fluorescent probes for studying copper in biological systems, Chem. Soc. Rev., 2015, 44, 4400–4414 RSC.
- M. Han, J. Chang and J. Kim, Loss of divalent metal transporter 1 function promotes brain copper accumulation and increases impulsivity, J. Neurochem., 2016, 138, 918–928 CrossRef CAS PubMed.
- I. N. Sharonova, V. S. Vorobjev and H. L. Haas, Interaction between copper and zinc at GABAA receptors in acutely isolated cerebellar Purkinje cells of the rat, Br. J. Pharmacol., 2000, 130, 851–856 CrossRef CAS PubMed.
- H. Kim and R. L. Macdonald, An N-terminal histidine is the primary determinant of α subunit-dependent Cu2+ sensitivity of αβ3γ2L GABAA receptors, Mol. Pharmacol., 2003, 64, 1145–1152 CrossRef CAS PubMed.
- N. J. Ataie, Q. Q. Hoang, M. P. Zahniser, Y. Tu, A. Milne, G. A. Petsko and D. Ringe, Zinc coordination geometry and ligand binding affinity: The structural and kinetic analysis of the second-shell serine 228 residue and the methionine 180 residue of the aminopeptidase from Vibrio proteolyticus, Biochemistry, 2008, 47, 7673–7683 CrossRef CAS PubMed.
- M. Dudev, J. Wang, T. Dudev and C. Lim, Factors governing the metal coordination number in metal complexes from Cambridge Structural Database analyses, J. Phys. Chem. B, 2006, 110, 1889–1895 CrossRef CAS PubMed.
- M. J. Lachenmann, J. E. Ladbury, J. Dong, K. Huang, P. Carey and M. A. Weiss, Why zinc fingers prefer zinc: Ligand-field symmetry and the hidden thermodynamics of metal ion selectivity, Biochemistry, 2004, 43, 13910–13925 CrossRef CAS PubMed.
- K. Kluska, J. Adamczyk and A. Krężel, Metal binding properties, stability and reactivity of zinc fingers, Coord. Chem. Rev., 2018, 367, 18–64 CrossRef CAS.
- V. A. Narayan, R. W. Kriwacki and J. P. Caradonna, Structures of zinc finger domains from transcription factor Sp1. Insights into sequence-specific protein–DNA recognition, J. Biol. Chem., 1997, 272, 7801–7809 CrossRef CAS PubMed.
- A. Klug, The discovery of zinc fingers and their applications in gene regulation and genome manipulation, Annu. Rev. Biochem., 2010, 79, 213–231 CrossRef CAS PubMed.
- S. J. Lee and S. L. Michel, Structural metal sites in nonclassical zinc finger proteins involved in transcriptional and translational regulation, Acc. Chem. Res., 2014, 47, 2643–2650 CrossRef CAS PubMed.
- M. Hernick and C. A. Fierke, Zinc hydrolases: The mechanisms of zinc-dependent deacetylases, Arch. Biochem. Biophys., 2005, 433, 71–84 CrossRef CAS PubMed.
- A. Takeda, Zinc homeostasis and functions of zinc in the brain, Biometals, 2001, 14, 343–351 CrossRef CAS PubMed.
- S. A. Testero, C. Granados, D. Fernández, P. Gallego, G. Covaleda, D. Reverter, J. Vendrell, F. X. Avilés, I. Pallarès and S. Mobashery, Discovery of mechanism-based inactivators for human pancreatic carboxypeptidase A from a focused synthetic library, ACS Med. Chem. Lett., 2017, 8, 1122–1127 CrossRef CAS PubMed.
- A. M. Hosie, E. L. Dunne, R. J. Harvey and T. G. Smart, Zinc-mediated inhibition of GABAA receptors: Discrete binding sites underlie subtype specificity, Nat. Neurosci., 2003, 6, 362–369 CrossRef CAS PubMed.
- T. Fukada, S. Yamasaki, K. Nishida, M. Murakami and T. Hirano, Zinc homeostasis and signaling in health and diseases: Zinc signaling, J. Biol. Inorg. Chem., 2011, 16, 1123–1134 CrossRef CAS PubMed.
- J. L. Beard, Iron biology in immune function, muscle metabolism and neuronal functioning, J. Nutr., 2001, 131, 568S–579S CrossRef CAS PubMed.
- Y. Ke and Z. M. Qian, Brain iron metabolism: Neurobiology and neurochemistry, Prog. Neurobiol., 2007, 83, 149–173 CrossRef CAS PubMed.
- R. P. L. van Swelm, J. F. M. Wetzels and D. W. Swinkels, The multifaceted role of iron in renal health and disease, Nat. Rev. Nephrol., 2020, 16, 77–98 CrossRef CAS PubMed.
- N. C. Anastasiadis, G. Bilis, J. C. Plakatouras, C. P. Raptopoulou, V. Psycharis, C. Beavers, S. J. Teat, M. Louloudi and S. P. Perlepes, Iron(III) chloride-benzotriazole adducts with trigonal bipyramidal geometry: Spectroscopic, structural and catalytic studies, Polyhedron, 2013, 64, 189–202 CrossRef CAS.
- E. J. Hawrelak, W. H. Bernskoetter, E. Lobkovsky, G. T. Yee, E. Bill and P. J. Chirik, Square planar vs. tetrahedral geometry in four coordinate iron(II) complexes, Inorg. Chem., 2005, 44, 3103–3111 CrossRef CAS PubMed.
- J. Jung, C. M. Legendre, S. Demeshko, R. Herbst-Irmer and D. Stalke, Trigonal planar Iron(II) and Cobalt(II) complexes containing [RS(NtBu)3]n− (R = NtBu, n = 2; CH2PPh2, n = 1) as acute bite-angle chelating ligands: Soft P donor proves beneficial to magnetic Co species, Inorg. Chem., 2021, 60, 9580–9588 CrossRef CAS PubMed.
- R. Shi, W. Hou, Z.-Q. Wang and X. Xu, Biogenesis of iron-sulfur clusters and their role in DNA metabolism, Front. Cell Dev. Biol., 2021, 9, 735678 CrossRef PubMed.
- K. P. Kepp, Heme: From quantum spin crossover to oxygen manager of life, Coord. Chem. Rev., 2017, 344, 363–374 CrossRef CAS.
- B. San Francisco, E. C. Bretsnyder and R. G. Kranz, Human mitochondrial holocytochrome c synthase's heme binding, maturation determinants, and complex formation with cytochrome c, Proc. Natl. Acad. Sci. U. S. A., 2013, 110, E788–797 CrossRef CAS PubMed.
- R. G. Kranz, C. Richard-Fogal, J.-S. Taylor and E. R. Frawley, Cytochrome c biogenesis: Mechanisms for covalent modifications and trafficking of heme and for heme-iron redox control, Microbiol. Mol. Biol. Rev., 2009, 73, 510–528 CrossRef CAS PubMed.
- C. Sanders, S. Turkarslan, D.-W. Lee and F. Daldal, Cytochrome c biogenesis: The Ccm system, Trends Microbiol., 2010, 18, 266–274 CrossRef CAS PubMed.
- M. Fontecave, Iron–sulfur clusters: Ever-expanding roles, Nat. Chem. Biol., 2006, 2, 171–174 CrossRef CAS PubMed.
- M.-E. Pandelia, D. Bykov, R. Izsak, P. Infossi, M.-T. Giudici-Orticoni, E. Bill, F. Neese and W. Lubitz, Electronic structure of the unique [4Fe–3S] cluster in O2-tolerant hydrogenases characterized by 57Fe Mossbauer and EPR spectroscopy, Proc. Natl. Acad. Sci. U. S. A., 2013, 110, 483–488 CrossRef CAS PubMed.
- K. Waløen, R. Kleppe, A. Martinez and J. Haavik, Tyrosine and tryptophan hydroxylases as therapeutic targets in human disease, Expert Opin. Ther. Targets, 2017, 21, 167–180 CrossRef PubMed.
- P. Matak, A. Matak, S. Moustafa, D. K. Aryal, E. J. Benner, W. Wetsel and N. C. Andrews, Disrupted iron homeostasis causes dopaminergic neurodegeneration in mice, Proc. Natl. Acad. Sci. U. S. A., 2016, 113, 3428–3435 CrossRef CAS PubMed.
- N. Singh, S. Haldar, A. K. Tripathi, K. Horback, J. Wong, D. Sharma, A. Beserra, S. Suda, C. Anbalagan, S. Dev, C. K. Mukhopadhyay and A. Singh, Brain iron homeostasis: From molecular mechanisms to clinical significance and therapeutic opportunities, Antioxid. Redox Signaling, 2014, 20, 1324–1363 CrossRef CAS PubMed.
- S. Masaldan, A. I. Bush, D. Devos, A. S. Rolland and C. Moreau, Striking while the iron is hot: Iron metabolism and ferroptosis in neurodegeneration, Free Radical Biol. Med., 2019, 133, 221–233 CrossRef CAS PubMed.
- A. Ndayisaba, C. Kaindlstorfer and G. K. Wenning, Iron in neurodegeneration – cause or consequence?, Front. Neurosci., 2019, 13, 180 CrossRef PubMed.
- M. Jakaria, A. A. Belaidi, A. I. Bush and S. Ayton, Ferroptosis as a mechanism of neurodegeneration in Alzheimer's disease, J. Neurochem., 2021, 159, 804–825 CrossRef CAS PubMed.
- L. Mahoney-Sánchez, H. Bouchaoui, S. Ayton, D. Devos, J. A. Duce and J.-C. Devedjian, Ferroptosis and its potential role in the physiopathology of Parkinson's Disease, Prog. Neurobiol., 2021, 196, 101890 CrossRef PubMed.
- Y. An, S. Li, X. Huang, X. Chen, H. Shan and M. Zhang, The role of copper homeostasis in brain disease, Int. J. Mol. Sci., 2022, 23, 13850 CrossRef CAS PubMed.
- E. Bafaro, Y. Liu, Y. Xu and R. E. Dempski, The emerging role of zinc transporters in cellular homeostasis and cancer, Signal Transduction Targeted Ther., 2017, 2, 17029 CrossRef PubMed.
- M. Bisaglia and L. Bubacco, Copper ions and Parkinson's disease: Why is homeostasis so relevant?, Biomolecules, 2020, 10, 195 CrossRef CAS PubMed.
- F. Ren, B. L. Logeman, X. Zhang, Y. Liu, D. J. Thiele and P. Yuan, X-ray structures of the high-affinity copper transporter Ctr1, Nat. Commun., 2019, 10, 1386 CrossRef PubMed.
- C. J. De Feo, S. G. Aller, G. S. Siluvai, N. J. Blackburn and V. M. Unger, Three-dimensional structure of the human copper transporter hCTR1, Proc. Natl. Acad. Sci. U. S. A., 2009, 106, 4237–4242 CrossRef CAS PubMed.
- M. T. Morgan, D. Bourassa, S. Harankhedkar, A. M. McCallum, S. A. Zlatic, J. S. Calvo, G. Meloni, V. Faundez and C. J. Fahrni, Ratiometric two-photon microscopy reveals attomolar copper buffering in normal and Menkes mutant cells, Proc. Natl. Acad. Sci. U. S. A., 2019, 116, 12167–12172 CrossRef CAS PubMed.
- I. F. Scheiber, J. F. Mercer and R. Dringen, Metabolism and functions of copper in brain, Prog. Neurobiol., 2014, 116, 33–57 CrossRef CAS PubMed.
- M. Lu, J. Chai and D. Fu, Structural basis for autoregulation of the zinc transporter YiiP, Nat. Struct. Mol. Biol., 2009, 16, 1063–1067 CrossRef CAS PubMed.
- J. I. Mujika, X. Lopez, E. Rezabal, R. Castillo, S. Marti, V. Moliner and J. M. Ugalde, A QM/MM study of the complexes formed by aluminum and iron with serum transferrin at neutral and acidic pH, J. Inorg. Biochem., 2011, 105, 1446–1456 CrossRef CAS PubMed.
- Z. M. Qian, H. Y. Li, H. Z. Sun and K. Ho, Targeted drug delivery via the transferrin receptor-mediated endocytosis pathway, Pharmacol. Rev., 2002, 54, 561–587 CrossRef CAS PubMed.
- C. Schulz, G. Eisenhofer and H. Lehnert, Principles of catecholamine biosynthesis, metabolism and release, Front. Horm. Res., 2004, 31, 1–25 CAS.
- R. Dalangin, A. Kim and R. E. Campbell, The role of amino acids in neurotransmission and fluorescent tools for their detection, Int. J. Mol. Sci., 2020, 21, 6197 CrossRef CAS PubMed.
- D. Vallone, R. Picetti and E. Borrelli, Structure and function of dopamine receptors, Neurosci. Biobehav. Rev., 2000, 24, 125–132 CrossRef CAS PubMed.
- B. W. Dunlop and C. B. Nemeroff, The role of dopamine in the pathophysiology of depression, Arch. Gen. Psychiatry, 2007, 64, 327–337 CrossRef CAS PubMed.
- D. Meder, D. M. Herz, J. B. Rowe, S. Lehéricy and H. R. Siebner, The role of dopamine in the brain – lessons learned from Parkinson's disease, NeuroImage, 2019, 190, 79–93 CrossRef CAS PubMed.
- L. Chen and J. Xie, Dopamine in Parkinson's disease: Precise supplementation with motor planning, Neurosci. Bull., 2018, 34, 873–874 CrossRef PubMed.
- M.-M. Liu, S.-M. Han, X.-W. Zheng, L.-L. Han, T. Liu and Z.-Y. Yu, Experimental and theoretical prediction of the redox potential of dopamine and its supramolecular complex with glycine, Int. J. Electrochem. Sc., 2015, 10, 235–247 CrossRef.
- A. N. Pham and T. D. Waite, Cu(II)-catalyzed oxidation of dopamine in aqueous solutions: Mechanism and kinetics, J. Inorg. Biochem., 2014, 137, 74–84 CrossRef CAS PubMed.
- J. S. Thompson and J. C. Calabrese, Copper catechol chemistry – synthesis, spectroscopy, and structure of Bis(3,5-di-tert-butyl-o-semiquinato)copper(II), J. Am. Chem. Soc., 1986, 108, 1903–1907 CrossRef CAS.
- Y. Sun, A. N. Pham and T. D. Waite, Elucidation of the interplay between Fe(II), Fe(III), and dopamine with relevance to iron solubilization and reactive oxygen species generation by catecholamines, J. Neurochem., 2016, 137, 955–968 CrossRef CAS PubMed.
- G. P. Maier, C. M. Bernt and A. Butler, Catechol oxidation: considerations in the design of wet adhesive materials, Biomater. Sci., 2018, 6, 332–339 RSC.
- E. Monzani, S. Nicolis, S. Dell’Acqua, A. Capucciati, C. Bacchella, F. A. Zucca, E. V. Mosharov, D. Sulzer, L. Zecca and L. Casella, Dopamine, oxidative stress and protein-quinone modifications in Parkinson's and other neurodegenerative diseases, Angew. Chem., Int. Ed., 2019, 58, 6512–6527 CrossRef CAS PubMed.
- M. Asanuma, I. Miyazaki and N. Ogawa, Dopamine- or L-DOPA-induced neurotoxicity: The role of dopamine quinone formation and tyrosinase in a model of Parkinson's disease, Neurotoxic. Res., 2003, 5, 165–176 CrossRef PubMed.
- J. Segura-Aguilar, On the role of aminochrome in mitochondrial dysfunction and endoplasmic reticulum stress in Parkinson's disease, Front. Neurosci., 2019, 13, 271 CrossRef PubMed.
- J. Segura-Aguilar, I. Paris, P. Muñoz, E. Ferrari, L. Zecca and F. A. Zucca, Protective and toxic roles of dopamine in Parkinson's disease, J. Neurochem., 2014, 129, 898–915 CrossRef CAS PubMed.
- A. M. Willard, R. S. Bouchard and A. H. Gittis, Differential degradation of motor deficits during gradual dopamine depletion with 6-hydroxydopamine in mice, Neuroscience, 2015, 301, 254–267 CrossRef CAS PubMed.
- R. Cataldi, S. Chia, K. Pisani, F. S. Ruggeri, C. K. Xu, T. Šneideris, M. Perni, S. Sarwat, P. Joshi, J. R. Kumita, S. Linse, J. Habchi, T. P. J. Knowles, B. Mannini, C. M. Dobson and M. Vendruscolo, A dopamine metabolite stabilizes neurotoxic amyloid-β oligomers, Commun. Biol., 2021, 4, 19 CrossRef CAS PubMed.
- M. Ramesh, P. Makam, C. Voshavar, H. Khare, K. Rajasekhar, S. Ramakumar and T. Govindaraju,
L-Dopa and dopamine conjugated naphthalenediimides modulate amyloid β toxicity, Org. Biomol. Chem., 2018, 16, 7682–7692 RSC.
- E. Nam, J. S. Derrick, S. Lee, J. Kang, J. Han, S. J. C. Lee, S. W. Chung and M. H. Lim, Regulatory activities of dopamine and its derivatives toward metal-free and metal-induced Amyloid-β aggregation, oxidative stress, and inflammation in Alzheimer's disease, ACS Chem. Neurosci., 2018, 9, 2655–2666 CrossRef CAS PubMed.
- C. Bacchella, S. Dell’Acqua, S. Nicolis, E. Monzani and L. Casella, The reactivity of copper complexes with neuronal peptides promoted by catecholamines and its impact on neurodegeneration, Coord. Chem. Rev., 2022, 471, 214756 CrossRef CAS.
- C. Bacchella, S. Nicolis, S. Dell’Acqua, E. Rizzarelli, E. Monzani and L. Casella, Membrane binding strongly affecting the dopamine reactivity induced by copper prion and copper/amyloid-β (Aβ) peptides. A ternary copper/Aβ/prion peptide complex stabilized and solubilized in sodium dodecyl sulfate micelles, Inorg. Chem., 2020, 59, 900–912 CrossRef CAS PubMed.
- E. Höglund, Ø. Øverli and S. Winberg, Tryptophan metabolic pathways and brain serotonergic activity: A comparative review, Front. Endocrinol., 2019, 10, 158 CrossRef PubMed.
- C. N. Sutanto, W. W. Loh and J. E. Kim, The impact of tryptophan supplementation on sleep quality: a systematic review, meta-analysis, and meta-regression, Nutr. Rev., 2022, 80, 306–316 CrossRef PubMed.
-
O. Kokturk and A. Kanbay, Tryptophan metabolism and sleep, in tryptophan metabolism: Implications for biological processes, health and disease. ed. A. Engin and A. Engin, Humana Press, Cham, 2015 Search PubMed.
- R. C. Dunbar, J. D. Steill, N. C. Polfer and J. Oomens, Dimeric complexes of tryptophan with M2+ metal ions, J. Phys. Chem. A, 2009, 113, 845–851 CrossRef CAS PubMed.
- M. Remko, D. Fitz, R. Broer and B. M. Rode, Effect of metal Ions (Ni2+, Cu2+ and Zn2+) and water coordination on the structure of L-phenylalanine, L-tyrosine, L-tryptophan and their zwitterionic forms, J. Mol. Model, 2011, 17, 3117–3128 CrossRef CAS PubMed.
- A. Mor, A. Tankiewicz-Kwedlo, A. Krupa and D. Pawlak, Role of kynurenine pathway in oxidative stress during neurodegenerative disorders, Cells, 2021, 10, 1603 CrossRef CAS PubMed.
- D. C. Maddison and F. Giorgini, The kynurenine pathway and neurodegenerative disease, Semin. Cell Dev. Biol., 2015, 40, 134–141 CrossRef CAS PubMed.
- L. B. Martins, A. L. M. Silveira and A. L. Teixeira, The involvement of kynurenine pathway in neurodegenerative diseases, Curr. Neuropharmacol., 2023, 21, 260–272 CrossRef CAS PubMed.
- M. Fathi, K. Vakili, S. Yaghoobpoor, A. Tavasol, K. Jazi, R. Hajibeygi, S. Shool, F. Sodeifian, A. Klegeris, A. McElhinney, M. R. Tavirani and F. Sayehmiri, Dynamic changes in metabolites of the kynurenine pathway in Alzheimer's disease, Parkinson's disease, and Huntington's disease: A systematic review and meta-analysis, Front. Immunol., 2022, 13, 997240 CrossRef CAS PubMed.
- T. Behl, I. Kaur, A. Sehgal, S. Singh, S. Bhatia, A. Al-Harrasi, G. Zengin, A. G. Bumbu, F. L. Andronie-Cioara, A. C. Nechifor, D. Gitea, A. F. Bungau, M. M. Toma and S. G. Bungau, The footprint of kynurenine pathway in neurodegeneration: Janus-faced role in Parkinson's disorder and therapeutic implications, Int. J. Mol. Sci., 2021, 22, 6737 CrossRef CAS PubMed.
- M. Platten, E. A. A. Nollen, U. F. Röhrig, F. Fallarino and C. A. Opitz, Tryptophan metabolism as a common therapeutic target in cancer, neurodegeneration and beyond, Nat. Rev. Drug Discovery, 2019, 18, 379–401 CrossRef CAS PubMed.
- K. Hestad, J. Alexander, H. Rootwelt and J. O. Aaseth, The role of tryptophan dysmetabolism and quinolinic acid in depressive and neurodegenerative diseases, Biomolecules, 2022, 12, 998 CrossRef CAS PubMed.
- G. V. Barnett, G. Balakrishnan, N. Chennamsetty, L. Hoffman, J. Bongers, L. Tao, Y. Huang, T. Slaney, T. K. Das, A. Leone and S. R. Kar, Probing the tryptophan environment in therapeutic proteins: Implications for higher order structure on tryptophan oxidation, J. Pharm. Sci., 2019, 108, 1944–1952 CrossRef CAS PubMed.
- J. Kindler, C. K. Lim, C. S. Weickert, D. Boerrigter, C. Galletly, D. Liu, K. R. Jacobs, R. Balzan, J. Bruggemann, M. O’Donnell, R. Lenroot, G. J. Guillemin and T. W. Weickert, Dysregulation of kynurenine metabolism is related to proinflammatory cytokines, attention, and prefrontal cortex volume in schizophrenia, Mol. Psychiatry, 2020, 25, 2860–2872 CrossRef PubMed.
- P. A. Shah, C. J. Park, M. P. Shaughnessy and R. A. Cowles, Serotonin as a mitogen in the gastrointestinal tract: Revisiting a familiar molecule in a new role, Cell Mol. Gastroenterol. Hepatol., 2021, 12, 1093–1104 CrossRef CAS PubMed.
- T. Guzel and D. Mirowska-Guzel, The role of serotonin neurotransmission in gastrointestinal tract and pharmacotherapy, Molecules, 2022, 27, 1680 CrossRef CAS PubMed.
- M. Politis and F. Niccolini, Serotonin in Parkinson's disease, Behav. Brain Res., 2015, 277, 136–145 CrossRef CAS PubMed.
- X. Wang, M. Hu, Q. Xie, C. Geng, C. Jin, W. Ren, J. Fan, T. Ma and B. Hu, Amyloid β oligomers disrupt piriform cortical output via a serotonergic pathway, Neurobiol. Aging, 2023, 121, 64–77 CrossRef CAS PubMed.
- A. P. Reddy, N. Sawant, H. Morton, S. Kshirsagar, L. E. Bunquin, X. Yin and P. H. Reddy, Selective serotonin reuptake inhibitor citalopram ameliorates cognitive decline and protects against amyloid beta-induced mitochondrial dynamics, biogenesis, autophagy, mitophagy and synaptic toxicities in a mouse model of Alzheimer's disease, Hum. Mol. Genet., 2021, 30, 789–810 CrossRef CAS PubMed.
- C. E. Jones, C. K. Underwood, E. J. Coulson and P. J. Taylor, Copper induced oxidation of serotonin: Analysis of products and toxicity, J. Neurochem., 2007, 102, 1035–1043 CrossRef CAS PubMed.
- S. Mukherjee, M. Seal and S. G. Dey, Kinetics of serotonin oxidation by heme–Aβ relevant to Alzheimer's disease, J. Biol. Inorg. Chem., 2014, 19, 1355–1365 CrossRef CAS PubMed.
- A. K. Nath, A. Ghatak, A. Dey and S. G. Dey, Intermediates involved in serotonin oxidation catalyzed by Cu bound Aβ peptides, Chem. Sci., 2020, 12, 1924–1929 RSC.
- Y. Kato, S. Ono, N. Kitamoto and A. J. Kettle, Covalent modification of cytoskeletal proteins in neuronal cells by tryptamine-4,5-dione, Redox. Biol., 2014, 2, 983–990 CrossRef CAS PubMed.
- Y. Kato, A. V. Peskin, N. Dickerhof, D. T. Harwood and A. J. Kettle, Myeloperoxidase catalyzes the conjugation of serotonin to thiols via free radicals and tryptamine-4,5-dione, Chem. Res. Toxicol., 2012, 25, 2322–2332 Search PubMed.
- M. Z. Wrona and G. Dryhurst, A putative metabolite of serotonin, tryptamine-4,5-dione, is an irreversible inhibitor of tryptophan hydroxylase: Possible relevance to the serotonergic neurotoxicity of methamphetamine, Chem. Res. Toxicol., 2001, 14, 1184–1192 Search PubMed.
- H. Liang, N. Liu, R. Wang, Y. Zhang, J. Chen, Z. Dai, Y. Yang, G. Wu and Z. Wu,
N-acetyl serotonin alleviates oxidative damage by activating nuclear factor erythroid 2-related factor 2 signaling in porcine enterocytes, Antioxidants, 2020, 9, 303 Search PubMed.
- N. Suga, A. Murakami, Y. Nakamura, A. Ishisaka, N. Kitamoto, M. Ito and Y. Kato, Cytotoxic and cytoprotective effects of tryptamine-4,5-dione on neuronal cells: A double-edged sword, Free Radical Res., 2017, 51, 545–553 CrossRef CAS PubMed.
- J. Motiejunaite, L. Amar and E. Vidal-Petiot, Adrenergic receptors and cardiovascular effects of catecholamines, Ann. Endocrinol., 2021, 82, 193–197 CrossRef PubMed.
- J. A. Sierra-Fonseca and K. L. Gosselink, Tauopathy and neurodegeneration: A role for stress, Neurobiol. Stress, 2018, 9, 105–112 CrossRef PubMed.
- V. M. Costa, R. Silva, L. M. Ferreira, P. S. Branco, F. Carvalho, M. L. Bastos, R. A. Carvalho, M. Carvalho and F. Remião, Oxidation process of adrenaline in freshly isolated rat cardiomyocytes: Formation of adrenochrome, quinoproteins, and GSH adduct, Chem. Res. Toxicol., 2007, 20, 1183–1191 Search PubMed.
- K. Jodko and G. Litwinienko, Oxidative stress in the neurodegenerative diseases-potential antioxidant activity of catecholamines, Postepy Biochem., 2010, 56, 248–259 Search PubMed.
- A. Roberts, D. Bar-Or, J. V. Winkler and L. T. Rael, Copper-induced oxidation of epinephrine: Protective effect of D-DAHK, a synthetic analogue of the high affinity copper binding site of human albumin, Biochem. Biophys. Res. Commun., 2003, 304, 755–757 CrossRef CAS PubMed.
- R. Sainz, M. Del Pozo, M. Vilas-Varela, J. Castro-Esteban, M. P. Corral, L. Vázquez, E. Blanco, D. Peña, J. A. Martín-Gago, G. J. Ellis, M. D. Petit-Domínguez, C. Quintana and E. Casero, Chemically synthesized chevron-like graphene nanoribbons for electrochemical sensors development: Determination of epinephrine, Sci. Rep., 2020, 10, 14614 CrossRef CAS PubMed.
- J. P. Spencer, M. Whiteman, P. Jenner and B. Halliwell, 5-s-Cysteinyl-conjugates of catecholamines induce cell damage, extensive DNA base modification and increases in caspase-3 activity in neurons, J. Neurochem., 2002, 81, 122–129 CrossRef CAS PubMed.
-
M. Akram, M. Daniyal, A. Ali, R. Zainab, S. Muhammad, A. Shah, N. Munir and I. M. Tahir, Role of phenylalanine and its metabolites in health and neurological disorders, in Synucleins—biochemistry and role in diseases, ed. A. Surguchov, IntechOpen, 2020 Search PubMed.
- J. D. Fernstrom and M. H. Fernstrom, Tyrosine, phenylalanine, and catecholamine synthesis and function in the
brain, J. Nutr., 2007, 137, 1539S–1547S CrossRef CAS PubMed.
- Z. Xie and G. M. Miller, β-phenylethylamine alters monoamine transporter function via trace amine-associated receptor 1: Implication for modulatory roles of trace amines in brain, J. Pharmacol. Exp. Ther., 2008, 325, 617–628 CrossRef CAS PubMed.
- G. Zsilla, D. E. Hegyi, M. Baranyi and E. S. Vizi, 3,4-Methylenedioxymethamphetamine, mephedrone, and β-phenylethylamine release dopamine from the cytoplasm by means of transporters and keep the concentration high and constant by blocking reuptake, Eur. J. Pharmacol., 2018, 837, 72–80 CrossRef CAS PubMed.
- D. Bagchi, A. Maity, S. K. De and A. Chakraborty, Metal-ion-induced evolution of phenylalanine self-assembly: Structural polymorphism of novel metastable intermediates, J. Phys. Chem. Lett., 2022, 13, 10409–10417 CrossRef CAS PubMed.
- T. Kawano, Prion-derived copper-binding peptide fragments catalyze the generation of superoxide anion in the presence of aromatic monoamines, Int. J. Biol. Sci., 2007, 3, 57–63 CrossRef CAS PubMed.
- M. K. Mazumder, R. Paul and A. Borah, β-phenethylamine-a phenylalanine derivative in brain-contributes to oxidative stress by inhibiting mitochondrial complexes and DT-diaphorase: An in silico study, CNS Neurosci. Ther., 2013, 19, 596–602 CrossRef CAS PubMed.
- T. Sengupta and K. P. Mohanakumar, 2-Phenylethylamine, a constituent of chocolate and wine, causes mitochondrial complex-I inhibition, generation of hydroxyl radicals and depletion of striatal biogenic amines leading to psycho-motor dysfunctions in Balb/c mice, Neurochem. Int., 2010, 57, 637–646 CrossRef CAS PubMed.
- S. A. Hudson, H. Ecroyd, T. W. Kee and J. A. Carver, The thioflavin T fluorescence assay for amyloid fibril detection can be biased by the presence of exogenous compounds, FEBS J., 2009, 276, 5960–5972 CrossRef CAS PubMed.
- A. F. Russo, Overview of neuropeptides: Awakening the senses?, Headache, 2017, 57, 37–46 CrossRef PubMed.
- X. Y. Yeo, G. Cunliffe, R. C. Ho, S. S. Lee and S. Jung, Potentials of neuropeptides as therapeutic agents for neurological diseases, Biomedicines, 2022, 10, 343 CrossRef CAS PubMed.
- J. Han, J. Yoon, J. Shin, E. Nam, T. Qian, Y. Li, K. Park, S. H. Lee and M. H. Lim, Conformational and functional changes of the native neuropeptide somatostatin occur in the presence of copper and amyloid-β, Nat. Chem., 2022, 14, 1021–1030 CrossRef CAS PubMed.
- E. Chruścinska, I. Derdowska, H. Kozłowski, B. Lammek, M. Łuczkowski, S. Ołdziejb and J. Świątek-Kozłowska, Unusual gain in the coordination ability of vasopressin-like peptides towards Cu2+ ions by insertion of the highly hydrophobic side chain, New J. Chem., 2003, 27, 251–256 RSC.
- W. Bal, H. Kozlowski, B. Lammek, L. D. Pettit and K. Rolka, Potentiometric and spectroscopic studies of the Cu(II) complexes of Ala-Arg8-vasopressin and oxytocin: Two vasopressin-like peptides, J. Inorg. Biochem., 1992, 45, 193–202 CrossRef CAS PubMed.
- T. Kleszczewski, B. Modzelewska, W. Bal, M. Sipowicz and A. Kostrzewska, Cu(II) complexation potentiates arginine vasopressin action on nonpregnant human myometrium in vitro, Contraception, 2003, 67, 477–483 CrossRef CAS PubMed.
- T. Wyttenbach, D. Liu and M. T. Bowers, Interactions of the hormone oxytocin with divalent metal ions, J. Am. Chem. Soc., 2008, 130, 5993–6000 CrossRef CAS PubMed.
- I. Alshanski, D. E. Shalev, S. Yitzchaik and M. Hurevich, Determining the structure and binding mechanism of oxytocin-Cu2+ complex using paramagnetic relaxation enhancement NMR analysis, J. Biol. Inorg. Chem., 2021, 26, 809–815 CrossRef CAS PubMed.
- H. Inoue and M. Hirobe, Interchange reaction of disulfides and denaturation of oxytocin by copper(II)/ascorbic acid/O2 system, Biochem. Biophys. Res. Commun., 1987, 145, 596–603 CrossRef CAS PubMed.
- O. Rogoza, K. Megnis, M. Kudrjavceva, A. Gerina-Berzina and V. Rovite, Role of somatostatin signalling in neuroendocrine tumours, Int. J. Mol. Sci., 2022, 23, 1447 CrossRef CAS PubMed.
- S. Reichlin, Somatostatin, N. Engl. J. Med., 1983, 309, 1495–1501 CrossRef CAS PubMed.
- P. Barnett, Somatostatin and somatostatin receptor physiology, Endocrine, 2003, 20, 255–264 CrossRef CAS PubMed.
- G. Lepousez, A. Mouret, C. Loudes, J. Epelbaum and C. Viollet, Somatostatin contributes to in vivo gamma oscillation modulation and odor discrimination in the olfactory bulb, J. Neurosci., 2010, 30, 870–875 CrossRef CAS PubMed.
- S. Nocera, A. Simon, O. Fiquet, Y. Chen, J. Gascuel, F. Datiche, N. Schneider, J. Epelbaum and C. Viollet, Somatostatin serves a modulatory role in the mouse olfactory bulb: Neuroanatomical and behavioral evidence, Front. Behav. Neurosci., 2019, 13, 61 CrossRef CAS PubMed.
- D. Saiz-Sanchez, I. Ubeda-Bañon, A. Flores-Cuadrado, M. Gonzalez-Rodriguez, S. Villar-Conde, V. Astillero-Lopez and A. Martinez-Marcos, Somatostatin, olfaction, and neurodegeneration, Front. Neurosci., 2020, 14, 96 CrossRef PubMed.
- Y.-H. Song, J. Yoon and S.-H. Lee, The role of neuropeptide somatostatin in the brain and its application in treating neurological disorders, Exp. Mol. Med., 2021, 53, 328–338 CrossRef CAS PubMed.
- A. Flores-Cuadrado, I. Ubeda-Bañon, D. Saiz-Sanchez and A. Martinez-Marcos, α-Synucleinopathy in the human amygdala in Parkinson disease: Differential vulnerability of somatostatin- and parvalbumin-expressing neurons, J. Neuropathol. Exp. Neurol., 2017, 76, 754–758 CrossRef CAS PubMed.
- H. Wang, L. D. Muiznieks, P. Ghosh, D. Williams, M. Solarski, A. Fang, A. Ruiz-Riquelme, R. Pomès, J. C. Watts, A. Chakrabartty, H. Wille, S. Sharpe and G. Schmitt-Ulms, Somatostatin binds to the human amyloid β peptide and favors the formation of distinct oligomers, eLife, 2017, 6, e28401 CrossRef PubMed.
- M. Solarski, H. Wang, H. Wille and G. Schmitt-Ulms, Somatostatin in Alzheimer's disease: A new role for an old player, Prion, 2018, 12, 1–8 CrossRef CAS PubMed.
- R. Wu, L. R. Benzenberg, D. Svingou and R. Zenobi, The structure of cyclic neuropeptide somatostatin and octapeptide octreotide in the presence of copper ions: Insights from transition metal ion FRET and native ion mobility-mass spectrometry, J. Am. Chem. Soc., 2023, 145, 10542–10547 CrossRef CAS PubMed.
- T. A. Treschan and J. Peters, The vasopressin system: Physiology and clinical strategies, Anesthesiology, 2006, 105, 599–612 CrossRef CAS PubMed.
- L. Bankir, S. Fernandes, P. Bardoux, N. Bouby and D. G. Bichet, Vasopressin-V2 receptor stimulation reduces sodium excretion in healthy humans, J. Am. Soc. Nephrol., 2005, 16, 1920–1928 CrossRef CAS PubMed.
- L. Bankir, Antidiuretic action of vasopressin: Quantitative aspects and interaction between V1a and V2 receptor-mediated effects, Cardiovasc. Res., 2001, 51, 372–390 CrossRef CAS PubMed.
- M. Birnbaumer, The V2 vasopressin receptor mutations and fluid homeostasis, Cardiovasc. Res., 2001, 51, 409–415 CrossRef CAS PubMed.
- A. W. Cowley, Jr. and J. F. Liard, Vasopressin and arterial pressure regulation. Special lecture, Hypertension, 1988, 11, I25–32 CrossRef PubMed.
- H. Knotzer, W. Pajk, S. Maier, R. Ladurner, A. Kleinsasser, V. Wenzel, M. W. Dünser, H. Ulmer and W. R. Hasibeder, Arginine vasopressin reduces intestinal oxygen supply and mucosal tissue oxygen tension, Am. J. Physiol. Heart Circ. Physiol., 2005, 289, H168–173 CrossRef CAS PubMed.
- L. V. Scott and T. G. Dinan, Vasopressin and the regulation of hypothalamic-pituitary-adrenal axis function: Implications for the pathophysiology of depression, Life Sci., 1998, 62, 1985–1998 CrossRef CAS PubMed.
- T. G. Dinan, S. O’Brien, E. Lavelle and L. V. Scott, Further neuroendocrine evidence of enhanced vasopressin V3 receptor responses in melancholic depression, Psychol. Med., 2004, 34, 169–172 CrossRef CAS PubMed.
- S. Kotliarova, N. R. Jana, N. Sakamoto, M. Kurosawa, H. Miyazaki, M. Nekooki, H. Doi, Y. Machida, H. K. Wong, T. Suzuki, C. Uchikawa, Y. Kotliarov, K. Uchida, Y. Nagao, U. Nagaoka, A. Tamaoka, K. Oyanagi, F. Oyama and N. Nukina, Decreased expression of hypothalamic neuropeptides in Huntington disease transgenic mice with expanded polyglutamine-EGFP fluorescent aggregates, J. Neurochem., 2005, 93, 641–653 CrossRef CAS PubMed.
- X. Zhang, F. Zhao, C. Wang, J. Zhang, Y. Bai, F. Zhou, Z. Wang, M. Wu, W. Yang, J. Guo and J. Qi, AVP(4-8) improves cognitive behaviors and hippocampal synaptic plasticity in the APP/PS1 mouse model of Alzheimer's disease, Neurosci. Bull., 2020, 36, 254–262 CrossRef CAS PubMed.
- G. Valensin, A. Maccotta, E. Gaggelli, Z. Grzonka, F. Kasprzykowski and H. Kozlowski,
1H-NMR and 13C-NMR investigation of complexes of Mn2+ with ocytocin analogues in (2H6)dimethylsulfoxide, Eur. J. Biochem., 1996, 240, 118 CrossRef CAS PubMed.
- H.-J. Lee, A. H. Macbeth, J. H. Pagani and W. S. Young, 3rd, Oxytocin: The great facilitator of life, Prog. Neurobiol., 2009, 88, 127–151 CAS.
- H. H. Zingg and S. A. Laporte, The oxytocin receptor, Trends Endocrinol. Metab., 2003, 14, 222–227 CrossRef CAS PubMed.
- L. Yuan, S. Liu, X. Bai, Y. Gao, G. Liu, X. Wang, D. Liu, T. Li, A. Hao and Z. Wang, Oxytocin inhibits lipopolysaccharide-induced inflammation in microglial cells and attenuates microglial activation in lipopolysaccharide-treated mice, J. Neuroinflammation, 2016, 13, 77 CrossRef PubMed.
- H. Amini-Khoei, A. Mohammadi-Asl, S. Amiri, M.-J. Hosseini, M. Momeny, M. Hassanipour, M. Rastegar, A. H. Mirzaian, A. H. Mirzaian, H. Sanjarimoghaddam, S. E. Mehr and A. R. Dehpour, Oxytocin mitigated the depressive-like behaviors of maternal separation stress through modulating mitochondrial function and neuroinflammation, Prog. Neuropsychopharmacol. Biol. Psychiatry, 2017, 76, 169–178 CrossRef CAS PubMed.
- K. Karelina, K. A. Stuller, B. Jarrett, N. Zhang, J. Wells, G. J. Norman and A. C. DeVries, Oxytocin mediates social neuroprotection after cerebral ischemia, Stroke, 2011, 42, 3606–3611 CrossRef CAS PubMed.
- S. E. Moghadam, A. A. Tameh, Z. Vahidinia, M. A. Atlasi, H. H. Bafrani and H. Naderian, Neuroprotective effects of oxytocin hormone after an experimental stroke model and the possible role of Calpain-1, J. Stroke Cerebrovasc. Dis., 2018, 27, 724–732 CrossRef PubMed.
|
This journal is © The Royal Society of Chemistry 2023 |
Click here to see how this site uses Cookies. View our privacy policy here.