DOI:
10.1039/D2BM01625G
(Paper)
Biomater. Sci., 2023,
11, 235-247
A multifunctional black phosphorus-based adhesive patch intrinsically induces partial EMT for effective burn wound healing†
Received
6th October 2022
, Accepted 10th November 2022
First published on 18th November 2022
Abstract
The ultimate goal of cutaneous wound healing is to reform a stratified epithelium to restore the normal epidermal barrier, which involves the epithelial-to-mesenchymal transition (EMT) process. However, healing strategies based on EMT induction are immature and ambiguous to date. Excessive induction of EMT may cause fibrosis, hypertrophic scarring, and increased risk of malignancy. Here, we present a new EMT-inducing strategy for eliciting partial EMT to facilitate proper epithelial cell migration. The new EMT-inducing system integrates black phosphorus nanosheets (BPNSs), catechol-modified chitosan (CA-CS), and oxidized dextran (Odex) to engineer an adhesive hydrogel patch (C&BP-Patch) with remarkable efficacy on infectious burn wound healing. The C&BP-Patch can orchestrate key early skin wound healing processes including hemostasis, inflammation, and proliferation, which enable fast partial EMT induction to restore an intact epithelial barrier. The C&BP-Patch acts initially as a high-performance bio-sealant to create a moist and stable microenvironment for EMT. Moreover, the photothermal effects of the C&BP-Patch can eliminate bacteria, accelerate microcirculation and reduce inflammation to maintain a proper EMT. Most importantly, the BPNSs can intrinsically induce partial EMT of epithelial cells via a Snail1-mediated signaling pathway. Therefore, our study proposes a new strategy for effective infectious burn wound healing based on inducing partial EMT.
Introduction
Burn trauma is a type of injury that can be caused by heat, electricity, freezing, and chemicals, and dermal tissue is the first to bear the brunt. In addition to causing skin defects, there are many fatal physical complications of burn victims depending on the burn location and depth, including infection, electrolyte imbalances, and shock. Furthermore, long-term hospitalization, scarring, and deformity will lead to severe psychological and emotional distress.1,2 Various woven and nonwoven gauze dressings have been developed and are used in the clinic.3 However, major complications arise from frequent dressing changing and the resulting immense pain when stripping intertwined sections of dressing from wounds, which further worsen the wound condition, including raising inflammation and hindering the re-epithelialization.4,5 Moreover, these wound dressings cannot provide a proper moist environment for wound healing.3
Skin wound healing is a highly orchestrated process that comprises four sequential, yet overlapping phases: (1) hemostasis, (2) inflammation, (3) proliferation, and (4) remodeling.6,7 Any perturbation in one of the four stages may prolong the phase and lead to an unsatisfactory outcome resulting in chronic wound healing. Upon injury, a fibrin clot rapidly forms to maintain homeostasis.8 Platelets within the blood trigger a coagulation cascade and secrete several growth factors (GFs), such as platelet-derived growth factor (PDGF), insulin-like growth factor-1 (IGF-1), and transforming growth factor-β (TGF-β), which initiate wound healing.9 In deep dermal burn wounds, the most frequently encountered complications are bacterial and fungal infections, which often led to death.10 Meanwhile, the effect of bacterial infection appears to disrupt epithelial cell junctions and epithelial migration.11,12
The ultimate goal of cutaneous wound healing is to reform a stratified epithelium to rebuild the normal epidermal barrier, which involves the epithelial-to-mesenchymal transition (EMT) process of epidermal basal layer cells surrounding the wound.13 During the process of EMT, epithelial basal cells undergo cytoskeleton rearrangement, losing intercellular junctions and polarity, yet acquiring a mesenchymal cell phenotype with enhanced motility and invasiveness.13,14 Most recently, EMT has been recognized as a “continuum” where metastable epidermal cells (ECs) can exhibit varying degrees of intermediate states, also known as partial EMT, between the epithelial “E” state and the mesenchymal “M” state.15 However, excessive EMT is not conducive to re-epithelialization and also leads to scarring in wound healing.16 In this regard, the ideal wound dressing should promote partial EMT of ECs to quickly restore the normal skin barrier.
The treatment of wound healing directly based on the EMT strategy is rarely reported and ambiguous. This is mainly due to the concern relating to fibrosis formation (or scarring) caused by excessive EMT.17 Clinical application of GFs includes platelet-derived growth factor (PDGF), vascular endothelial growth factor (VEGF), epidermal growth factor (EGF), and TGF-β, which often have multiple roles that can contribute to re-epithelialization.18 However, efficacy is limited since GFs rapidly diffuse from the wound bed and are readily deactivated by enzymes in the wound area.19 Moreover, high-level GFs may cause fibrosis and hypertrophic scarring, characterized by hyperproliferation of fibroblasts and overproduction of extracellular matrix (ECM).20 Therefore, GF-free strategies that induce partial EMT are particularly attractive.
Hydrogels are considered as ideal wound dressings.21 They are hydrophilic polymers with a 3D network structure that can absorb a large volume of water.22 Injectable adhesive hydrogels have the potential to adhere and bond damaged tissues together, thus creating a stable microenvironment and prompting the wound to heal properly. Such dressing sealants can also act as hemostatic agents, prevent the leakage of fluids and protect the wound from external bacteria.23,24 By integrating the advantages of hydrogels and the photothermal (PT) effect, multifunctional photo-responsive hydrogels are increasingly used in wound repair.25,26 However, no study strives to identify a bioactive hydrogel with intrinsic partial EMT-inducing activity.
Herein, we have developed a near-infrared (NIR) responsive wound dressing (C&BP-Patch) that integrates catechol-modified chitosan (CA-CS), oxidized dextran (Odex), and black phosphorus nanosheets (BPNSs). CA-CS shows a strong adhesive capability with wet heterogeneous wound surfaces and can absorb wound exudates.27,28 Oxidized dextran has multiple functional aldehyde groups, and can serve as a macromolecular crosslinker for those natural and synthetic polymers bearing free amino groups to form hydrogels.29 Therefore, CA-CS and Odex form a rapidly in situ gellable hydrogel by reacting amino residues on catechol chitosan with aldehyde groups on Odex via the Schiff base. Moreover, both the catechol modification and the crosslinker Odex promote the adhesion of CA-CS with wound tissue. As an emerging 2D layered nanomaterial, BPNSs show promising potential in tissue regeneration, attributed to their easy biodegradation and remarkable optical properties, and they can be used as effective photothermal agents.27,30
Acting as a temporary wound dressing, the C&BP-Patch can promote skin wound healing at multiple levels. The patch acts initially as a high-performance bio-sealant that can shield wound tissues from the external environment and create a moist and stable microenvironment for EMT and skin wound healing. Furthermore, the C&BP-Patch generates local heat under NIR laser (808 nm) irradiation which can eliminate bacteria, accelerate microcirculation and reduce inflammation to maintain the proper EMT via the PT effect. More importantly, the C&BP-Patch without any GFs can regulate the expression of Snail1 to induce partial EMT, which enables rapid epithelial cell migration to the injury site to rebuild the normal epidermal barrier. Therefore, we provide proof-of-principle evidence for the use of a GF-free NIR-responsive adhesive hydrogel to intrinsically promote EC migration via partial EMT for deep wound healing at multiple levels (Scheme 1).
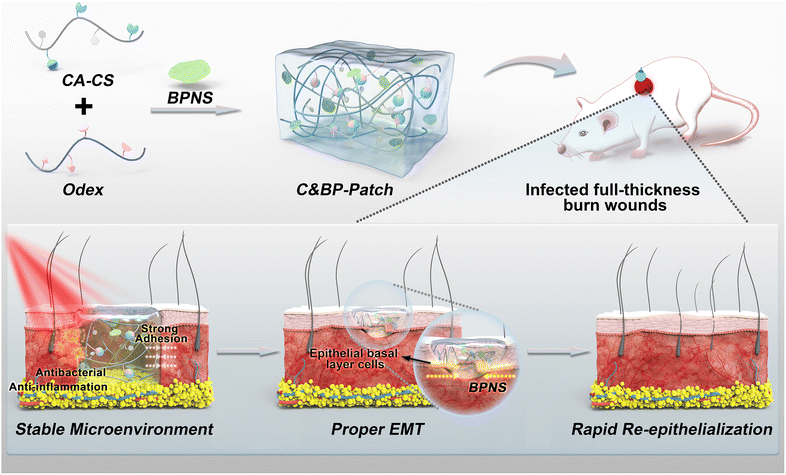 |
| Scheme 1 Schematic illustration of the GF-free NIR-responsive adhesive C&BP-Patch intrinsically inducing proper EMT (partial EMT) to enable rapid re-epithelialization for full-thickness infectious burn wound healing. | |
Results and discussion
Preparation and characterization of the C&BP-Patch
Transmission electron microscopy (TEM) showed that the nanostructure of the as-prepared BPNSs was a sheet-like morphology with an average size of about 20 nm (Fig. 1a). Atomic force microscopy (AFM) further verified the ultrathin structure of BPNSs with a thickness of about 2.2 nm (Fig. 1b and c), which proved that our synthesized BPNS was a 2D nanosheet. The scanning electron microscope (SEM) results showed that the porosity of the C&BP-Patch did not change obviously with the incorporation of BPNSs (Fig. 1d).
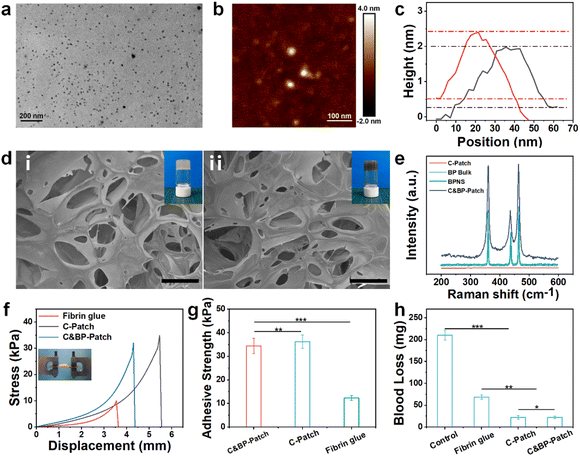 |
| Fig. 1 Characterization of C&BP-Patch. (a) TEM image of BPNS. (b) AFM image of BPNS. (c) Quantitative measurement of the thickness of BPNSs based on the AFM image. (d) SEM images of the C-Patch (i) and the C&BP-Patch (ii). Scale bar: 50 μm. (e) Raman spectrum of the C-Patch, the BP bulk, the BPNS, and the C&BP-Patch. (f) Typical stress–displacement curves of porcine skin bonded by hydrogels. (g) Adhesive strength of the hydrogels to porcine skin with hydrogels. (h) Hemostatic performance of the hydrogels. | |
Tube inverting test showed that the gelation speed did not vary significantly with the addition of BPNSs (Fig. 1d and S1a, ESI†), which ensured immediate gelation after injection through the double-barreled helical needle. At the same time, with the increase in the BPNS doping amount, the storage modulus of the C&BP-Patch did not increase markedly, which may be caused by a weaker conjugation effect with catechol due to the small doping mass of BPNSs (Fig. S1b, ESI†). The Raman spectrum results showed that the C&BP-Patch contained characteristic peaks at 358, 434, and 460 cm−1, which further indicated that BPNSs were indeed successfully incorporated into the C&BP-Patch matrix (Fig. 1e). The FT-IR spectra of the C&BP-Patch showed a strong peak at 1630 cm−1, which confirmed the formation of imine bonds (Fig. S1c, ESI†). Hence, the Raman spectrum and FT-IR spectra proved that we successfully synthesized the C&BP-Patch containing BPNSs.
The swelling test results indicated that the CA-CS/Odex hydrogel (C-Patch) and the C&BP-Patch swelled slightly due to hydration (Fig. S1d and S2, ESI†). Compared with the C-Patch, the swelling ratio of the C&BP-Patch was augmented with the increase in the BPNS content. This may be due to BPNS hydrolysis reducing the pH of the solution, which in turn alleviated the catechol complexation. The enhanced swelling ability of the C&BP-Patch allowed it to take in more fluids, dead cells, and other debris from the wound, while the exudate could be drained. We further evaluated the self-healing ability of the C&BP-Patch by a classical rheological shear recovery test. G′ and G′′ changed synchronously with strain alternation and this process was reproducible (Fig. S1e, ESI†). These results indicated that the C&BP-Patch exhibited excellent self-healing ability, and it could be administered with a 29G needle, which was beneficial for intraoperative administration (Fig. S3, ESI†).
Bio-multifunctional properties of the C&BP-Patch in vitro
The adhesion strength and hemostatic efficiency are critical factors in designing dressings for wound closure,31 which can insulate external interference during the preliminary stages of healing.32–34 By lap shear test, we found that the C&BP-Patch showed excellent skin adhesion ability (Fig. 1f). The adhesion strength of the C&BP-Patch was about 35 kPa, which was about 3 times greater than that of the fibrin glue (Fig. 1g). The remarkable adhesion performance of the hydrogel resulted from the modification with catechol groups. Therefore, the C&BP-Patch could stick on the wound surface to provide a hospitable and moist environment for the migration and survival of epithelial cells.
A rapidly in situ forming adhesive hydrogel is essential for efficient hemostasis due to its easy administration and minimal invasion.35 Hence, we established a mouse liver bleeding model to evaluate the hemostasis ability of the hydrogels (Fig. 1h). The blood loss in the C&BP-Patch group was 26 mg, while that in the control group was 217 mg, which demonstrated that the hydrogels had excellent hemostasis capability. Thus, the C&BP-Patch can be used as a clinically effective hemostatic dressing that significantly shortens bleeding time during wound treatment. When the temperature increased with NIR irradiation, the movement of polymer segments and water molecules was accelerated. The storage modulus of the C&BP-Patch dropped sharply, which facilitated the painless removal of wound dressings after administration (Fig. S1f, ESI†). Hence, the patch might be suggested for such simply and conveniently removable dressings, tending to be less painful and relieving some of the patient's pain.
To explore the biocompatibility of the C&BP-Patch, we tested the cytotoxicity of the hydrogel on L929 cells. The cell viability of all groups exceeded 85% during 72 h of co-culture (Fig. S5, ESI†). We also observed cell proliferation co-cultured with hydrogels for 48 h (Fig. S9, ESI†). The cells that were cultured directly with the C-Patch and the C&BP-Patch could proliferate equivalently to the control group. In the scratch assay, scratches were made with a pipette tip in each well of a 6-well plate, which was used to mimic the collective migration of wound healing in vivo to some extent. The cell scratch experiment showed that the migration of cells was augmented with the addition of the C&BP-Patch (Fig. S10a and b, ESI†), but the C&BP-Patch/PT group had no increase in the cell migration area compared to the C&BP-Patch. This might be due to the degradation of BPNSs that stimulates the myofibroblast contraction, thus leading to epithelial cell migration.36,37 Alterations of extracellular phosphate levels exert effects on surrounding tissues and phosphate itself can activate signal transduction to regulate cell behaviors for promoting cell migration.38,39 Thus, BPNSs contained in the C&BP-Patch could promote cell migration without the PT effect. These results demonstrated that the C&BP-Patch had excellent biocompatibility and was available for wound healing.
The PT effect and antibacterial activity of the C&BP-Patch
In the deep wound healing process, there is a high risk of external bacterial infection, which causes a severe delay of deep burn wound healing.40 In burn care, pathogens of wound infection, especially multi-drug resistant organisms, exhibit a major challenge.41 To improve the efficiency of burn treatment and reduce the overuse of antibiotics, antibacterial photothermal therapy was introduced into wound dressings. Considering the potential of BPNSs in PT-mediated antibacterial effects, we tested the anti-infection ability of the C&BP-Patch in vivo and in vitro.
As observed, under the NIR laser, the temperature of the C&BP-Patch gradually increased in a time-dependent manner in vitro (Fig. 2a). The PT effect of the C&BP-Patch was correlated with the concentration of BPNSs and the power of the NIR laser (Fig. 2b and c). It is worth noting that the C&BP-Patch had excellent photothermal stability and still retained a significant PT effect after five complete cycles of NIR laser irradiation (Fig. 2d). The C&BP-Patch and C&BP-Patch/PT could inhibit the growth of S. aureus, MRSA, E. coli, and P. aeruginosa on agar plates (Fig. 2e). In addition, the antibacterial effect of the C&BP-Patch was significantly enhanced with NIR laser, which was proved by its amplified mean diameter of the inhibition zone (Fig. 2g). Additionally, SEM showed that the number of attached bacteria on the surface of the hydrogel was significantly reduced and a large number of lysed bacteria could be observed in the C&BP-Patch/PT group (Fig. 2f). These results demonstrated that the antibacterial effect of C&BP-Patch/PT was enhanced markedly compared with the other groups. This antibacterial property is mainly because of the PT effect of BPNSs and the bacteriolysis effects of rich amino groups in chitosan that accelerate the cracking of a bacterial cytomembrane.42 Thus, the C&BP-Patch demonstrated wonderful antibacterial activity in vitro.
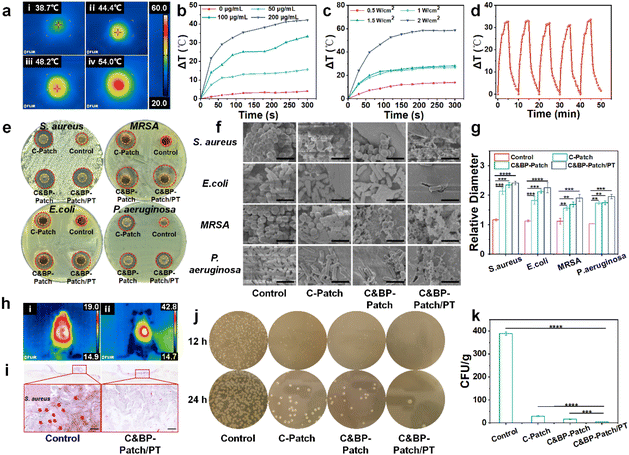 |
| Fig. 2 The C&BP-Patch eliminated bacteria through PT effects. (a) Thermal images of the C&BP-Patch under NIR irradiation in vitro. (b) Photothermal curves of the C&BP-Patch containing different concentrations of BPNS. (c) PT effect of the different radiant power of 808 nm laser. (d) Temperature curves of the C&BP-Patch under continuous NIR laser irradiation (5 cycles, 808 nm, 1.0 W cm−2, 300 s with laser and 300 s without laser for each cycle). (e) The C-Patch, C&BP-Patch, and C&BP-Patch/PT antibacterial rings of S. aureus, E. coli, MRSA, and P. aeruginosa on agar. The yellow circle and the red circle indicate the hydrogel and the scope of restraining fungi respectively. (f) The SEM images of S. aureus, E. coli, MRSA, and P. aeruginosa in different groups. Scale bar: 2 μm. (g) Statistical analysis of antibacterial rings’ relative diameter. (h) Thermal images of the C&BP-Patch under NIR irradiation in vivo. (i) Gram staining of S. aureus of infected wound tissue with the C&BP-Patch with NIR irradiation. The paraffin section of S. aureus was prepared for gram staining as the positive control. Red arrow: S. aureus. Scale bar: 20 μm. (j) and (k) The CFUs of skin burns with different groups and quantified analysis of CFUs. | |
On the skin surface, the temperature of burn wounds increased in a time-dependent manner when a NIR laser was applied in the C&BP-Patch group (Fig. 2h and S4, ESI†). Moreover, the temperature was raised gently on the wound surface under a NIR laser (41.2 ± 1.5 °C, 120 s) in the C&BP-Patch group, indicating that it could be conducive to clinical therapy (Fig. S4, ESI†). In addition, when the temperature is controlled to a mild extent (below 47 °C), it can inhibit the growth of MRSA and shows no obvious thermal damage to the wounds and surrounding tissue.43 In addition, cells stimulated at mild temperature might readily acquire resistance to thermal stress and markedly increase their survival rate.44
The gram staining of the control and C&BP-Patch/PT groups showed that Gram-positive bacteria were distributed in infected wounds obviously, and NIR laser irradiation could decrease bacterial colonies effectively (Fig. 2i). In addition, a microbial culture on agar from burn wound samples infected with S. aureus showed that the C&BP-Patch and C&BP-Patch/PT groups had fewer bacterial colonies compared with the control and C-Patch groups (Fig. 2j). The bacterial colony on the wound surface was reduced notably after NIR irradiation in the C&BP-Patch/PT group (Fig. 2k). These results demonstrate that the C&BP-Patch exhibited excellent anti-bacterial activity, and could alleviate the perturbance of epithelial cell migration when exposed to bacterial pathogens.11 Moreover, the mild photothermal hydrogel can enhance the heat resistance of cells,45 thereby promoting cell proliferation and differentiation for tissue repair, and ultimately accelerating wound healing.46 Similarly, the C&BP-Patch with a mild PT effect could increase the EC survival rate and be used for effectively treating infectious burn wounds in vivo.
The C&BP-Patch facilitated infectious full-thickness cutaneous burn wound healing under NIR
Burns are open wounds, which are mainly caused by heat.47 The disruption of the skin can lead to infection, dehydration, hypothermia, scarring, and shape changes. Furthermore, there are plenty of necrotic tissue fragments in the burn wound bed, which may be a natural medium for potential pathogens.48,49 Based on this, we established an infectious skin burnt model by applying a bacterial suspension on wound surfaces to evaluate the repairing efficiency of the C&BP-Patch under NIR (Fig. 3a). Then, we evaluated the progress of wound healing at different time points within 21 days after post-operation. Gross examination of the wounds at 14 d revealed a delay of wound closure in the control group compared with the C&BP-Patch and C&BP-Patch/PT (Fig. 3b). The areas of wounds in the C&BP-Patch/PT group shrank remarkably to 0.16 cm2 at 14 d (Fig. S6, ESI†).
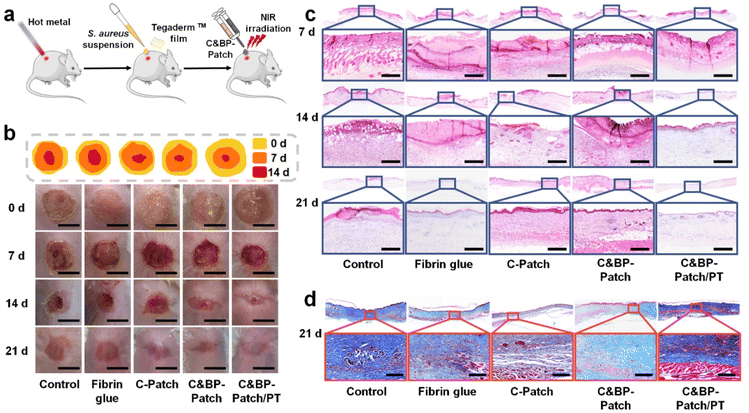 |
| Fig. 3 The C&BP-Patch facilitated cutaneous infectious burn wound healing under a NIR laser. (a) Schematic illustration of constructing an infectious burn wound model. (b) Repair of mouse skin burn wounds. Scale bar: 1 cm. (c) H&E staining of skin burns. Scale bar: 400 μm. (d) Masson's trichrome staining of skin burns on day 21. Scale bar: 200 μm. The type I collagen fibers were dyed blue. | |
H&E staining confirmed that the epidermis and dermis layers had been destroyed and eschar had formed on the wound surface of each group at 7 d (Fig. 3c). The remaining hydrogel left on burn wounds mixed with the eschar on day 7 with the exudation absorbed into the hydrogel, and degraded within 14 d (Fig. S11 and S12, ESI†). On day 14 post-wounding, the C&BP-Patch and C&BP-Patch/PT groups yielded accelerated healing kinetics, which resulted in faster EC migration and regenerated skin. The C&BP-Patch/PT group healed completely at 21 d with a mature epithelial morphology that formed hair follicles and sebaceous glands. In addition, the regenerated epidermis exhibited no obvious over-thickening at 21 d (Fig. 3c). The suggested C&BP-Patch can effectively facilitate re-epithelialization in infectious full-thickness cutaneous burn wound repair.
Burn injuries can extend deep to the dermis, causing damage to subdermal tissue, especially blood vessels.50 To investigate angiogenesis within the wound, we used immunohistochemical (IHC) staining to measure the expression of alpha smooth muscle actin (α-SMA) and CD31. We found that wounds treated with C&BP-Patch/PT demonstrated an increasing number of blood vessels in the vascular network layer (Fig. S7a and b, ESI†). A large number of α-SMA and CD31 positive cells were observed in the C&BP-Patch and C&BP-Patch/PT groups at 7 d. These results indicated that the C&BP-Patch along with the PT effect could promote burn wound healing by enhancing angiogenesis.
Collagen deposition is a common marker of wound healing. However, scar formation is also related to excessive collagen fiber synthesis and alignment in the presence of tensile stress generated by wound contraction.51 Thus, moderately ordered collagen deposition is conducive to scarless wound healing. Herein, we tested the deposition of collagen fibers by Masson's trichrome and Sirius red staining. As shown in Fig. 3d, the collagen deposition on the wound bed of C&BP-Patch/PT was relatively orderly and more continuous at 21 d. However, collagen fibers were annularly crosslinked and cicatrized in the control group without treatment. Sirius red staining showed that the neogenesis collagen fibers deposited as mature type I collagen at 14 d in the C&BP-Patch/PT group (Fig. S8a, ESI†). The remodeling rate of collagen fibers and the collagen content of the C&BP-Patch and C&BP-Patch/PT group were higher than those of the control group (Fig. S8b, ESI†). In scarless repair, collagen is deposited in a fine reticular pattern indistinguishable from the surrounding tissue.52 The decreased amount of crosslinked collagen fibers may lead to flexible wound healing that contributes to scarless repair. Overall, these results indicated that the C&BP-Patch could provide a stable microenvironment favorable for re-epithelialization, angiogenesis, and scarless wound healing.
The C&BP-Patch promoted inflammation resolution under NIR laser in burn wounds
During the inflammatory phase of the wound healing process, the innate immune system is activated by locally released cytokines to regulate the healing state.53,54 However, infection of burn wounds can lead to dysregulation of inflammation, and sustained inflammatory response will impair re-epithelialization.55–57 Therefore, to explore the effect of the C&BP-Patch under a NIR laser on inflammation, we assessed the expression of CD68, tumor necrosis factor-α (TNF-α), interleukin 1β (IL-1β), and IL-6 by IHC staining. Among them, CD68 is a transmembrane glycoprotein of monocytes and macrophages. TNF-α, IL-1β, and IL-6 are important pro-inflammatory cytokines that can delay wound healing.58
In this study, we found that there was a significantly decreased expression of those inflammatory factors in the C&BP-Patch/PT groups at 7 d (Fig. 4a). The C&BP-Patch/PT could preferably inhibit the expression of CD68, TNF-α, IL-1β, and IL-6 at 7 d (Fig. 4c). Because wound closure was almost completed at 14 d, the subcutaneous inflammatory response was significantly alleviated in all groups compared with that at 7 days post-surgery (Fig. 4b and d). These results demonstrated that the C&BP-Patch with a NIR laser could alleviate the inflammatory response during the early stage of healing. The anti-inflammation ability of the C&BP-Patch can provide a proper immune microenvironment for partial EMT during skin regeneration.
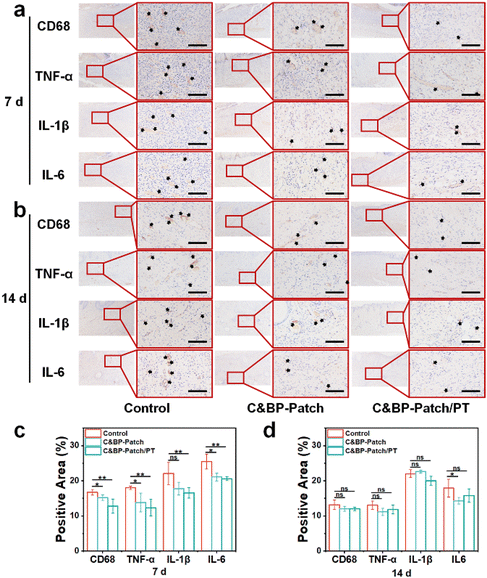 |
| Fig. 4 The C&BP-Patch promoted inflammation resolution in burn wound healing under NIR laser irradiation. (a) and (b) IHC staining of CD68, TNF-α, IL-1β, and IL-6 on days 7 and 14 post-surgery. Scale bar: 100 μm. The red blood cells were brown mainly due to the natural peroxidase in cells. (c) and (d) Percentage of the positive area of CD68, TNF-α, IL-1β, and IL-6 on days 7 and 14 post-surgery respectively. | |
The C&BP-Patch promoted epithelial migration through partial EMT
In epithelial wound healing, cells migrate from the edge of the wounds into damaged areas to re-establish normal tissue structure in a process referred to as partial EMT.59 During EMT, keratinocytes lose their epithelial characteristics and acquire mesenchymal characteristics, which are reflected in changes in gene expression and post-translational modification.60 During the healing process, we observed that the C&BP-Patch significantly facilitated epithelial cell migration to the wound bed (Fig. 5a). Morphometric analysis of wound sections on day 7 was carried out to evaluate the wound healing process at tissue levels (Fig. 5b). The quantification of the length of the newly formed epithelium (NFE) and the area of the hyperproliferative epidermis (HPE) covering the wound further confirmed that the C&BP-Patch group induced faster epithelial cell migration compared with the control group (Fig. 5c and d). Likewise, a decreased wound width (Fig. 5e) and a significant improvement in the wound closure rate (Fig. 5f) were achieved in the C&BP-Patch group. These results demonstrated the positive role of the C&BP-Patch in promoting rapid re-epithelialization in vivo.
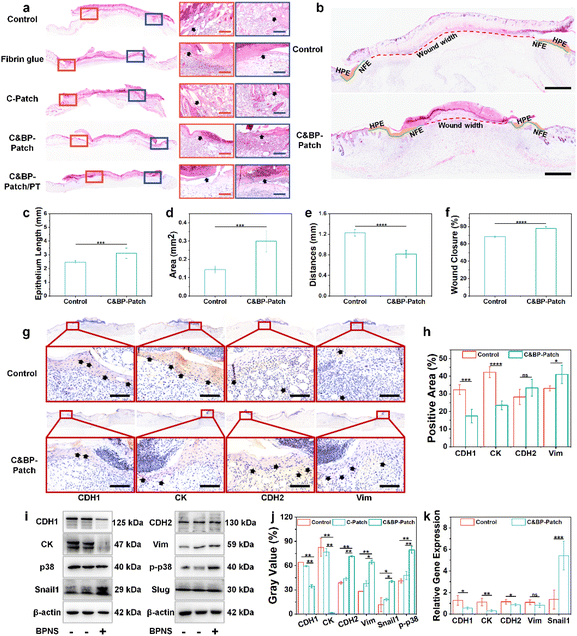 |
| Fig. 5 The C&BP-Patch accelerated epithelial migration through partial EMT. (a) Migration of ECs of skin burns on day 7 in vivo. Scale bar: 200 μm. (b) H&E staining of the control and C&BP-Patch groups on day 7 showing the morphometric analysis of the length of the newly formed epithelium (NFE, green line), area of the hyperproliferative epidermis (HPE), and wound width (red dotted line). Scale bar: 1 mm. (c–f) Statistics of day 7 wound sections for (c) re-epithelialization (length of NFE), (d) area of HPE, (e) wound width, and (f) percentage of wound closure (NFE/NFE + wound width × 100%) in the control and C&BP-Patch groups. (g) IHC staining of CDH1 (E-cadherin), CK (cytokeratin), CDH2 (N-cadherin), and Vim (vimentin) of histological sections in vivo. Scale bar: 100 μm. (h) Percentage of the positive area of CDH1, CK, CDH2, and Vim in IHC staining. (i) WB assay of EMT-related proteins CDH1, CK, p38/p-p38, CDH2, Vim, Snail1, and Slug (Snail2). (j) Corresponding quantitative data of the expression level of WB. (k) Relative gene expression of CDH1, CK, CDH2, Vim, and Snail1. | |
In this study, both epithelial markers (E-cadherin, occludin, and cytokeratin) and mesenchymal markers (N-cadherin and vimentin) were selected for evaluating the epithelial or mesenchymal state of keratinocytes in vitro and in vivo.9 In partial EMT during wound healing, cell adhesion of the epidermis is characterized by diminished desmosome components and adherent junction components, such as E-cadherin.61 IHC staining proved that E-cadherin (CDH1) and cytokeratin (CK) positive cells decreased, while vimentin (Vim) positive cells increased in the C&BP-Patch group in vivo (Fig. 5g and h), but the positive expression of N-cadherin (CDH2) showed no significant change in vivo. The results of real-time fluorescence quantitative reverse transcription-polymerase chain reaction (RT-PCR) and western blotting (WB) explicated that the expression of CDH1 and CK decreased, while the expression of CDH2 and Vim increased with the incorporation of BPNSs (Fig. 5i, j, and k). These results suggested that BPNSs within the C&BP-Patch could promote the partial EMT of keratinocytes both in vivo and in vitro.
At present, convincing evidence shows that Snail1 and Slug (Snail2) closely correlate with EMT via p38 and phosphorylated p38 signaling.60,62–64 The induction of phosphorylated p38 regulates the activity of Snail transcription factors.65 As displayed in Fig. 5i, j, and k, the expression of Snail1 and phosphorylated p38 was increased with the addition of C&BP-Patch, while the expression of Slug showed no obvious change. In addition, the expression of α-SMA was elevated in the C&BP-Patch treated group in vivo (Fig. S7, ESI†), which could significantly promote epithelial cell migration.66 Taken together, the C&BP-Patch could induce the partial EMT of keratinocytes in the burn wound healing process, which enabled rapid epithelial cell migration to the injury site to rebuild the normal epidermal barrier.
Conclusions
Herein, we developed a NIR-responsive black phosphorus-based adhesive hydrogel patch with intrinsic partial EMT-inducive activity. This novel stimuli-responsive GF-free hydrogel patch can effectively promote infectious full-thickness burn wound healing at multiple levels. The C&BP-Patch coated on the wound beds as bio-sealants can protect burn injuries from the external environment to provide a stable microenvironment for EMT and tissue regeneration. Furthermore, the C&BP-Patch generating local heat under NIR laser (808 nm) irradiation will eliminate bacteria, simultaneously accelerate microcirculation and maintain a moderate inflammatory response. More importantly, the C&BP-Patch can dramatically promote partial EMT of ECs. Overall, our study demonstrated that the NIR-responsive BPNS-modified hydrogel patch could effectively promote rapid re-epithelialization and enable complete skin regeneration via inducing proper EMT (partial EMT). Thus, the NIR-responsive C&BP-Patch displayed promising therapeutic efficacy for infected burn wound healing.
Experimental section
Materials
Chitosan (degree of deacetylation ∼95%, 100–200 MPs), dextran (Mw: ∼70 kDa), 3,4-dihydroxy hydro cinnamic acid, and 1-ethyl-3-(3-dimethylaminopropyl)-carbodiimide hydrochloride (EDC) were purchased from Aladdin (Shanghai, China). N-Methyl pyrrolidone (NMP) and sodium periodate (NaIO4) were purchased from Acros Bio-Technology Co., Ltd. Black phosphorus was purchased from XFNANO (Nanjing, China). The Cell Counting Kit was purchased from Invigentech (CA, USA). Masson's trichrome staining was purchased from Solarbio (Beijing, China). Picro Sirius red solution was purchased from G-CLONE (Beijing, China). The Streptavidin–biotin–peroxidase (SP) Immunohistochemical Stain Kit was purchased from ZSGB-Bio (Beijing, China). Primary antibodies were purchased from Bioss (Beijing, China) and Proteintech (Rosemont, IL, USA). Secondary antibodies were obtained from Proteintech (Rosemont, IL, USA). ICR mice were obtained from Liaoning Changsheng Biotechnology Co. (Benxi, China). Other chemical reagents were analytically pure and no further purification was required.
Preparation of BPNS
First, 50 mg of BP powder was added to a 100 mL centrifuge tube filled with 50 mL of NMP. Then BP powder was stripped with a sonic grinder probe for 6 h (450 W, 25 kHz). The obtained dispersion was centrifuged at 7500 rpm for 20 min, then the supernatant was slowly introduced into a high-speed centrifuge tube and centrifuged at 12
000 rpm for 20 min, and then the supernatant was discarded. Finally, the centrifuging sedimentation was cleaned three times through redispersion under ultrasonication followed by centrifugation. The temperature was controlled below 4 °C throughout the whole process of preparation.
Synthesis of the C-Patch and the C&BP-Patch
The preparation of CA-CS and Odex was reported previously.27,29 In brief, CA-CS was synthesized via the EDC activation reaction between NH2 groups in the chitosan and carboxyl groups in hydro-cinnamic acid, and the degree of functional group grafting onto chitosan was about 20%. Odex was synthesized by oxidation of dextran with sodium periodate, and the oxidation degree was about 35%. Then CA-CS (20 mg) and Odex (40 mg) were dissolved in PBS (1 mL) respectively for preparing adhesive hydrogel patches. The C-Patch was prepared by mixing CA-CS and Odex solutions directly at equal volumes. For the preparation of the C&BP-Patch, 500 μL of BPNS solution (200, 400, and 800 μg mL−1, respectively) was mixed with 500 μL of Odex solution first to get Odex-BP, and then CA-CS solution (1 mL) was added to obtain the C&BP-Patch. The gel time was verified by a tube inverting test.
Characterization of the C&BP-Patch
FT-IR spectra analysis.
The C-Patch and the C&BP-Patch were freeze-dried, mixed with KBr, and ground into fine powder. The powder was analyzed using a spectrophotometer in the range of 400–4000 cm−1.
Raman spectra analysis.
The C-Patch and the C&BP-Patch were freeze-dried first and then analyzed using a Horiba-Jobin Yvon LabRAM ARAMIS. The system has a 633 nm diode laser and a resolution of ca. 4 cm−1. For each sample, three different regions were scanned and the resulting spectra were averaged.
Injectability assessment.
The mixture of CA-CS (500 μL, 20 mg mL−1) and Odex-BP (500 μL, containing 200 μg mL−1 BPNS and 20 mg mL−1 Odex) was added to different channels of the syringe and injected through a spiral needle. When injecting the C&BP-Patch using a single-channel needle (diameter: 29 G), CA-CS and Odex-BP were mixed first for about 10 s, and then the hydrogel mixture was suctioned into the syringe and extruded outside.
Rheological test.
C-Patch and C&BP-Patch disks were prepared with 30 mm diameter and 3 mm height. The storage modulus and the loss modulus of the hydrogel were obtained using parallel 25 mm diameter plate geometry. The self-healing property of the C&BP-Patch was tested using the rheological test. The temperature was maintained constant at 25 °C. Oscillatory stress sweeps at 1 Pa were carried out at a frequency of 0.1 Hz to determine the linear viscoelastic range. The G′ and G′′ values of the patches in continuous step strain were recorded at a fixed frequency of 10 rad s−1. Each strain interval was set as 150 s.
Morphological features.
The microstructure of the C-Patch and the C&BP-Patch was assessed via SEM. The lyophilized fractured pieces of the C-Patch and the C&BP-Patch (0.3 × 0.3 × 0.2 cm3) were secured on brass stubs with double-sided conductive sticky tabs and sputtered with gold, and then observed with a JEOL FESEM 6700F SEM operated at 3 kV.
Swelling rate analysis.
The C-Patch (1 mL) and C&BP-Patch (1 mL) disks were placed in Petri dishes with a diameter of 9 cm contained within 10 mL of PBS for 30 min. Then the hydrogel was taken out of the Petri dish and weighed after removing the excess PBS on the surface. The swelling rate (SR) was calculated using the following formula:
SR (%) = (Wt − W0)/W0 × 100% |
where Wt is the weight of the hydrogel taken out of the Petri dish at different time points, and W0 is the initial weight of the wet hydrogel. The experiment was repeated three times.
Adhesive and hemostatic ability of the C&BP-Patch.
Fresh pig skin was used to test the adhesive strength between the hydrogel and host tissue. The fresh pig skin was fully washed and cut into rectangles (20 × 40 mm2). The C-Patch (1 mL) and the C&BP-Patch (1 mL) were injected onto the surface of pig skin respectively, and then the other piece of skin was buttoned up on it. The adhesion area was 20 × 10 mm2 and the adhesive strength was tested with a tensile machine. All these tests were carried out three times.
The liver hemorrhagic model of ICR mice (female, weight 20–30 g) was used to observe the hemostatic property of the hydrogels. Briefly, the mice were anesthetized with 4% w/v chloral hydrate and fixed on a surgical board. The mouse liver was exposed by an abdominal incision, and extra fluid around the liver was carefully removed. A pre-weighed filter paper was placed under the liver. Bleeding of the liver was induced using a scalpel incision with the board tilted at an angle of about 30°. The C-Patch and the C&BP-Patch were pre-mixed for 10 s, and then the C-Patch or C&BP-Patch solution was immediately injected onto the bleeding site with a syringe. Those left untreated after cutting the liver served as controls. After 10 min, the blood-absorbed filter paper was weighed with electronic-analytical balances.
Assessment of PT properties
The PT properties of the C&BP-Patch were assessed both in vitro and in vivo. 300 μL of the C&BP-Patch hydrogel was added to a 24-well plate to evaluate its PT effects in vitro. 500 μL of the C&BP-Patch hydrogel was applied to burn skin defects of mice to evaluate its PT effects in vivo. The temperature change of the hydrogel under irradiation with a fiber-coupled 808 nm diode laser (MDL-H-808/5000 mV, China) was monitored using a thermal infrared imaging camera (FLIR-E6, USA).
Cytotoxicity test
The extract solutions of the hydrogels were used to detect their cytotoxicity on L929 cells. Briefly, the extracts were prepared by immersing the dried C-Patch and the C&BP-Patch (containing 50, 100, and 200 μg mL−1 BPNS, respectively) in the medium for 24 h at 37 °C. The L929 cells were seeded into a 96-well plate (5000 cells per well), and pre-cultured for 12 h before replacing the culture medium with the fresh medium containing a series of different concentrations of hydrogel extract solutions. Cells seeded on the plate without extract solution served as the control group. After being cultured for 24 h, 48 h, and 72 h, the cell viability was evaluated by a Cell Counting Kit assay. The absorbance value at 590 nm was measured using a microplate reader.
Scratch assay
HaCaT cells were seeded with 200
000 cells per well in a 6-well plate and cultured until complete confluence. Three parallel scratches were made with a pipette tip in each well. Then the medium was removed, and 2 mL of the FBS-free medium containing the C-Patch or the C&BP-Patch (containing 200 μg mL−1 BPNS) was added to each well. Half of the wells with the C&BP-Patch added were irradiated with a NIR laser for 2 min. After 24 h and 48 h, the scratched area was observed using a microscope (IX83, Olympus, Tokyo, Japan) and analyzed with the Image J (V1.53, NIH, Bethesda, USA).
Western blotting (WB)
WB analysis was performed to assess the levels of EMT-related proteins in vitro. Firstly, the sterilized hydrogel extract solutions were prepared by immersing the dried C-Patch and the C&BP-Patch (containing 200 μg mL−1 BPNS) in the medium for 24 h at 37 °C. Then, HaCaT cells were seeded in a 6-well plate (100
000 cells per well) and pre-cultured for 12 h before replacing the culture medium with the fresh medium containing hydrogel extract solutions (2 mL). After being cultured for 5 d, cells were lysed in RIPA buffer to obtain cellular protein. The protein extracts were run on SDS-PAGE and then transferred to a polyvinylidene fluoride membrane. The blot was blocked with 5% skim milk for 2 h at room temperature and incubated with primary antibodies overnight at 4 °C. The following primary antibodies were used: anti-β-actin (Proteintech, 66009-1-AP, dilution, 1
:
5000), anti-E-cadherin (Proteintech, 20874-1-AP, dilution, 1
:
10000), anti-vimentin (Proteintech, 10366-1-AP, dilution, 1
:
5000), anti-Snail1 (Bioss, bs-1371R, dilution, 1
:
1000), anti-Snail2 (Proteintech, 12129-1-AP, dilution, 1
:
1000), anti-cytokeratin (Proteintech, 10143-1-AP, dilution, 1
:
1000), anti-N-cadherin (Proteintech, 22018-1-AP, dilution, 1
:
3000), anti-p38 (CST, 9212, dilution, 1
:
1000), and anti-p-p38 (CST, 9211, dilution, 1
:
1000). Then, secondary antibodies were added and incubated for 1 h at room temperature. The blot was stripped with stripping buffer according to the user guide. The signal was detected using an enhanced chemiluminescence reagent, and band density was analyzed with Image J (V1.53, NIH, Bethesda, USA).
Real-time RT-PCR analysis
EMT-related genes were analyzed by real-time RT-PCR in vitro. Firstly, the sterilized hydrogel extract solutions were prepared by immersing the dried C-Patch and C&BP-Patch (containing 200 μg mL−1 BPNS) in the medium for 24 h at 37 °C. Then, HaCaT cells were seeded in a 6-well plate (100
000 cells per well), and pre-cultured for 12 h before replacing the culture medium with the fresh medium containing hydrogel extract solutions. After being cultured for 5 d, total RNA was isolated from cells with TRIeasy™ LS Total RNA Extraction Reagent (Yeasen, 19201ES60, Shanghai, China). The quality of RNA was determined at the ratio 260/280. RNA was reverse transcribed with the Hifair® II 1st Strand cDNA Synthesis SuperMix for qPCR (gDNA digester plus) (Yeasen, 11123ES60, Shanghai, China). The Hieff® qPCR SYBR Green Master Mix (Yeasen, 11202ES08, Shanghai, China) was used for real-time PCR analysis. Relative differences in gene expression between groups were expressed using cycle time (Ct) values. These Ct values were first normalized with those of GAPDH in the same sample and expressed as fold change compared to control (100%). GAPDH was used as an internal control of the reaction. The expression levels of CDH1, CK, CDH2, Vim, and Snail1 were determined using a two-color real-time PCR detection system (Bio-Rad, CFX96, Hercules, CA) according to the manufacturer's instructions. The primer sequences used are mentioned in Table S1.†
Animal experiments
A mouse full-thickness burn wound infection model was employed to evaluate the effect of the C&BP-Patch on wound healing. All animal procedures were performed in accordance with the Guidelines for Care and Use of Laboratory Animals of Jilin University and approved by the Jilin University Animal Care and Use Committee (20200680). 100 ICR mice (female, body weight 20–30 g) were divided into five groups randomly, including the blank control group, fibrin glue group, C-Patch group, C&BP-Patch (containing 200 μg mL−1 BPNS) group, and C&BP-Patch/PT (containing 200 μg mL−1 BPNS) group, with 5 mice for each group (n = 5). The mice were anesthetized and the hairs on their back were shaved with electric clippers. An iron rod (1.5 cm in diameter), which was heated in a flame for 2 min, was placed on the skin for 10 s to create a full-thickness burn injury. The bacterial suspension (50 μL, 100
000 CFU mL−1) was applied to the wound surface evenly. Then the wound infected area was coated with TegadermTM films. After 24 h, the films were removed and the defects were covered with fibrin glue, the C-Patch, and the C&BP-Patch. The C&BP-Patch/PT group was irradiated with an infrared laser for 2 min. The control group had no treatment after burn wound infection. The mice were sacrificed at days 7, 14 and 21 for histopathological examination. The wound areas were determined by calculating their surface area. A ruler was positioned to calibrate the magnification of wound area photographs during measurement analysis. The wound tissues were collected and immersed in 4% formaldehyde for H&E staining, Masson's trichrome staining, and Sirius red staining. The tissue sections of defect areas were photographed using a microscope (BX53, Olympus, Tokyo, Japan) and measured using Image J (V1.53, NIH, Bethesda, USA). The amount of formed collagen fibers of the wound in different groups at each time point was calculated using the formula: collagen fiber (%) = A0[type I collagen area]/A1[total tissue area] × 100%.
Antibacterial property analysis
S. aureus, MRSA, E. coli, and P. aeruginosa were inoculated in Brain Heart Infusion Broth (BHI, Qingdao Hope Bio-Technology Co., Ltd, China) and cultured aerobically for 12 h at 37 °C.
After generating the burn and infection in mice, a small piece of dorsal skin (about 2 × 2 mm2 in size) of the defects was cut out with scissors and immersed in sterile PBS. The dorsal skin samples were homogenized in PBS and filtered with cell strainers. The filtered liquid of the homogenized skin extract in PBS was spread on BHI agar plates. The number of CFUs (colony-forming units) was counted and photographed to obtaining images.
The C-Patch (200 μL) and the C&BP-Patch (200 μL, containing 200 μg mL−1 BPNS) were immersed in 500 μL of the bacterial suspension in BHI (100
000 CFU mL−1) and half of the C&BP-Patch group was irradiated with an infrared laser for 2 min. After incubating at 37 °C for 24 h, the bacterial suspension was centrifuged at 4500 rpm for 10 min, then the supernatant was removed and the samples were gently washed with PBS. For SEM, the samples were fixed with 2.5% glutaraldehyde solution for 4 h and serially dehydrated with ethanol (30–100% for 15 min each) at room temperature. Lyophilized fractured samples were secured on brass stubs with double-sided conductive sticky tabs, sputtered with gold, and observed with a JEOL FESEM 6700F SEM operated at 3 kV.
The bacterial suspension (100 μL, 100
000 CFU mL−1) was spread on a BHI agar plate. The C-Patch (500 μL) and the C&BP-Patch (500 μL, containing 200 μg mL−1 BPNS) were placed in pre-punched holes (diameter: 10 mm) on the agar plates. Half of the C&BP-Patch group was irradiated with an infrared laser for 2 min. After incubation at 37 °C for 24 h, the diameter of the inhibition zone was measured. The relative diameter (RD) was used to indicate the antibacterial activity, which was calculated using the formula RD = D1/D2, where D1 and D2 refer to the diameter of the inhibition zone and the hydrogel disk, respectively.
IHC staining
To determine the angiogenesis and inflammatory state of burnt skin, IHC staining was used to visualize the vascular markers (α-SMA and CD31) and inflammatory factors (CD68, TNF-α, IL-1β, and IL-6). The EMT-related markers were also visualized by IHC staining for CDH1, CK, CHD2, and Vim. The skin sections were subjected to immunostaining according to the instructions of a Streptavidin–biotin–peroxidase (SP) immunohistochemical stain kit with slight modifications. In detail, the skin slides were heated in sodium citrate solution for antigen repair, blocked with 5% goat serum albumin, followed by incubation of antibodies, CD31 antibody (Proteintech, 28083-1-AP, dilution, 1
:
1000), α-SMA antibody (Proteintech, 14395-1-AP, dilution, 1
:
5000), CD68 antibody (Proteintech, 28058-1-AP, dilution, 1
:
1000), TNF-α antibody (Bioss, bs-10802R, dilution, 1
:
400), IL-1β antibody (Bioss, bs-0812R, dilution, 1
:
400), IL-6 antibody (Proteintech, 21865-1-AP, dilution, 1
:
400), E-cadherin antibody (Proteintech, 20874-1-AP, dilution, 1
:
4000), vimentin antibody (Proteintech, 10366-1-AP, dilution, 1
:
3000), cytokeratin antibody (Proteintech, 10143-1-AP, dilution, 1
:
200), and N-cadherin antibody (Proteintech, 22018-1-AP, dilution, 1
:
2000), and then incubated with horseradish peroxidase (HRP) conjugated Goat anti-Mouse/Rabbit IgG. Finally, the slides were stained with diaminobenzidine (DAB) solution and hematoxylin. The histological images were observed using a microscope (BX53, Olympus, Tokyo, Japan).
Statistical analysis
All experiments were repeated at least 3 times. All the data are presented as mean ± standard deviations (SD). Student's t-tests and one-way ANOVA analysis were performed to evaluate the statistical significance among the groups. P < 0.05 was regarded as statistical significance.
Conflicts of interest
There are no conflicts to declare.
Acknowledgements
This study was supported by the Science and Technology Development Project of Jilin Province (20210101251JC), the Science and Technology Research Projects of the Education Office of Jilin Province (JJKH20211217KJ), the Interdisciplinary Integration and Innovation Project of Jilin University (JLUXKJC2020309), the Project of Changchun Science and Technology Bureau (21ZGM24), the National Natural Science Foundation of China (no. 21975098), and the Interdisciplinary Integration and Innovation Project of JLU (JLUXKJC2021ZZ07).
References
- M. G. Jeschke, M. E. van Baar, M. A. Choudhry, K. K. Chung, N. S. Gibran and S. Logsetty, Nat. Rev. Dis. Primers, 2020, 6, 1–25 CrossRef.
- Y. Wang, J. Beekman, J. Hew, S. Jackson, A. C. Issler-Fisher, R. Parungao, S. S. Lajevardi, Z. Li and P. K. M. Maitz, Adv. Drug Delivery Rev., 2018, 123, 3–17 CrossRef CAS PubMed.
- M. Farahani and A. Shafiee, Adv. Healthcare Mater., 2021, 10, 2100477 CrossRef CAS.
- K. F. Cutting, J. Wound Care, 2010, 19, 4–9 CrossRef.
- J. Boateng and O. Catanzano, J. Pharm. Sci., 2015, 104, 3653–3680 CrossRef CAS.
- M. Rodrigues, N. Kosaric, C. A. Bonham and G. C. Gurtner, Physiol. Rev., 2019, 99, 665–706 CrossRef CAS.
- G. C. Gurtner, S. Werner, Y. Barrandon and M. T. Longaker, Nature, 2008, 453, 314–321 CrossRef CAS PubMed.
- E. M. Golebiewska and A. W. Poole, Blood Rev., 2015, 29, 153–162 CrossRef CAS PubMed.
- S. Enoch and D. J. Leaper, Surgery, 2005, 23, 37–42 Search PubMed.
- R. Edward and K. G. Harding, Curr. Opin. Infect. Dis., 2004, 17, 91–96 CrossRef.
- M. Zheng, S. Sun, J. Zhou and M. Liu, J. Clin. Lab. Anal., 2021, 35, e23627 CAS.
- S. B. Chaney, K. Ganesh, S. Mathew-Steiner, P. Stromberg, S. Roy, C. K. Sen and D. J. Wozniak, Wound Repair Regener., 2017, 25, 541–549 CrossRef PubMed.
- J. P. Thiery, H. Acloque, R. Y. Huang and M. A. Nieto, Cell, 2009, 139, 871–890 CrossRef CAS PubMed.
- H. Acloque, M. S. Adams, K. Fishwick, M. Bronner-Fraser and M. A. Nieto, J. Clin. Invest., 2009, 119, 1438–1449 CrossRef CAS.
- M. A. Nieto, R. Y. J. Huang, R. A. Jackson and J. P. Thiery, Cell, 2016, 166, 21–45 CrossRef CAS.
- F. L. Yuan, Z. L. Sun, Y. Feng, S. Y. Liu, Y. Du, S. Yu, M. L. Yang and G. Z. Lv, J. Cell. Physiol., 2019, 234, 21662–21669 CrossRef CAS PubMed.
- R. Weiskirchen, S. Weiskirchen and F. Tacke, Mol. Aspects Med., 2019, 65, 2–15 CrossRef CAS.
- S. Yamakawa and K. Hayashida, Burns Trauma, 2019, 7, 1–13 Search PubMed.
- B. A. Mast and G. S. Schultz, Wound Repair Regener., 1996, 4, 411–420 CrossRef CAS.
- C. E. Weber, N. Y. Li, P. Y. Wai and P. C. Kuo, J. Burn Care Res., 2012, 33, 311–318 CrossRef PubMed.
- Y. Liang, J. He and B. Guo, ACS Nano, 2021, 15, 12687–12722 CrossRef CAS PubMed.
- Y. S. Zhang and A. Khademhosseini, Science, 2017, 356, eaaf3627 CrossRef PubMed.
- W. Zhang, R. Wang, Z. Sun, X. Zhu, Q. Zhao, T. Zhang, A. Cholewinski, F. Yang, B. Zhao, R. Pinnaratip, P. K. Forooshani and B. P. Lee, Chem. Soc. Rev., 2020, 49, 433–464 RSC.
- Y. H. Xiong, L. Zhang, Z. Xiu, B. Yu, S. Duan and F. J. Xu, Acta Biomater., 2022, 148, 119–132 CrossRef CAS.
- A. Maleki, J. He, S. Bochani, V. Nosrati, M. A. Shahbazi and B. Guo, ACS Nano, 2021, 15, 18895–18930 CrossRef CAS PubMed.
- B. Sui, Y. Zhang, L. Huang, Y. Chen, D. Li, Y. Li and B. Yang, ACS Sustainable Chem. Eng., 2020, 8, 18492–18499 CrossRef CAS.
- J. H. Ryu, H. J. Kim, K. Kim, G. Yoon, Y. Wang, G. S. Choi, H. Lee and J. S. Park, Adv. Funct. Mater., 2019, 29, 1900495 CrossRef.
- X. Tang, X. Wang, Y. Sun, L. Zhao, D. Li, J. Zhang, H. Sun and B. Yang, Adv. Funct. Mater., 2021, 31, 2105718 CrossRef CAS.
- J. P. Dray, B. Delaey, A. Van de Voorde, A. Van Den Bulcke, B. Bogdanov and E. Schacht, Biomaterials, 1998, 19, 99–107 CrossRef.
- R. Yu, H. Zhang and B. Guo, Nano-Micro Lett., 2022, 14, 1 CrossRef CAS PubMed.
- Y. Q. Wang, X. Y. Dou, H. F. Wang, X. Wang and D. C. Wu, Chin. J. Polym. Sci., 2021, 39, 1421–1430 CrossRef CAS.
- B. Guo, R. Dong, Y. Liang and M. Li, Nat. Rev. Chem., 2021, 5, 773–791 CrossRef CAS.
- L. Liu, E. Hu, K. Yu, R. Xie, F. Lu, B. Lu, R. Bao, Q. Li, F. Dai and G. Lan, Biomater. Sci., 2021, 9, 7343–7378 RSC.
- D. Du, X. Chen, C. Shi, Z. Zhang, D. Shi, D. Kaneko, T. Kaneko and Z. Hua, Acta Biomater., 2021, 136, 223–232 CrossRef CAS.
- H. Ye, Y. Xian, S. Li, C. Zhang and D. Wu, Biomater. Sci., 2022, 10, 4218–4227 RSC.
- A. J. Dahlstedt, A. Katz and H. Westerblad, J. Physiol., 2001, 533, 379–388 CrossRef CAS PubMed.
- F. C. Tsai, G. H. Kuo, S. W. Chang and P. J. Tsai, BioMed Res. Int., 2015, 2015, 1–13 Search PubMed.
- T. Michigami, M. Kawai, M. Yamazaki and K. Ozono, Physiol. Rev., 2018, 98, 2317–2348 CrossRef CAS PubMed.
- L. Guo, C. Xu, D. Li, X. Zheng, J. Tang, J. Bu, H. Sun, Z. Yang, W. Sun and X. Yu, PLoS One, 2015, 10, 1–17 Search PubMed.
- J. A. D'Abbondanza and S. Shahrokhi, Surg. Infect., 2021, 22, 58–64 CrossRef.
- C. D. Laurie, B. K. Hogan, C. K. Murray, F. L. Loo, D. R. Hospenthal, L. C. Cancio, S. H. Kim, E. M. Renz, D. Barillo, J. B. Holcomb, C. E. Wade and S. E. Wolf, Burns, 2010, 36, 773–779 CrossRef.
- R. Singh, K. Shitiz and A. Singh, Int. Wound J., 2017, 14, 1276–1289 CrossRef PubMed.
- X. Dong, J. Ye, Y. Chen, T. Tanziela, H. Jiang and X. Wang, Chem. Eng. J., 2022, 432, 134061 CrossRef CAS.
- G. Cheng and B. Li, Mater. Today Adv., 2020, 6, 100049 CrossRef.
- L. Ma, X. Feng, H. Liang, K. Wang, Y. Song, L. Tan, B. Wang, R. Luo, Z. Liao, G. Li, X. Liu, S. Wu and C. Yang, Mater. Today, 2020, 36, 48–62 CrossRef CAS.
- X. Zhang, B. Tan, Y. Wu, M. Zhang and J. Liao, Polymers, 2021, 13, 2100 CrossRef CAS PubMed.
- Y. Yao, A. Zhang, C. Yuan, X. Chen and Y. Liu, Biomater. Sci., 2021, 9, 4523–4540 RSC.
- A. B. Wysocki, AACN Adv. Crit. Care, 2002, 13, 382–397 Search PubMed.
- Y. Huang, L. Mu, X. Zhao, Y. Han and B. Guo, ACS Nano, 2022, 16, 13022–13036 CrossRef CAS PubMed.
- S. A. Eming, B. Brachvogel, T. Odorisio and M. Koch, Prog. Histochem. Cytochem., 2007, 42, 115–170 CrossRef CAS.
- L. Yang, T. M. Witten and R. M. Pidaparti, J. Theor. Biol., 2013, 332, 228–248 CrossRef PubMed.
- A. Leung, T. M. Crombleholme and S. G. Keswani, Curr. Opin. Pediatr., 2012, 24, 371–378 CrossRef PubMed.
- S. K. Shukla, A. K. Sharma, V. Gupta and M. H. Yashavarddhan, J. Tissue Viability, 2019, 28, 218–222 CrossRef.
- D. Wang, X. Wang, L. Huang, Z. Pan, K. Liu, B. Du, Y. Xue, B. Li, Y. Zhang, H. Wang, D. Li and H. Sun, Adv. Healthcare Mater., 2021, 10, e2101586 CrossRef.
- A. Krejner, M. Litwiniuk and T. Grzela, Chronic Wound Care M., 2016, 3, 29–39 Search PubMed.
- T. M. Oberyszyn, Front. Biosci., 2007, 12, 2993–2999 CrossRef CAS.
- P. Rousselle, F. Braye and G. Dayan, Adv. Drug Delivery Rev., 2019, 146, 344–365 CrossRef CAS PubMed.
- S. Ellis, E. J. Lin and D. Tartar, Curr. Dermatol. Rep., 2018, 7, 350–358 CrossRef PubMed.
- T. J. Shaw and P. Martin, Curr. Opin. Cell Biol., 2016, 42, 29–37 CrossRef CAS PubMed.
- J. Yang, P. Antin, G. Berx, C. Blanpain, T. Brabletz, M. Bronner, K. Campbell, A. Cano, J. Casanova, G. Christofori, S. Dedhar, R. Derynck, H. L. Ford, J. Fuxe, A. G. de Herreros, G. J. Goodall, A. K. Hadjantonakis, R. Y. J. Huang, C. Kalcheim, R. Kalluri, Y. Kang, Y. Khew-Goodall, H. Levine, J. Liu, G. D. Longmore, S. A. Mani, J. Massagué, R. Mayor, D. McClay, K. E. Mostov, D. F. Newgreen, M. A. Nieto, A. Puisieux, R. Runyan, P. Savagner, B. Stanger, M. P. Stemmler, Y. Takahashi, M. Takeichi, E. Theveneau, J. P. Thiery, E. W. Thompson, R. A. Weinberg, E. D. Williams, J. Xing, B. P. Zhou and G. Sheng, Nat. Rev. Mol. Cell Biol., 2020, 21, 341–352 CrossRef CAS.
- Y. Koike, M. Yozaki, A. Utani and H. Murota, Sci. Rep., 2020, 10, 1–13 CrossRef.
- J. Zhu, Y. Zheng, H. Zhang, Y. Liu, H. Sun and P. Zhang, Am. J. Transl. Res., 2019, 11, 3862–3878 CAS.
- L. G. Hudson, C. Choi, K. M. Newkirk, J. Parkhani, K. L. Cooper, P. Lu and D. F. Kusewitt, Mol. Carcinog., 2007, 46, 257–268 CrossRef CAS.
- H. W. Yang, S. A. Lee, J. M. Shin, I. H. Park and H. M. Lee, Sci. Rep., 2017, 7, 1–12 CrossRef PubMed.
- Z. Wang, L. Liu, W. Bu, M. Zheng, N. Jin, K. Zhang, X. Xu, D. Zhou, B. Yang and H. Sun, Adv. Funct. Mater., 2020, 30, 1–16 Search PubMed.
- Y. Si, J. Wang, J. Guan, Q. Han and Y. Hui, J. Ocul. Pharmacol. Ther., 2013, 29, 310–318 CrossRef CAS PubMed.
|
This journal is © The Royal Society of Chemistry 2023 |