DOI:
10.1039/D2BM01081J
(Paper)
Biomater. Sci., 2023,
11, 195-207
Ultra-small NIR J-aggregates of BODIPY for potent phototheranostics†
Received
11th July 2022
, Accepted 2nd November 2022
First published on 9th November 2022
Abstract
Cancer phototheranostics that combines diagnosis with phototherapy has emerged as a new mode of precise treatment. Nevertheless, taking highly effective phototheranostics into consideration, it is still a tremendous challenge to design multifunctional photothermal agents (PTAs) that combine the features of intensive near-infrared (NIR) absorption/emission, high photothermal conversion efficiency (PCE) and preferable tumor accumulation. Herein, seeking a convenient method to facilitate absorption red-shift, promote the accumulation of drugs in tumors and heighten the PCE appears to be particularly important for cancer theranostics. In this work, heavy-atom-free boron dipyrromethene (BODIPY) was assembled with F127 to fabricate ultra-small J-aggregated nanoparticles (named as BNPs). Compared to free BODIPY, BNPs exhibited 63 nm redshifted absorption, deep-tissue fluorescence imaging, enhanced cellular uptake, preferable tumor accumulation, elevated PCE, excellent photothermal stability and water dispersibility. In vivo experiments demonstrated that BNPs could behave as high-performance tumor fluorescent imaging probes and antitumor PTAs to conduct NIR imaging-guided PTT. This work offers a novel J-aggregated framework on developing robust diagnostic and therapeutic agents.
1. Introduction
Photothermal therapy (PTT), in which photothermal agents (PTAs) utilize light energy to generate local overheating within tumor tissues and ablate pathological cells has made tremendous strides in cancer treatment thanks to the merits of high therapeutic efficacy, little drug resistance, non-invasiveness and negligible systemic toxicity.1–10 As a key component, the inherent performance of PTAs is closely related to PTT outcomes: (1) PTAs that are triggered by long-wavelength light can achieve effective tumor imaging and treatment;11 (2) PTAs with small size can selectively accumulate into tumors endowed by enhanced permeation and retention (EPR) effects, thereby enhancing anticancer potency and reduce systemic toxicity to normal tissues;12,13 (3) PTAs with high photothermal conversion efficiency (PCE) can kill cancer cells efficiently.14 In this regard, exploration of a feasible strategy to construct PTAs that possess the above-mentioned properties appears particularly important. However, integrating all the advantages into one PTA is still a great challenge.
As a typical representative of organic dyes, boron-dipyrromethene (BODIPY) has emerged as a promising PTA due to outstanding physicochemical properties and excellent biocompatibility.15–23 More intriguingly, the optical performance of BODIPY chromophores can be modulated by diverse functionalization and aggregation of molecules.24–47 Moreover, the J-aggregates of BODIPY endow them with distinct advantages over those of monomers, which bring about considerably bathochromic-shifted absorption, low radiation transition, and high photostability. Several BODIPY-based PTAs have been reported to have J-aggregation behaviors. For example, Guo et al. reported that the J-aggregation of phenanthrene-[b]-fused BODIPY exhibited red-shifted absorption and elevated PCE.48 Yan et al. reported that J-stacked halogenated BODIPY-Gd(III) could enhance photothermal performance.49 Liu et al. reported that a BODIPY-Ir(III) complex formed J-aggregates via micellization to promote photodynamic therapy (PDT) and PTT.50 He et al. fabricated J-aggregates of BODIPY vesicles for PDT-synergized PTT.51 Our group also constructed J-aggregates of BODIPY to boost PTT.52 Nevertheless, like majority nanotherapeutic agents, nearly all J-aggregates are larger than 100 nm, which are not conducive to tumor accumulation. Furthermore, to date, no J-aggregated BODIPY that owns the features of NIR absorption and emission, ultra-small size, tumor specific accumulation and remarkable PTT enhancement has been developed yet.
In this work, the J-aggregation of BODIPY could be readily made and modulated by varying the solvent and temperature. In order to enhance the water solubility, biocompatibility and stability, BNPs were fabricated by encapsulating the J-aggregates into the hydrophobic core of Pluronic F127 (Scheme 1a). In contrast to BODIPY with the absorption at 687 nm, the absorption of BNPs red-shifted by 63 nm. In addition, the PCE of BNPs was promoted to 35.7%, which was 4.5 times to that of BODIPY. More significantly, BNPs had ultra-small size (16.6 ± 0.4 nm), which was beneficial for tumor accumulation because of the EPR effect. BNPs exhibited strong photocytotoxicity against HeLa and A549 cells. After intravenous injection, BNPs accumulated preferentially in the tumor tissues, the superiorities of NIR irradiation for deep tissue penetration and high PCE endowed BNPs with superior antitumor PTT efficacy (Scheme 1b).
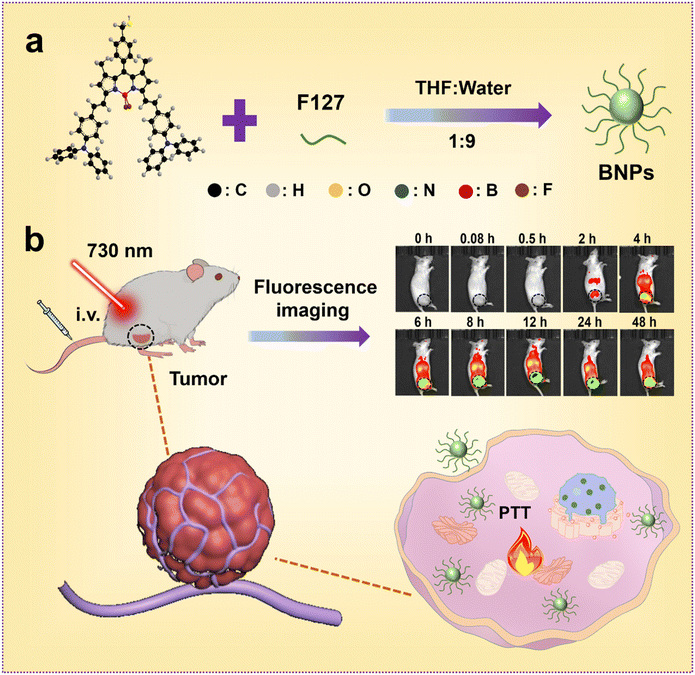 |
| Scheme 1 Schematic illustration of the (a) preparation and (b) applications of BNPs in fluorescence imaging and PTT. | |
2. Results and discussion
2.1. J-aggregation of BODIPY
BODIPY was synthesized according to our previous work.53 The UV-vis absorption spectrum of BODIPY shows that it absorbs significantly at 687 nm (Fig. 1a, black line). The intense emission was observed at 750 nm under excitation at 680 nm (Fig. 1a, red solid line). An obvious redshift of absorption will occur due to J-aggregation when BODIPY is dissolved into a mixture of organic solvents and water, which was verified by a series of experiments.54,55
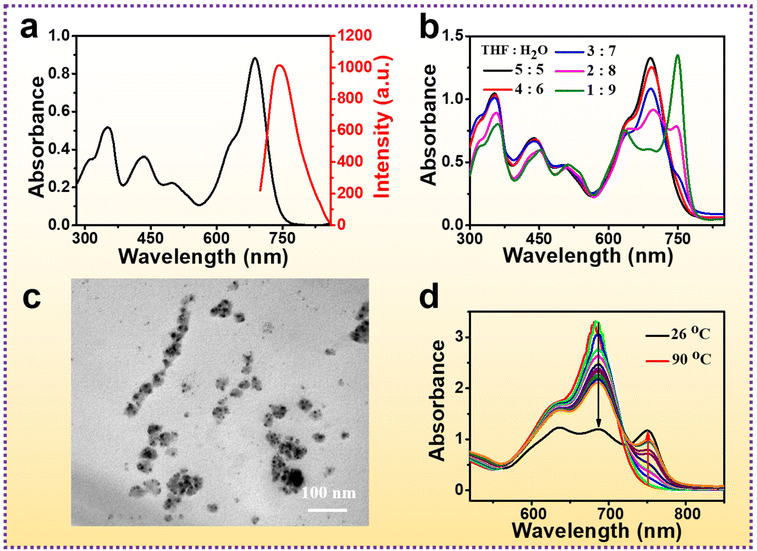 |
| Fig. 1 (a) UV-vis absorption and fluorescence spectra of BODIPY. (b) UV-vis absorption spectra of BODIPY in different volume ratios of THF/water. (c) TEM image of BODIPY J-aggregates (VTHF/VWater = 1 : 9). (d) Temperature-dependent absorption spectra of BODIPY (Vtotal = 10 mL, VTHF/Vwater = 2 : 8, 28.9 μM). | |
First, different volumes (VTHF = 5, 4, 3, 2 and 1 mL) of BODIPY solution were mixed with water (Vwater = 5, 6, 7, 8 and 9 mL) to form a THF-water solution, and the final concentration of BODIPY was 28.9 μM. The peak at 687 nm weakened with the increase in water content (Fig. 1b). When VTHF/Vwater = 2
:
8, a characteristic J-aggregate band appeared at 750 nm, along with the typical peak of monomers, indicating that monomers and J-aggregates coexisted in the solution. As VTHF/Vwater = 1
:
9, the characteristic absorption peak of monomers disappeared, while the peak at 750 nm brilliantly enhanced, verifying that monomers completely converted into J-aggregates in the mixed solvent. The maximum absorption peak of J-aggregates generated 63 nm of bathochromic shift in contrast to that of the monomer. In addition, the aggregates in the solution of VTHF/Vwater = 1
:
9 were measured by transmission electron microscopy (TEM), most of them were ∼10 nm spherical nanoparticles (NPs) (Fig. 1c), and some aggregates further assembled into bigger clusters (∼40 nm). The aggregation processes were studied using UV-vis absorption spectra (Fig. 1d). When the THF-water solution (Vtotal = 10 mL, VTHF/Vwater = 2
:
8, 28.9 μM) was heated to 90 °C, the maximum absorption peak appeared at 687 nm, indicating that BODIPY was in a monomeric state. During the process of temperature gradually falling from 90 °C to room temperature (26 °C), the peak at 687 nm slightly redshifted with the occurrence of the peak at 750 nm, demonstrating that BODIPY was in a J-aggregated state.
To further verify whether BODIPY forms J-aggregates, we calculated the electronic properties of BODIPY and excited state by density functional theory (DFT). The theoretical absorption spectrum (Fig. S1†) was basically consistent with the experimental one. The Multiwfn analysis of Fig. S1† indicated that the peak at 687 nm came from the contribution of S0 → S1.56 According to the frontier orbital theory, the contribution of S0 → S1 is approximated by the transition of the highest occupied molecular orbital (HOMO) to lowest unoccupied molecular orbital (LUMO). The calculated LUMO mainly locates in the BODIPY core, while the HOMO distributes throughout the molecule. LUMO and HOMO energies are −2.99 eV and −4.90 eV, and the energy gap is 1.91 eV (Fig. 2). Then, we simply utilized bimolecular BODIPY aggregates (J-Dimer) as models to simulate J-aggregates. In short, the configuration of J-Dimer was first obtained using AutoDock (Fig. S2†),57 and then its ground state energy was calculated using Gaussian 09 (method: B3LYP/6-311G (*)). The energy of LUMO and HOMO is −5.99 and −6.26 eV respectively, and the energy gap is 0.27 eV (Fig. 2). Compared with the monomer, the energy of J-Dimer decreased dramatically and the energy gap was obviously narrowed, which was conducive to the electronic transition from S0 to S1 and induced the red-shift of absorption. The theoretical calculation was agreed with the experimental results.
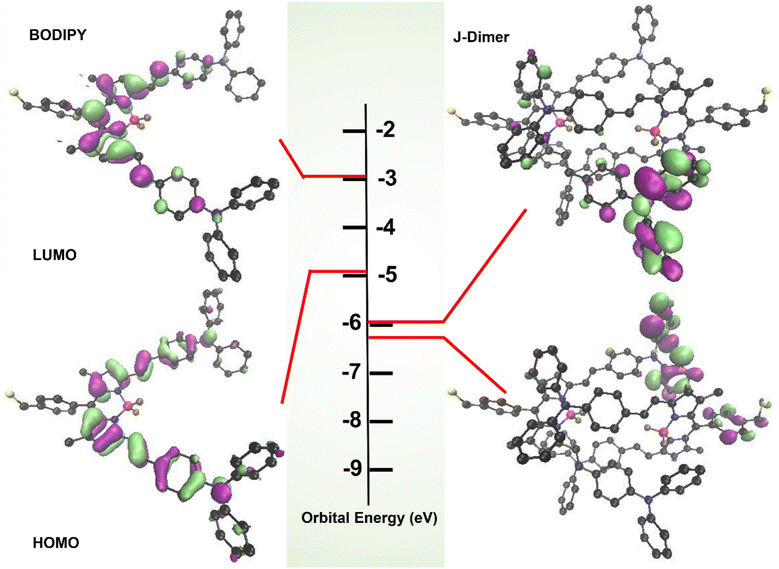 |
| Fig. 2 LUMO and HOMO of BODIPY and J-Dimer. | |
2.2 Preparation of BODIPY NPs
Since BODIPY is hydrophobic and only soluble in organic solvents, it seriously limits its biomedical application. In order to disperse BODIPY in an aqueous medium and improve its colloidal stability, Pluronic F-127 was utilized to encapsulate BODIPY and fabricate different concentrations of BODIPY NPs. The UV-vis absorption spectrum was measured by diluting the different concentrations of BODIPY NPs 40 times (Fig. 3a). As the concentration was 1.4 and 7.4 μM, their absorption bands were similar to those of BODIPY. When the concentration increased to 15.9 μM, a new shoulder peak appeared at 740 nm, indicating that J-aggregates began to form (Fig. 3a, pink line). When the concentration of BODIPY was between 39.5 and 153.0 μM, the absorption exhibited a significant bathochromic shift, and 750 nm of peak became the strongest one. The emission peaks of BODIPY NPs showed a blue-shift relative to free BODIPY, accompanied by obvious emission quenching with the increase in the concentration of BODIPY NPs (Fig. 3b). The bathochromically shifted absorption and considerably quenched fluorescence verified the generation of J-aggregates.
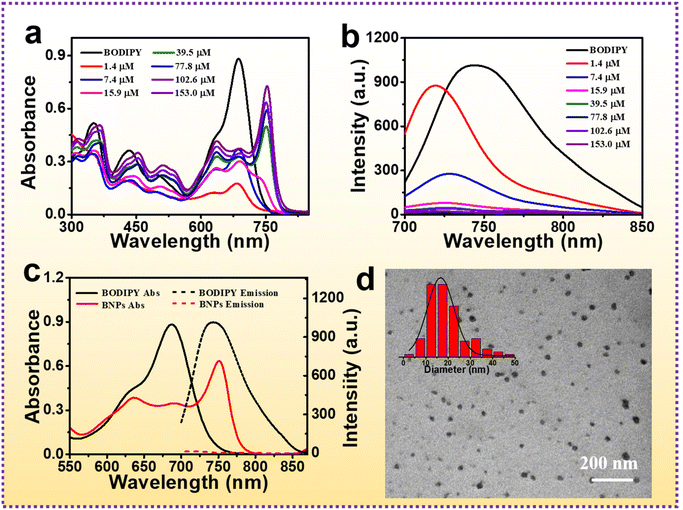 |
| Fig. 3 (a) UV-vis absorption and (b) fluorescence spectra of BODIPY and different concentrations of BODIPY NPs. (c) UV-vis absorption and fluorescence spectra of BNPs. (d) TEM image of BNPs. | |
Inspired by the NIR absorption ability of BODIPY NPs, BNPs were prepared for the follow-up experiments. A mixture of BODIPY (0.5 mg) and F-127 (10 mg) in THF (0.7 mL) was added dropwise to water (6.3 mL) and stirred for 24 h. The crude product was purified by centrifugation (5000 rpm, 5 min) to remove non-assembled BODIPY and then dialyzed for 24 h. The loading content of BODIPY was deduced to be 20.4%, according to the standard curve of BODIPY in DMF/water (v/v, 9
:
1) (Fig. S3†). The maximum absorption band of BNPs was centered at 750 nm, which generated a bathochromic-shift by 63 nm. The fluorescence was quenched due to the J-aggregation of BODIPY (Fig. 3c). The TEM images (Fig. 3d) definitely confirmed the pseudo spherical nanostructure of BNPs and the average size was ∼16.6 nm by measuring the diameter of 100 NPs using the Image J software to make sure the statistical significance. The ultra-small size is beneficial for tumor accumulation via the EPR effect. To investigate the stability of BNPs, the hydrodynamic diameter (HD) in water, PBS and 10% FBS were monitored by dynamic light scattering (DLS), which was almost constant after storage for seven days (water and PBS) or one day (10% FBS) with low PDI (Fig. S4†), demonstrating the colloidal stability of BNPs. To ensure the reliability of data, we repeated the TEM and DLS experiments three times, and similar results were obtained. The size of BNPs in water determined by TEM was smaller than that measured by DLS, because TEM characterized the samples in a dry environment and BNPs were largely shrunk in the solid state, whereas DLS was conducted in the aqueous medium and BNPs were in swollen states.
2.3 Photothermal effect of BNPs
The PTT efficacy of BNPs was investigated by changing the laser power density or the concentration of BNPs. At a constant concentration of BNPs (Fig. 4a), the temperature elevation was directly proportional to the laser power density, which rose by 43 °C in 5 min under irradiation of 0.8 W cm−2. Meanwhile, when 730 nm laser (0.6 W cm−2, 5 min) was used to irradiate different concentrations (0–28.9 μM) of BNPs, the temperature enhancement was positively correlated with the concentration (Fig. 4b). Thus, the photothermal triggered temperature rise was directly related to the laser power density and concentration, demonstrating the photothermal conversion process could be well controlled. Moreover, the PCE of BNPs was calculated to be 35.7%, according to the photothermal efficiency (Fig. 4c) and time constant (Fig. S5†). In stark contrast, the PCE of BODIPY was only 7.9%, which was much lower than that of BNPs. Obviously, J-aggregation could considerably enhance the PCE, making it more practicable for PTT. The anti-photobleaching ability of PTT agents under laser illumination is of great concern. The photostability of BNPs was evaluated by repeated irradiation/cooling cycles (Fig. 4d). The temperature increase of BNPs remained basically unchanged even after 5 irradiation/cooling cycles, undoubtedly proving the extraordinary photostability of BNPs. The photothermal images of BNPs and water upon 730 nm laser illumination are depicted in Fig. 4e. Within 5 min of irradiation, the temperature of BNPs increased by 32.1 °C, while the temperature of water only increased by 5 °C, indicating that BNPs have a high photothermal effect.
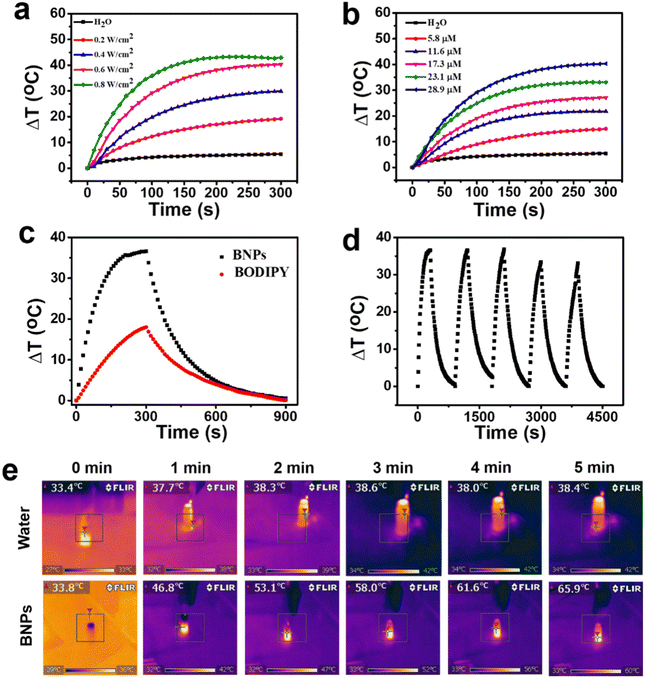 |
| Fig. 4 (a) Temperature changes of the BNP solution (23.1 μM) under laser irradiation (730 nm, 5 min) at different power densities. (b) Temperature changes of diverse concentrations of BNPs (0–28.9 μM) under laser irradiation (730 nm, 0.6 W cm−2, 5 min). (c) Photothermal efficiency of BNPs and BODIPY. (d) Temperature changes of the BNP aqueous solution (23.1 μM) in 5 heating and natural cooling cycles. (e) Infrared thermal images of water and BNP solutions. | |
2.4 Internalization and cytotoxicity of BNPs
Making use of the NIR absorption, high PCE, prominent photothermal stability as well as admirable fluorescence imaging ability, BNPs were employed as potential candidates for PTT applications. The internalization of BNPs was investigated toward HeLa cells. HeLa cells were incubated with BODIPY or BNP solutions at an equivalent concentration of 9.2 μM, and fluorescence was monitored using a confocal laser scanning microscope (CLSM). The CLSM images (Fig. 5a) depict that HeLa cells presented red fluorescence within 0.5 h and the fluorescence intensity enhanced by prolonging the incubation time (Fig. 5c). Thus, it was concluded that BNPs could be readily taken up by HeLa cells. By contrast, the cells treated with BODIPY monomers could only emit extremely faint fluorescence even after 4 h (Fig. 5b and c), demonstrating that free BODIPY was hard to be internalized. As BODIPY is very hydrophobic and BNPs are stable in physiological media, only a small fraction of BNPs might disassemble into BODIPY monomers in living cells, whose luminescence is enough for fluorescence imaging. The overwhelming majority of the internalized BNPs remain stable in the cells. We are confident that the main species involved in the photothermal effect are BNPs.
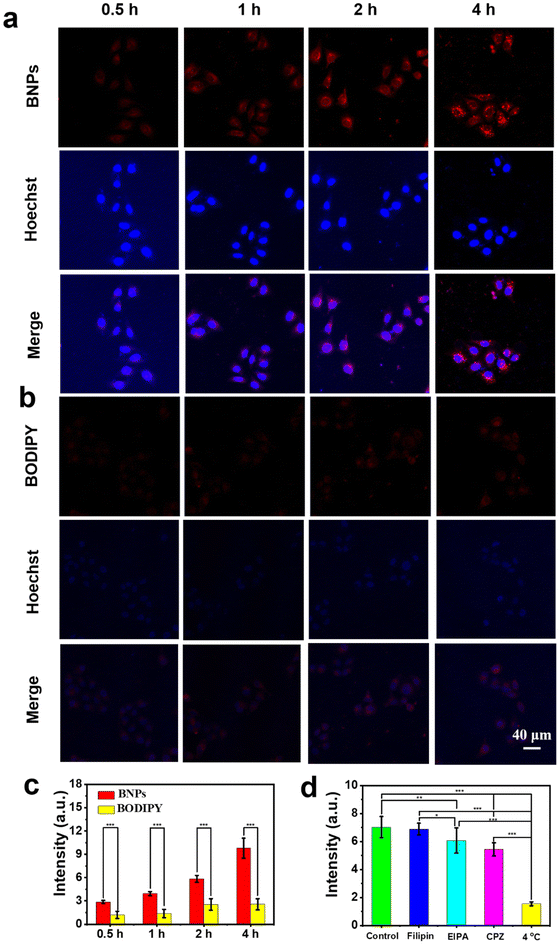 |
| Fig. 5 CLSM images of HeLa cells cultured with (a) BNPs or (b) BODIPY (CBODIPY = 9.2 μM) at different time points. (c) Quantitative analysis of (a) and (b). (d) Quantitative analysis of the different treatment groups in Fig. 6. The level of significance was set at a probability of *p < 0.05, **p < 0.01, and ***p < 0.001. | |
Due to the high efficiency of cellular uptake of BNPs, we investigated their endocytic pathway. First, we assessed the effect of energy supply on cellular uptake. HeLa cells were incubated with BNPs (9.2 μM) at 4 °C and 37 °C, respectively. As shown in Fig. 6 and 5d, after incubation for 4 h, despite violent red fluorescence presented in the 37 °C group, the emission in the 4 °C group was hardly visible, implying that the endocytosis of BNPs was mainly related to energy-dependent transport. We then used three endocytic inhibitors to validate the major endocytic pathways. Chlorpromazine (CPZ) interferes with clathrin-dependent pathways by blocking the assembly of clathrin-coated pits. 5-(N-Ethyl-N-isopropyl)-Amiloride (EIPA) can specifically inhibit the Na+/H+ pump on the cell membrane and inhibit the endocytosis of macrophages by preventing the signal transduction of Rac1 and Cdc4. Filipin is a cholesterol-binding agent that affects lipid raft-mediated endocytosis. As depicted in Fig. 6, the fluorescence in the Filipin group was similar to that of the 37 °C group. However, the emission in the EIPA and CPZ groups reduced obviously. The fluorescence intensity of the Filipin, EIPA, CPZ and 4 °C groups decreased by 2%, 14%, 23% and 78%, respectively (Fig. 5d). Thus, the internalization of BNPs was mainly energy-dependent and possibly accompanied by clathrin-mediated endocytosis and macropinocytosis.
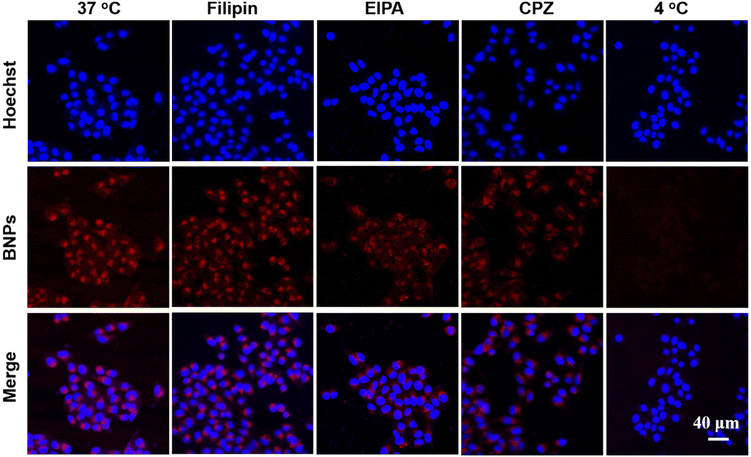 |
| Fig. 6 CLSM images of HeLa cells treated with different endocytosis inhibitors for 4 h. | |
The hemolytic effect of BNPs was evaluated toward red blood cells (RBCs). As shown in Fig. S6,† RBCs in the BNPs group hardly lysed, indicating that BNPs have good hemo-compatibility. The standard 3-(4,5-dimethylthiazol-2-yl)-2,5-diphenyltetrazolium ammonium bromide (MTT) assay was utilized to assess the biocompatibility and PTT effect of BNPs. Without laser irradiation, all of the cell viabilities were over 90%, implying that BNPs have good cytocompatibility (Fig. 7a). Upon irradiation (730 nm, 0.6 W cm−2, 5 min), the survival rate lowered significantly in a dosage-dependent manner and more than 88% of HeLa were killed as the concentration reached 11.6 μM. The IC50 value of BNPs was 4.5 μM. In addition, similar results were obtained when human lung cancer (A549) cells were treated with BNPs, and the IC50 was deduced to be 5.5 μM (Fig. S7a†). The results indicated that BNPs could significantly kill cancer cells upon NIR light exposure. By contrast, BNPs exhibited low phototoxicity toward normal cells (L929 cells, Fig. S7b†).
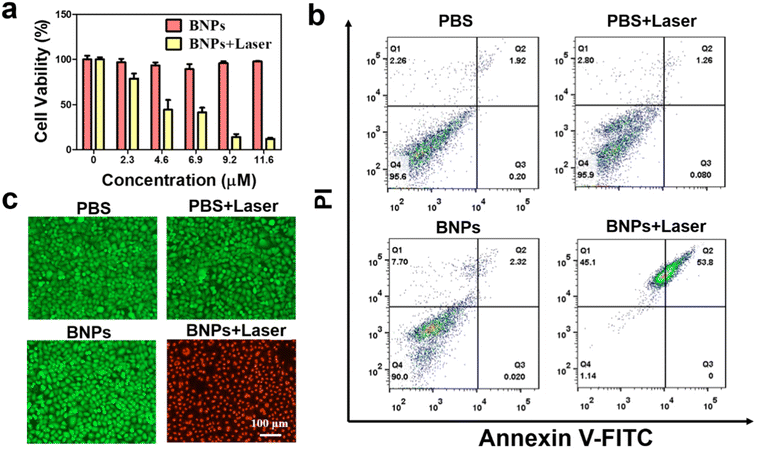 |
| Fig. 7 (a) Cytotoxicity of BNPs to HeLa cells without or under irradiation (730 nm, 0.6 W cm−2, 5 min). (b) Apoptosis of HeLa cells in the different treatment groups was analysed by flow cytometry (BNPs 11.6 μM). The four regions (Q1–Q4) represent the statuses of necrosis, late apoptosis, early apoptosis and live.58 (c) Fluorescence images of live-dead staining of HeLa cells from different treatment groups. | |
We further detected the necrosis and apoptosis of HeLa cells by flow cytometry (Fig. 7b). In the groups of PBS, PBS + Laser and BNPs, the percentage of necrosis and late apoptosis was only 4.2%, 4.1%, and 10.0%, respectively. By contrast, in the BNPs + Laser group, the rate of necrosis and late apoptosis was up to 98.9%, confirming the high PTT efficiency of BNPs. The phototoxicity of BNPs on cancer cells was also estimated by staining the live and dead cells with calcein-acetoxymethyl (calcein-AM) and propidium iodide (PI) (Fig. 7c). The green colour is the fluorescence emitted by calcein-AM, while the red colour is the fluorescence emitted by PI. In the PBS groups, cells irradiated with or without NIR laser displayed intense green fluorescence, suggesting that only laser irradiation would not cause cell apoptosis. In addition, no apoptosis occurred in the BNPs (11.6 μM) treatment group, again confirming that BNPs have good cytocompatibility. Upon irradiation, almost all of HeLa cells in the BNPs + Laser group died with luminous red fluorescence signal. These results indicate that BNPs have excellent biocompatibility and favorable PTT effects.
2.5 NIR Fluorescence imaging of BNPs in vivo
To research the tumor imaging ability of BNPs, U14 (Mouse cervical cancer cells) subcutaneous xenograft tumor were modeled in female Kunming mice. BNPs (3 mg kg−1) were administrated intravenously (i.v.) and the fluorescence images (Fig. 8a and b) of mice were monitored by an in vivo optical imaging system (PerkinElmer IVIS Lumina Series III). BNPs started to accumulate at the tumor in two hours after injection. The fluorescence signal of the tumour reached its peak at 12 h. Hence, 12 h after administration was the optimal exposure time for PTT in vivo. Even after 48 h, the tumour site still shows a bright fluorescence signal. To study the biodistribution of BNPs, the mice were executed at 48 h, the primary organs and tumors were imaged (Fig. 8c). The signal of liver was the brightest, followed by the tumor tissue (Fig. 8d and e), indicating that BNPs could selectively locate the tumor and were mainly metabolized by the liver. The above-mentioned results indicate that BNPs possess good pharmacokinetic performance in vivo.
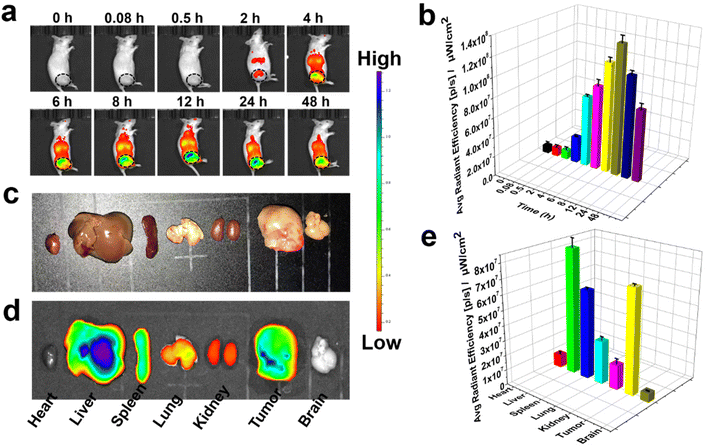 |
| Fig. 8 (a) Fluorescence images of the mouse (black dotted circles: tumor). (b) Quantitative fluorescence intensity of a. Photographs (c) and fluorescence images (d) of the major organs and tumor after BNPs treatment for 48 h. (e) Quantitative fluorescence intensity of d. | |
2.6 Anti-tumor activity of BNPs in vivo
To assess the anti-tumour potential of BNPs, tumour-bearing mice were grouped into PBS, PBS + Laser, BNPs, and BNPs + Laser. When the tumor volume reached 100–150 mm3, PBS or BNPs (3 mg kg−1) were intravenously injected into mice. After 12 hours, the tumor was exposed to laser (730 nm, 0.6 W cm−2). The temperature in the tumor increased quickly from 33.9 to 55.0 °C within 5 min (Fig. 9a), indicating that BNPs could preferentially accumulate into tumor areas and maintain the photothermal activity in vivo. By contrast, the temperature in the PBS group was only elevated by 1.8 °C, demonstrating that laser irradiation alone has ignorable heating effect. On day 16, the major organs and tumors were harvested. As shown in Fig. 9c–e, the tumors in the PBS, PBS + Laser and BNP groups showed rapid growth trends, and the tumor volume increased by 14.4, 16.3 and 15.7 times, suggesting that only laser irradiation or BNPs could not inhibit tumor proliferation. In sharp contrast, the tumor in the BNPs + Laser group was severely restrained and even some tumors were completely ablated, approving that BNPs could locate in the tumors and eradicate cancer cells upon irradiation. During the treatment, similar to the other three groups, the body weight in the BNPs + Laser group went up steadily (Fig. 9b), indicating that BNPs possess negligible systemic toxicity under current conditions. The tumor apoptosis was investigated by histological hematoxylin and eosin (H&E) staining assay. As depicted in Fig. 9f, no pathological changes were observed in the PBS, PBS + Laser and BNPs groups, manifesting that apoptosis and necrosis can be overlooked. In the BNPs + Laser group, a large number of necrotic areas appeared. As for the major organs (Fig. S8†), there was unconspicuous difference among the four groups. The whole blood panels were examined, and the result is shown in Fig. S9;† all the markers in the BNPs + Laser group were similar to those in the PBS group, indicating the acceptable biosafety of BNPs.
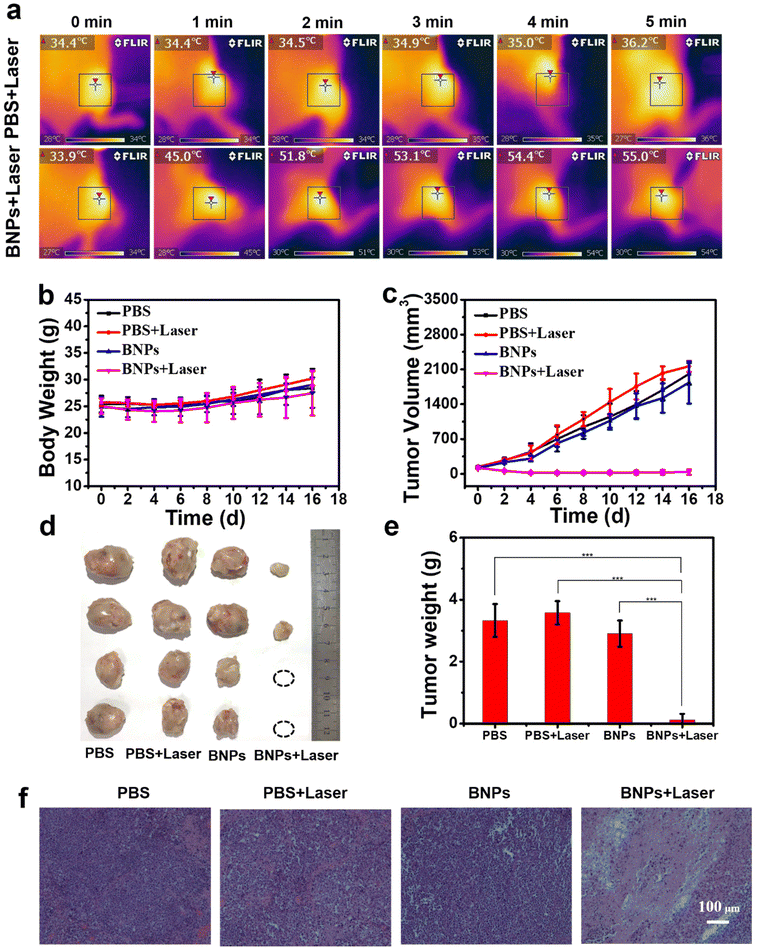 |
| Fig. 9 (a) Infrared photothermal images of tumor sites under laser irradiation (730 nm, 0.6 W cm−2, 5 min). (b) Changes in body weight of mice in the four groups (n = 4). (c) Tumor volume of each group (n = 4). (d) Pictures of tumors 16 days after various treatments; the black dotted circle represents the tumor was thoroughly ablated. (e) Mean tumour weights of the groups. Data are expressed as mean ± SD (n = 4). (f) H&E staining images of tumor slices. The level of significance was set at a probability of ***p < 0.001. | |
3. Conclusions
Stable J-aggregates were formed from the THF/water solution of BODIPY, whose absorption red-shifted from 687 to 750 nm. BNPs were constructed by the assembly of BODIPY with F127, which presented a spherical morphology with an ultra-small size of 16.6 ± 0.4 nm. Furthermore, BNPs still preserved the characteristics of J-aggregation with strong NIR absorption and significantly enhanced PCE. High photothermal activity and small size of BNPs resulted in the effective tumor accumulation and noninvasive NIR fluorescence imaging, which eventually led to the effective ablation of tumors with ignorable systemic toxicity. This work achieves a remarkable breakthrough in developing multifunctional nanomaterials for potent cancer therapy.
4. Experimental section
4.1 The theoretical calculation
We calculated the electronic properties of BODIPY by density functional theory (DFT). First, the B3LYP/6-311G (*) method of Gaussian 09 was used to calculate the energy gap of BODIPY, and the ground-state geometric optimization was carried out within the DFT framework. Then, the energy and vibrator intensity of the excited state absorbing electron transition were calculated, and the theoretical absorption spectrum of BODIPY was obtained by using the wave function analysis software Multiwfn.
4.2 Preparation of BODIPY NPs
Different masses of BODIPY (0.01, 0.05, 0.1, 0.25, 0.5, 0.75 and 1.0 mg) and 10.0 mg of F127 were dissolved in THF (0.7 mL), and the solution was dropped into water (6.3 mL) and stirred overnight to form different concentrations of BODIPY NPs.
4.3 The blocking assay
Before adding BNPs, HeLa cells were incubated with Filipin (5 μg mL−1) for 30 min, with EIPA (20 μg mL−1) for 1 h, with CPZ (10 μg mL−1) for 20 min, and the 4 °C group was placed at 4 °C for 30 min, respectively. The control group did not undergo any treatment. Afterwards, the cells were washed three times with PBS (pH = 7.4). Then, the medium was replaced with BNPs and diluted with a fresh culture medium to a final concentration of 9.2 μM. Thereafter, except for the 4 °C group, the incubation was required at 4 °C for 4 h, and the other groups were incubated at 37 °C for 4 h. The cellular uptake of NPs was examined by CLSM.
4.4
In vivo antitumor efficacy
To assess the anti-tumour potential of BNPs, tumour-bearing mice were grouped into PBS, PBS + Laser, BNPs, BNPs + Laser groups. When the tumor volume reached 100–150 mm3, PBS or BNPs (3 mg kg−1) were intravenously injected into mice. After 12 hours, the tumor was exposed to laser (730 nm, 0.6 W cm−2). The tumor apoptosis was investigated by a histological hematoxylin and eosin (H&E) staining assay.
Author contributions
Kunmei Liu, Min Zheng, Zhigang Xie conceived the work. Kunmei Liu, Hongxin Liu and Chaonan Li participated in the design and implementation of the experiment. Kunmei Liu carried out data processing and analysis. Kunmei Liu, Min Zheng, Zhigang Xie wrote and modified paper. All authors discussed and approved the manuscript.
Ethical statement
The animal studies were approved by the local institutional review board and performed according to the Guidelines of the Committee on Animal Use and Care of Chinese Academy of Sciences.
Conflicts of interest
The authors declare no competing financial interest.
Acknowledgements
The financial support from the National Natural Science Foundation of China (No. 51873023), the Science and Technology Development Plan of Changchun City (No. 21ZY38), and the Jilin Province Science and Technology Research Project (No. 20200201088JC).
References
- L. Cheng, K. Yang, Y. G. Li, J. H. Chen, C. Wang, M. W. Shao, S. T. Lee and Z. Liu, Angew. Chem., Int. Ed., 2011, 50, 7385–7390 CrossRef CAS.
- W. Zhang, Z. Y. Guo, D. Q. Huang, Z. M. Liu, X. Guo and H. Q. Zhong, Biomaterials, 2011, 32, 8555–8561 CrossRef CAS PubMed.
- K. Yang, L. L. Hu, X. X. Ma, S. Q. Ye, L. Cheng, X. Z. Shi, C. H. Li, Y. G. Li and Z. Liu, Adv. Mater., 2012, 24, 1868–1872 CrossRef CAS PubMed.
- K. Yang, J. M. Wan, S. Zhang, B. Tian, Y. J. Zhang and Z. Liu, Biomaterials, 2012, 33, 2206–2214 CrossRef CAS.
- C. Deng, Q. Zhang, J. K. Guo, X. F. Zhao and Z. Y. Zhong, Adv. Drug Delivery Rev., 2020, 160, 199–211 CrossRef CAS PubMed.
- Y. Q. Zhu, Y. Jiang, F. H. Meng, C. Deng, R. Cheng, J. Zhang, J. Feijen and Z. Y. Zhong, J. Controlled Release, 2018, 278, 1–8 CrossRef CAS PubMed.
- Y. C. Zhang, H. Q. Tao, Q. G. Li, W. L. Sheng, Y. J. Xu, E. H. Hao, M. W. Chen, Z. Liu and L. Z. Feng, J. Controlled Release, 2020, 326, 256–264 CrossRef CAS PubMed.
- D. Jana, D. D. Wang, A. K. Bindra, Y. Guo, J. W. Liu and Y. L. Zhao, ACS Nano, 2021, 15, 7774–7782 CrossRef CAS.
- Z. Y. Wang, Z. Y. Li, Z. L. Sun, S. R. Wang, Z. S. Ali, S. H. Zhu, S. Liu, Q. S. Ren, F. G. Sheng, B. D. Wang and Y. L. Hou, Sci. Adv., 2020, 6, eabc8733 CrossRef CAS PubMed.
- J. J. Wang, X. Y. Wang, S. Y. Lua, J. Hua, W. Zhang, L. E. Xua, D. C. Gua, W. T. Yang, W. Tang, F. J. Liu, Y. Cao and H. Liu, Biomaterials, 2019, 223, 119465 CrossRef CAS PubMed.
- S. Chen, X. Wang, Y. J. Qiao, B. X. Gao, M. Zheng and Z. G. Xie, Chem. Eng. J., 2021, 419, 129552 CrossRef CAS.
- T. T. Sun, H. M. Liu, N. Jiang, Q. H. Wu, C. N. Li, R. Xia, B. X. Gao and Z. G. Xie, Adv. Funct. Mater., 2021, 31, 2103714 CrossRef CAS.
- M. Zheng, Y. Li, S. Liu, W. Q. Wang, Z. G. Xie and X. B. Jing, ACS Appl. Mater. Interfaces, 2016, 8, 23533–23541 CrossRef CAS.
- Z. Y. Jiang, C. L. Zhang, X. Q. Wang, M. Yan, Z. X. Ling, Y. C. Chen and Z. P. Liu, Angew. Chem., Int. Ed., 2021, 60, 22376–22384 CrossRef CAS.
- C. Ma, T. Zhang and Z. G. Xie, J. Mater. Chem. B, 2021, 9, 7318–7327 RSC.
- M. W. Yang, J. L. Fan, J. J. Du and X. J. Peng, Chem. Sci., 2020, 11, 5127–5141 RSC.
- Y. Zou, S. Long, T. Xiong, X. Z. Zhao, W. Sun, J. J. Du, J. L. Fan and X. J. Peng, ACS Cent. Sci., 2021, 7, 327–334 CrossRef CAS.
- M. H. Su, Q. J. Han, X. S. Yan, Y. N. Liu, P. Luo, W. H. Zhai, Q. Z. Zhang, L. Y. Li and C. H. Li, ACS Nano, 2021, 15, 5032–5042 CrossRef CAS PubMed.
- D. M. Xi, M. Xiao, J. F. Cao, L. Y. Zhao, N. Xu, S. Long, J. L. Fan, K. Shao, W. Sun, X. H. Yan and X. J. Peng, Adv. Mater., 2020, 32, 1907855 CrossRef CAS PubMed.
- Y. Su, N. Wang, B. J. Liu, Y. Y. Du, R. N. Li, Y. Meng, Y. Q. Feng, Z. Q. Shan and S. X. Meng, Dyes Pigm., 2020, 177, 108262 CrossRef CAS.
- N. Liu, M. Zhu, N. Niu, J. Ren, N. Yang and C. Yu, ACS Appl. Mater. Interfaces, 2020, 12, 41071–41078 CrossRef.
- J. Chen, Y. X. Cui, K. W. Song, T. Q. Liu, L. Y. Zhou, B. Q. Bao, R. B. Wang and L. H. Wang, Biomater. Sci., 2021, 9, 2115–2123 RSC.
- Y. Kage, H. Karasaki, S. Mori, H. Furuta and S. Shimizu, ChemPlusChem, 2019, 84, 1648–1652 CrossRef CAS PubMed.
- M. Hecht, T. Schlossarek, M. Stolte, M. Lehmann and F. Würthner, Angew. Chem., Int. Ed., 2019, 58, 12979–12983 CrossRef CAS.
- C. X. Sun, B. H. Li, M. Y. Zhao, S. F. Wang, Z. H. Lei, L. F. Lu, H. X. Zhang, L. S. Feng, C. R. Dou, D. R. Yin, H. X. Xu, Y. S. Cheng and F. Zhang, J. Am. Chem. Soc., 2019, 141, 19221–19225 CrossRef CAS.
- M. Más-Montoya and R. A. J. Janssen, Adv. Funct. Mater., 2017, 27, 1605779 CrossRef.
- G. Chen, H. Sasabe, Y. Sasaki, H. Katagiri, X. F. Wang, T. Sano, Z. Hong, Y. Yang and J. J. Kido, Chem. Mater., 2014, 26, 1356–1364 CrossRef CAS.
- K. Cai, J. J. Xie, D. Zhang, W. J. Shi, Q. F. Yan and D. H. Zhao, J. Am. Chem. Soc., 2018, 140, 5764–5773 CrossRef CAS PubMed.
- N. Keller, M. Calik, D. Sharapa, H. R. Soni, P. M. Zehetmaier, S. Rager, F. Auras, A. C. Jakowetz, A. Görling, T. Clark and T. Bein, J. Am. Chem. Soc., 2018, 140, 16544–16552 CrossRef CAS PubMed.
- M. H. Su, S. X. Li, H. Zhang, J. Q. Zhang, H. L. Chen and C. H. Li, J. Am. Chem. Soc., 2019, 141, 402–413 CrossRef CAS PubMed.
- K. Yuan, X. Wang, S. K. Mellerup, I. Kozin and S. N. Wang, J. Org. Chem., 2017, 82, 13481–13487 CrossRef CAS PubMed.
- D. Kand, P. Liu, M. X. Navarro, L. J. Fischer, L. Rousso-Noori, D. Friedmann-Morvinski, A. H. Winter, E. W. Miller and R. Weinstain, J. Am. Chem. Soc., 2020, 142, 4970–4974 CrossRef CAS.
- M. Poddar and R. Misra, Coord. Chem. Rev., 2020, 421, 213462 CrossRef CAS.
- C. S. Wijesooriya, J. A. Peterson, P. Shrestha, E. J. Gehrmann, A. H. Winter and E. A. Smith, Angew. Chem., Int. Ed., 2018, 57, 12685–12689 CrossRef CAS PubMed.
- M. L. Agazzi, M. B. Ballatore, A. M. Durantini, E. N. Durantini and A. C. Tomé, J. Photochem. Photobiol., C, 2019, 40, 21–48 CrossRef CAS.
- J. H. Zou, Z. H. Yin, K. K. Ding, Q. Y. Tang, J. W. Li, W. L. Si, J. J. Shao, Q. Zhang, W. Huang and X. C. Dong, ACS Appl. Mater. Interfaces, 2017, 9, 32475–32481 CrossRef CAS.
- W. H. Zhai, Y. K. Zhang, M. Liu, H. Zhang, J. Q. Zhang and C. H. Li, Angew. Chem., Int. Ed., 2019, 58, 16601–16609 CrossRef CAS PubMed.
- J. H. Olivier, J. Widmaier and R. Ziessel, Chem. – Eur. J., 2011, 17, 11709–11714 CrossRef CAS PubMed.
- Q. D. Zheng, G. X. Xu and P. N. Prasad, Chem. – Eur. J., 2008, 14, 5812–5819 CrossRef CAS.
- H. Wu, X. Guo, C. J. Yu, W. Y. Wong, E. Hao and L. J. Jiao, Dyes Pigm., 2020, 176, 108209 CrossRef CAS.
- Y. Su, S. Y. Lu, P. L. Gao, M. Zheng and Z. G. Xie, Mater. Chem. Front., 2019, 3, 1747–1753 RSC.
- M. L. Li, R. S. Tian, J. L. Fan, J. J. Du, S. R. Long and X. J. Peng, Dyes Pigm., 2017, 147, 99–105 CrossRef CAS.
- Z. Shahroosvand, N. R. Yeganeh, S. Haddadian, J. Farzaneh and M. S. Ardestani, Appl. Nanosci., 2020, 10, 1441–1452 CrossRef CAS.
- N. Song, L. Fu, Y. Liu, Y. Y. Li, L. Chen, X. Y. Wang, S. Liu and Z. G. Xie, Dyes Pigm., 2019, 162, 295–302 CrossRef CAS.
- C. N. Li, W. H. Lin, S. Liu, T. T. Sun and Z. G. Xie, Mater. Chem. Front., 2021, 5, 284–292 RSC.
- S. Chen, T. T. Sun, M. Zheng and Z. G. Xie, Adv. Funct. Mater., 2020, 30, 2004680 CrossRef CAS.
- T. Zhang, C. Ma, T. T. Sun and Z. G. Xie, Coord. Chem. Rev., 2019, 390, 76–85 CrossRef CAS.
- X. Guo, M. Li, H. Wu, W. L. Sheng, Y. M. Feng, C. J. Yu, L. J. Jiao and E. H. Hao, Chem. Commun., 2020, 56, 14709–14712 RSC.
- X. S. Yan, M. H. Su, Y. N. Liu, Y. K. Zhang, H. Zhang and C. H. Li, Adv. Funct. Mater., 2021, 31, 2008406 CrossRef CAS.
- B. Q. Liu, J. Jiao, W. Xu, M. Y. Zhang, P. Cui, Z. Q. Guo, Y. B. Deng, H. B. Chen and W. F. Sun, Adv. Mater., 2021, 33, 2100795 CrossRef CAS.
- H. He, S. S. Ji, Y. He, A. J. Zhu, Y. L. Zou, Y. B. Deng, H. T. Ke, H. Yang, Y. L. Zhao, Z. Q. Guo and H. B. Chen, Adv. Mater., 2017, 29, 1606690 CrossRef PubMed.
- J. X. Xia, Z. S. Li, Z. G. Xie and M. Zheng, J. Colloid Interface Sci., 2021, 599, 476–483 CrossRef CAS.
- C. N. Li, W. H. Lin, S. Liu, W. Zhang and Z. G. Xie, J. Mater. Chem. B, 2019, 7, 4655–4660 RSC.
- H. Y. Wang, J. J. Chang, M. W. Shi, W. Pan, N. Li and B. Tang, Angew. Chem., Int. Ed., 2019, 58, 1057–1061 CrossRef CAS PubMed.
- Z. J. Chen, Y. Liu, W. Wagner, V. Stepanenko, X. K. Ren, S. Ogi and F. Würthner, Angew. Chem., Int. Ed., 2017, 56, 5729–5733 CrossRef CAS.
- T. Lu and F. W. Chen, J. Comput. Chem., 2012, 33, 580–592 CrossRef CAS PubMed.
- G. M. Morris, D. S. Goodsell, R. S. Halliday, R. Huey, W. E. Hart, R. K. Belew and A. J. Olson, J. Comput. Chem., 1998, 19, 1639–1662 CrossRef CAS.
- G. Kumar, H. Degheidy, B. Casey and P. Goering, Food Chem. Toxicol., 2015, 85, 45–51 CrossRef CAS PubMed.
|
This journal is © The Royal Society of Chemistry 2023 |
Click here to see how this site uses Cookies. View our privacy policy here.