DOI:
10.1039/D2YA00161F
(Communication)
Energy Adv., 2022,
1, 671-676
PolarClean & dimethyl isosorbide: green matches in formulating cathode slurry†
Received
28th June 2022
, Accepted 1st September 2022
First published on 1st September 2022
Abstract
In this study, two green organic solvents are reported in LiNi1/3Co1/3Mn1/3O2 (NMC111)-based slurry preparation and subsequent cathode fabrication for Li ion batteries. NMC111, conductive carbon and poly(vinylidene fluoride) binder composite slurries prepared with methyl-5-(dimethylamino)-2-methyl-5-oxopentanoate (PolarClean) and dimethyl isosorbide (DMI) exhibit mechanically stable, crack-free uniform coating structures. Both slurries showed similar shear-thinning viscosity behavior that suggests similar processibility during electrode casting and coating. When used as the cathode in Li/NMC111 half cells, the electrode slurries prepared with PolarClean show promising electrochemical performance metrics with an average specific charge capacity of 155 ± 1 mA h g−1 at C/10 over 100 cycles, comparable to the films (152 ± 3 mA h g−1 at C/10) prepared with traditional N-methyl pyrrolidone (NMP) solvent. The use of PolarClean points to a potential route to replace toxic NMP in cathode fabrication without altering the manufacturing process. However, electrodes prepared with DMI demonstrate inferior electrochemical performance with an average charge capacity of 120 mA h g−1. Nonetheless, DMI may still offer some promising features and warrants further detailed investigation in terms of compatible electrolyte, tailoring the slurry preparation, and casting conditions.
Introduction
State-of-the-art lithium ion battery (LIB) manufacturing has recently focused on electrode fabrication procedures that minimize environmental hazards. Current electrode fabrication technique uses a slurry casting process where the polyvinylidene fluoride (PVDF) binder is dissolved in organic solvent and dispersed with the active material to create an interconnected, percolated conductive network. PVDF has only been shown to dissolve in a few organic solvents, such as N-methyl pyrrolidone (NMP), N,N-dimethyl formamide (DMF), and N,N-dimethylacetamide (DMAC), that disrupt the strong interchain interactions in crystalline PVDF.1,2 Despite successful solubilization of PVDF, these solvents exhibit slow biodegradability and high carcinogenic, mutagenic and reprotoxic (CMR) risks to the human health.3–5 NMP is declared as a priority substance in USA under the Toxic Substance Control Act (TSCA) for having “unreasonable risk” and requires immediate regulatory action.4,6 Water was investigated as a slurry solvent with the goal of establishing a sustainable cathode manufacturing process with reduced cost.7 However, water is restricted to water soluble binders only and these electrodes exhibit poor wettability, film cracking, poor stability with Ni-based active materials, corrosion of aluminum current collectors, and poor battery performance,8 so it has not been adopted as a successful casting solvent.9 In addition, water soluble polymer binders (e.g., carboxymethyl cellulose, polyacrylic acid) often suffer from agglomeration due to their long macromolecular chains, which hinders the homogeneous dispersion of active material in the slurry.10 Thus, sustainable fabrication of PVDF slurry-based electrode involving environmentally friendly organic solvent is urgently needed to mitigate risks to both the environment and human health. Recently, dimethyl sulfoxide (DMSO), γ-valerolactone, and cyrene were reported as greener alternatives for NMP that enable mass slurry production in a sustainable fashion.9,11,12 However, all these solvents require 60–90 °C for dissolving and processing PVDF binder during electrode casting, whereas current NMP solvent can be used at room temperature (RT). Slurry casting ideally occurs at RT, as high temperature might cause solvent decomposition and interfere with electrode performance.13 Additionally, DMSO is reported to have detrimental effects on red blood, vascular endothelial and skin fibroblast cells at low to moderate concentrations.14 Here, we report the use of two green organic solvents, dimethyl isosorbide (DMI) and methyl-5-(dimethylamino)-2-methyl-5-oxopentanoate (PolarClean), in fabricating cathode slurries.
DMI, a sugar-based dipolar aprotic solvent derived from D-sorbitol is commercially used in pharmaceutical additives and personal care products due to its non-toxic nature.3,15 DMI has been recently used as an alternative to NMP during the fabrication of a PVDF-based ultrafiltration membrane16 and delamination process of end-of-life LIB cathodes17 due to the excellent solubility of PVDF in DMI. Likewise, PolarClean is a nitrogen-containing dipolar aprotic solvent that is the byproduct of adiponitrile manufacturing. PolarClean has been recently added as a new member of the green solvent family3 and used to replace the solvent NMP used to make PVDF-based hollow fiber membranes.18 In addition, PolarClean is biodegradable (97% degrades in 3 weeks) with no detectable environment and health hazards in doses as high as 1000 mg (kg day)−1,3,19–22 whereas NMP exposure is restricted to 4.8 mg (kg day)−1 by dermal exposure in the European Union.11,23 Similarly, DMI was pronounced to “not likely be a concern”24 with respect to human health hazard properties (CMR) with a dose of 300 mg (kg day)−1, though it is not readily biodegradable like PolarClean.24,25 These findings inspired us to explore the use of these solvents to fabricate PVDF based composite cathode slurries for LIBs, which has not been investigated yet. Herein, we compare the rheological properties, coating qualities, and electrochemical stabilities of composite cathodes made with NMP, PolarClean, and DMI.
Results and discussion
We consider two potential greener alternatives to NMP solvent in composite cathode slurry preparation for LIBs: DMI and PolarClean (Fig. 1c and d; the structure of PVDF and NMP are shown in Fig. 1a and b, respectively). NMP is a nonaromatic molecule with a five membered lactam structure. PolarClean is an acyclic system which contains one aliphatic ester and aliphatic tertiary amide linkage, similar to DMAC, whereas DMI is a chiral, V-shaped ether with two cis-connected tetrahydrofuran rings. Although structurally different, all of these solvents have similar densities (1.04, 1.16 and 1.03 g mL−1 for PolarClean, DMI, and NMP, respectively) and comparable polarity (solvent properties are listed in Table 1). PolarClean has a dielectric constant ε = 28.3 ± 3.5 and dipole moment μ = 4.4 D close to that of NMP (ε = 32 and μ = 4.1 D), suggesting that both solvents may exhibit similar polarities and charge stabilities. The μ values for both solvents are higher than PVDF (1.3–2.1 D), that may facilitate the polymer interaction with the solvent molecules and favor the formation of all-trans planar zigzag conformation or thermodynamically stable β phase crystalline structure pre-dominantly, which demonstrates electroactivities including high piezo-, pyro-, and ferroelectric properties.26
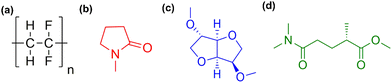 |
| Fig. 1 Chemical structure of PVDF binder (a), NMP solvent (b), DMI solvent (c), and PolarClean solvent (d). | |
Table 1 Physical properties of the proposed solvents13,17,21,27
Solvent |
CAS no. |
Molecular weight (g mol−1) |
Boiling point (°C) |
Flash point (°C) |
Appearance |
Density (g mL−1) |
Dielectric constant (ε) |
Dipole moment (D) |
NMP |
872-50-4 |
99.13 |
202 |
86–95 |
Clear, colorless liquid |
1.03 |
32 |
4.09 |
DMI |
5306-85-4 |
174.19 |
235 |
108 |
Clear, colorless liquid |
1.16 |
— |
— |
PolarClean |
1174627-68-9 |
187.24 |
280 |
144–146 |
Clear, slightly yellow liquid |
1.04 |
28.3 ± 3.5 |
4.41 |
Predictive principle for PVDF solubilization in chosen solvents
In slurry preparation, one of the crucial prerequisites is that the PVDF must dissolve in the solvent, so solubility parameters for the shortlisted candidates are investigated next (solubility parameters are listed in Table 2, as consistently found in other literature3,11,16). Interactions between individual organic solvents (Fig. 1b–d) and the PVDF binder solute (Fig. 1a) are evaluated with two thermodynamic parameters: the Hansen solubility parameter (HSP) and the Hildebrand parameter (δT) (Table 2). HSP considers the distance between the solvent and the polymer with respect to dispersion (δd), polar/dipole–dipole (δP), and hydrogen bonding (δh) interactions in the Hansen dimension space with the assumption that enthalpic contributions dominate solubilization.16,28,29Table 2 shows that PVDF, NMP, DMI, and PolarClean all exhibit similar HSP values for the dispersion term (17.2, 18.0, 17.6, and 15.8–16.6 MPa1/2, respectively), the polar/dipole–dipole term (12.5, 12.3, 7.1, and 10.7–13.4 MPa1/2 respectively), and the hydrogen bonding term (9.2, 7.2, 7.5, and 9.2–9.5 MPa1/2, respectively) so all three solvents are expected to show similar energy penalties for dispersing PVDF. Analyzing the HSP values more closely, PolarClean and DMI are found to have lower dispersion and polar terms respectively, whereas NMP exhibits the least hydrogen bonding term with respect to PVDF. Of the three potential solvents for PVDF, the one that deviates the most based on the HSP value is DMI, so we may predict a priori that DMI may not solubilize PVDF as well as NMP or PolarClean, which is consistent with our experimental observations (see below).
Polymer/solvent |
Hildebrand (δT) MPa1/2 |
HSP (δd) MPa1/2 |
HSP (δp) MPa1/2 |
HSP (δh) MPa1/2 |
R
0 MPa1/2 |
R
a MPa1/2 |
RED |
— Data not found. R0, an experimentally obtained value, specific for each polymer. R0 for PVDF is obtained from ref. 16 and 29. RED values are calculated using eqn (3). Ra value for PVDF-PolarClean was estimated using the HSP values for PolarClean as δd = 15.8 MPa1/2, δp = 10.7 MPa1/2 and δh = 9.2 MPa1/2. Ra values are obtained from ref. 16. |
PVDF |
24.2 |
17.2 |
12.5 |
9.2 |
9.6 |
— |
— |
NMP |
23.0 |
18.0 |
12.3 |
7.2 |
— |
2.6 |
0.3 |
DMI |
20.4 |
17.6 |
7.1 |
7.5 |
— |
5.7 |
0.6 |
Polarclean |
21.2 |
15.8–16.6 |
10.7–13.4 |
9.2–9.5 |
— |
3.3a |
0.3 |
Hildebrand solubility parameters (δT) were estimated based on the cohesive energies of HSPs following eqn (1) (Table 2). Similar δT values for PVDF (24.2 MPa1/2), NMP (23 MPa1/2), DMI (20.4 MPa1/2) and PolarClean (21.2 MPa1/2) agree well with the prediction that all three potential solvents should be able to solubilize PVDF.16
| 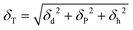 | (1) |
The affinity and relative energy difference (RED) parameters for the PVDF in the proposed solvents were estimated using eqn (2) and (3), where Ra is the difference between the HSP for the PVDF (A) and corresponding solvent (B), and R0 is the radius of interaction in the Hansen space.11,28 A RED value <1 indicates that the solvent will dissolve the polymer and >1 indicates poor solubility.16 In the present case, the RED parameters for PVDF in DMI and PolarClean both were found to have <116 (the RED parameters for DMI and PolarClean are 0.6 and 0.3, respectively), similar to NMP (0.3), further suggesting that they are both able to dissolve PVDF.
|  | (2) |
|  | (3) |
PVDF binder dissolution
In order to validate the above-mentioned predictive principles, PVDF dissolution test9,12,21 was carried out in NMP, DMI, and PolarClean at three different concentrations of 1, 3 and 10 wt/v%, described in Fig. S3–S6, ESI.† To do so, 0.01, 0.03 and 0.1 g of PVDF were added to 1 mL of each solvent to prepare 1, 3 and 10 wt/v% binder solutions. The 1 and 3 wt% samples produced clear solutions in NMP at RT, whereas the 10 wt% solution showed limited solubility with traces of polymer residue (Fig. S3, ESI†). Upon heating to 120 °C overnight, the solution gradually cleared. However, upon cooling, the 10 wt% solution turned into a semi-solid gel.
At low concentration (1 wt%) in PolarClean, PVDF forms a translucent solution with no sign of polymer residue at RT, (Fig. S4, ESI†) similar to NMP. However, when the PVDF concentration is raised to 3 wt%, the solution became cloudy with traces of PVDF sediments. It converts to a translucent solution after heating at 60 °C and became fully clear solution with no traces of PVDF residue, only when heated at elevated temperature of 120 °C. Unlike NMP, the higher concentrations of PVDF (10 wt%) in PolarClean leads into forming a white suspension which turns into a gel, while heated at 120 °C. We conclude that (i) PVDF is soluble in PolarClean at RT with a low concentration (e.g., 1 wt%) and (ii) shows temperature-dependent solubility profile (e.g., solid suspension to semi-solid gel) for higher concentrations (e.g., 10 wt%).
Likewise, the 1 wt% PVDF solution in DMI forms a viscous solution, and the 3 wt% has a cloudy appearance with traces of precipitating PVDF (Fig. S5, ESI†). Upon increasing temperature to 60 °C, the 3 wt% polymer solution becomes translucent. As expected, the 10 wt% polymer shows limited solubility at RT but forms a semi-solid gel while heated at 120 °C. Therefore, we conclude that DMI may dissolve small amounts of PVDF at RT, similar to NMP and PolarClean, however higher temperatures are required to prepare a transparent solution with higher concentrations of PVDF. Once dissolved, PVDF remains solubilized in PolarClean and DMI at RT after 1 month of storage without any precipitation (Fig. S6, ESI†). All these results agree with the solubility predictions previously discussed.
Slurry preparation and rheology
After confirming these solubilities, slurries were prepared (Fig. 2a, described in ESI†) by mixing NMC111, conductive carbon and PVDF binder dissolved in NMP, DMI and PolarClean at RT, respectively. The three most important features of a slurry that control the electrode morphology are: (i) the active material must not agglomerate or sediment in the colloidal suspension, (ii) the active material must not react with the processing solvent and current collector substrate, and the (iii) ease of processibility during coating.31–33 No particle sedimentation, gravitational settling, aggregation of active materials or sign of a byproduct gas evolution was noted during coating or drying of the slurries prepared in NMP, DMI and PolarClean (Fig. S1 and S7, ESI†). Conversely, severe agglomeration, bubble formation, and cracking were reported in water-based NMC slurries.8,34 The effectiveness of the homogenized slurry is typically characterized by its flow behavior and thus, viscosities for the corresponding slurries were examined by rheology. Ideally, the slurry should have high viscosity at low shear rate and low viscosity at high shear rate to stabilize the colloidal suspension and to form a uniform and defect-free coating.31–33 Initial viscosities (at a low shear rate of 0.1 to 1 1 s−1) were found to be 10.2, 21.5 and 1.5 Pa s, for slurries prepared in NMP, DMI and PolarClean, respectively (Fig. 2b). Higher viscosity at the low shear rate was found for the DMI processed slurry, which suggests more favorable and appropriate settling behavior of NMC in DMI, compared to NMP and PolarClean. However, all three slurries showed similar shear thinning viscosity behavior (i.e., decreasing viscosity with increasing shear rare) which is advantageous for fabricating a uniform coating (Fig. 2b).33 It is noteworthy that viscosities for all slurries reduced in the shear rate range of 10–100 (Fig. S8, ESI†), relevant for a doctor blade coating process.12 To summarize, all three slurries are comprised of well-dispersed particles and can facilitate smooth casting and coating on the metal current collector with similar processing conditions.
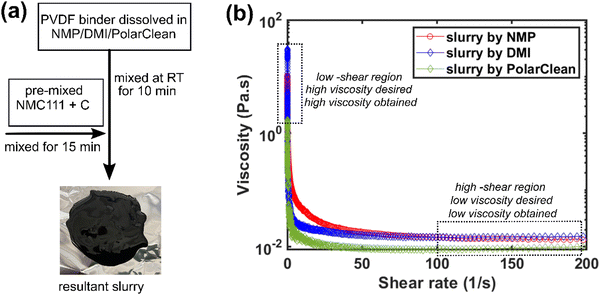 |
| Fig. 2 Schematic diagram of a slurry preparation protocol with sequential addition (a). Rheology plot (viscosity vs. shear rate) (b) shows desirable shear-thinning behavior (i.e., viscosity decreases with increasing shear rate) of NMC111 slurries prepared using NMP, DMI and PolarClean. | |
Morphology and stability of coated electrodes
All three slurries were cast onto Al substrates and dried to make the composite electrodes (Fig. 3a–c and Fig. S7, ESI†). It is imperative to ensure that the coated composite films are homogeneous and crack-free, thus scanning electron microscopy (SEM) analysis was performed next. No cracks were detected in the dried electrodes by top-view and cross-sectional SEM analysis (Fig. 3d–i and Fig. S9, S11, ESI†). Higher magnification SEM images of the electrodes made by DMI and PolarClean showed a high degree of adsorption of conductive carbon and PVDF binder on the surfaces of NMC111 (Fig. 3d–f and Fig. S9, S10, ESI†). This observation not only demonstrates the excellent dispersion of active material, conductive carbon and binder in the slurry, but provides mechanical strength to the coating. No delamination was noted for these electrodes after storing in LP30 electrolyte for one month (Fig. 3j–o) which further indicates the mechanical toughness, good solubility, and adhesive properties of PVDF retained in the slurries prepared by DMI and PolarClean. Additionally, the energy dispersive spectroscopy (EDS) analysis/mapping and cross-sectional SEM images of three electrodes displayed similar microscopic structures (Fig. 3g–i and Fig. S11, S15, S16, ESI†) where NMC111 formed conducting network filled with sponge-like porous microstructures made by conductive carbon and binder,35 further indicating solvent compatibility with the other ingredients in a slurry. Similar results were obtained for composite films containing other active materials (LiMn2O4 composites are shown in Fig. S12, ESI†). Taken together, these results highlight the fact that DMI and PolarClean allow us to produce similar slurries and electrode film properties to traditional NMP solvent preparation methods.
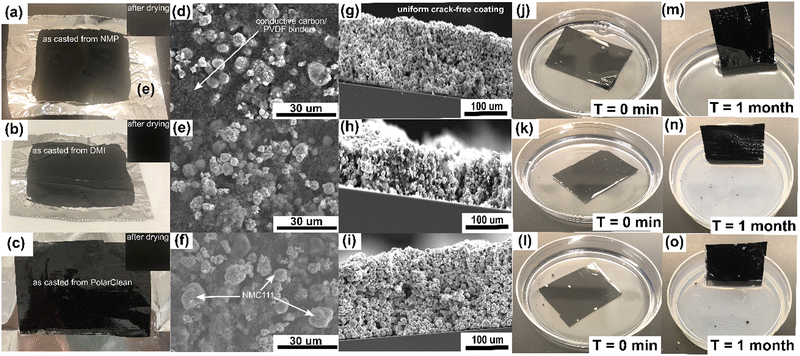 |
| Fig. 3 Photograph of home-made composite NMC111 films as casted and dried (inscribed) from solvents NMP, DMI and PolarClean, respectively (a–c). Top-view/surface and cross-section SEM micrographs of the dry films are shown in (d–i). Film stability in contact with LiPF6 electrolyte in DMC/EC solvent mixture was tested for a month, photographs of the films made by NMP (j and m), DMI (k and n) and PolarClean (l and o) are shown. | |
Electrochemical testing
To investigate the effect of solvent on electrochemical performance, we compared the capacity retention of Li/NMC half cells containing composite cathodes produced with slurry coating from NMP, DMI, and PolarClean (Fig. 4). We found that cells fabricated with PolarClean exhibited an average specific charge capacity at 155 ± 1 mA h g−1 (Fig. 4, green) while the NMP processed cells had an average specific charge capacity of 152 ± 3 mA h g−1 (Fig. 4, red) over 100 cycles. Both achieved similar average coulombic efficiencies (CEs) of 99.6% and 99.7% for NMP and PolarClean processed electrodes, respectively over 100 cycles. These performance metrics suggest that PolarClean is a promising green solvent candidate in cathode fabrication. Interestingly, the higher initial capacity exhibited by cells made with PolarClean (197 mA h g−1) vs. NMP (175 mA h g−1) is concomitant with higher overall capacity retentions over 100 cycles (66% for NMP vs. 75% for PolarClean). When examining the voltage profiles of those cells (Fig. S17 and S18, ESI†), we see stark differences. We find that the irreversible capacity in the first cycle is higher for cells made using PolarClean (58.8 mA h g−1) than NMP (27.4 mA h g−1), suggesting that films processed with PolarClean may promote different side reactions on the electrode surface. While the irreversible capacities for NMP cells decrease slightly over the first three cycles, the large irreversible capacity on the first cycle in PolarClean cells is lessened significantly in further cycles (on average, the irreversible capacities in the first three cycles of PolarClean cells are 58.8, 33.9, 19.8 mA h g−1 respectively, while for NMP cells they are 27.4, 13.6, and 13.8 mA h g−1). Interestingly, while in NMP cells, the discharge capacity remains largely constant over 3 cycles, the discharge capacities in cycles 2 and 3 in PolarClean cells are ∼13 mA h g−1 higher than in the first cycle, suggesting that the buildup of a stable cathode electrolyte interphase (CEI) in the first cycle may enable more complete lithiation in subsequent cycles. Notably, we did not observe diminished performance as we would expect if residual PolarClean remained in the electrode, further suggesting that we achieved successful elimination of PolarClean during the electrode drying process (described in ESI†) which is very energy intense during electrode fabrication.36 In contrast, the average charge capacity of the electrode casted from DMI at RT was only 104 mA h g−1, with a capacity retention 29% and CE of 98.8% (Fig. 4, blue), possibly due to the lack of PVDF dissolution. To determine if PVDF solubility was the issue, we fabricated cathodes after dissolving PVDF in DMI at 120 °C instead of RT prior to film casting. Although films cast at 120 °C show slightly higher average charge capacities of 120 mA h g−1 (Fig. S13, ESI†), capacity retention (41%), and CE (99.1%), they still did not achieve the same performances as those observed from films fabricated using NMP or PolarClean. SEM and rheological analyses indicate that the microscopic and shear-thinning behavior of the electrode films and the slurries made by DMI is similar to the NMP and PolarClean. Likewise, PVDF binder exposed to DMI was not altered structurally, confirmed by 1H NMR and FTIR.17 However, the difference in electrochemical performance, sloping overpotentials and low specific capacities (Fig. 4 and Fig. S19, ESI†) suggest that residual solvent molecules from the casting process may impact interphase formation or other parasitic reaction pathways that causes poor capacity retention in LIBs, preventing the use of DMI in carbonate electrolytes and explaining the differences in electrochemical behavior between NMP and PolarClean. Further investigation is currently underway to improve battery performance by altering the slurry mixing sequence,32,33 establishing a relation between solution viscosity and solute/solvent concentration to determine the PVDF chain entanglement at various stages of slurry preparation, standardizing the dispersion and conductivity of the slurry ingredient, detailed study of resistance or impedance measurements, and optimizing the adhesion of the slurry on the Al substrate by tailoring coating and drying conditions.37
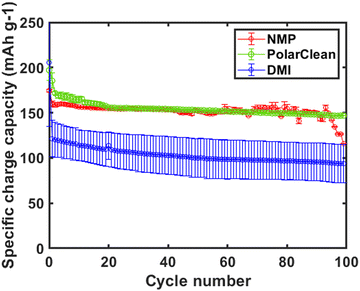 |
| Fig. 4 Cycling performances of the electrodes made using NMP, PolarClean and DMI slurry prepared and processed at RT. Error bars represent standard error of the mean (N = 3 for NMP, N = 2 for PolarClean and DMI). | |
Conclusions and challenges
Using green solvents in place of traditional hazardous solvents will lead to more sustainable LIB chemistries, which remains an important challenge facing the industry today. With the prevalence of green chemistry, we find that PolarClean may be used as an alternative solvent instead of NMP for casting cathode films for LIBs, and it exhibits similar solubility of the PVDF binder, shear thinning rheological properties, stable coating microstructure, room temperature casting process, and excellent electrochemical stability. While DMI is a potentially promising green solvent candidate for slurry casting, and a number of advanced synthetic approaches have been developed towards its large-scale production,15,38–40 it seems to facilitate parasitic side reactions after film coating that need to be addressed prior to application in LIBs. At present, the price of PolarClean (∼$9 per kg) might be an impeding factor for widespread use compared to NMP ($2–3 per kg),41 but it is worth noting that Solvay is currently producing PolarClean on a ton scale using a circular economy approach42 and more research on advanced synthetic routes21 might reduce the solvent cost significantly.
Conflicts of interest
The authors declare no conflict of interest.
Acknowledgements
A. S. acknowledges support from startup fund provided by Montclair State University (MSU) and partial support from National Science Foundation (CBET-2138438). R. M. acknowledges support from the US Department of Defense through the National Defense Science & Engineering Graduate (NDSEG) Fellowship program. Z. V. is supported by the MSU CSAM Faculty-Student Summer Undergraduate Research Program. L. M. acknowledges supports from Columbia University.
Notes and references
- A. Sarkar, R. May, S. Ramesh, W. Chang and L. E. Marbella, ChemistryOpen, 2021, 10, 545–552 CrossRef CAS
.
- A. Bottino, G. Capannelli, S. Munari and A. Turturro, J. Polym. Sci., Part B: Polym. Phys., 1988, 26, 785–794 CrossRef CAS
.
- W. Xie, T. Li, A. Tiraferri, E. Drioli, A. Figoli, J. C. Crittenden and B. Liu, ACS Sustainable Chem. Eng., 2021, 9, 50–75 CrossRef CAS
.
-
M. McCoy, C&EN Global Enterprise, 2019, vol. 97, p. 9 Search PubMed.
- K. Sitarek and J. Stetkiewicz, Int. J. Occup. Environ. Health, 2008, 21, 73–80 Search PubMed
.
- US-EPA Draft Evaluation for N-methylpyrrolidone (NMP). https://www.epa.gov/assessing-and-managing-chemicals-under-tsca/draft-risk-evaluation-n-methylpyrrolidone-nmp (accessed November 1, 2021).
- F. A. Cetinel and W. Bauer, Bull. Mater. Sci., 2014, 37, 1685–1690 CrossRef CAS
.
- R. Sahore, D. L. Wood III, A. Kukay, K. M. Grady, J. Li and I. Belharouak, ACS Sustainable Chem. Eng., 2020, 8, 3162–3169 CrossRef CAS
.
- H. Zhou, B. Pei, Q. Fan, F. Xin and M. S. Whittingham, J. Electrochem. Soc., 2021, 168, 040536 CrossRef CAS
.
- J. Feng, D. Wang, Q. Zhang, J. Liu, Y. Wu and L. Wang, ACS Appl. Mater. Interfaces, 2021, 13, 44312–44320 CrossRef CAS
.
- M. Wang, X. Dong, I. C. Escobar and Y. T. Cheng, ACS Sustainable Chem. Eng., 2020, 8, 11046–11051 CrossRef CAS
.
- V. R. Ravikumar, A. Schroder, S. Kohler, F. A. Cetinel, M. Schmitt, A. Kondrakov, F. Eberle, J.-O. Eichler-Haeske, D. Klein and B. Schmidt-Hansberg, ACS Appl. Energy Mater., 2021, 4, 696–703 CrossRef CAS
.
- O. Chernysh, V. Khomenko, I. Makyeyeva and V. Barsukov, Mater. Today: Proc., 2019, 6, 42–47 CAS
.
- K. Kim and S.-E. Lee, Chemosphere, 2021, 270, 129405 CrossRef CAS PubMed
.
- F. Arico and P. Tundo, Beilstein J. Org. Chem., 2016, 12, 2256–2266 CrossRef CAS PubMed
.
- F. Russo, F. Galiano, F. Pedace, F. Arico and A. Figoli, ACS Sustainable Chem. Eng., 2020, 8, 659–668 CrossRef CAS
.
- O. Buken, K. Mancini and A. Sarkar, RSC Adv., 2021, 11, 27356–27368 RSC
.
- J. T. Jung, H. H. Wang, J. F. Kim, J. Lee, J. S. Kim, E. Drioli and Y. M. Lee, J. Membr. Sci., 2018, 559, 117–126 CrossRef CAS
.
- N. T. Hassankiadeh, Z. Cui, J. H. Kim, D. W. Shin, S. Y. Lee, A. Sanguineti, V. Arcella, Y. M. Lee and E. Drioli, J. Membr. Sci., 2015, 479, 204–212 CrossRef CAS
.
- V. Paolucci, G. D’Olimpio, L. Lozzi, A. M. Mio, L. Ottaviano, M. Nardone, G. Nicotra, P. Le-Cornec, C. Cantalini and A. Politano, ACS Sustainable Chem. Eng., 2020, 8, 18830–18840 CrossRef CAS PubMed
.
- L. Cseri and G. Szekely, Green Chem., 2019, 21, 4178–4188 RSC
.
- U. S. Environemntal, Protection Agency, Methyl 5-(dimethyl-amino)-2-,ethyl-5-oxopentanoate, exemption from the Requirement of a tolerance, Fed. Regist., 2013, 78, 32157–32161 Search PubMed
.
- Annex XVII to Commission Regulation (EC) No. 1907/2006 of the European Parliament and of the Council Concerning the Registration, Evaluation, Authorization and Restriction of Chemicals (REACH) as regards 1-Methyl-2-Pyrrolidone. Official Journal of the European Union, 2018, vol. L 99, pp. 3–6.
-
S. L. Waaijers-van der Loop, Z. Dang, E. Rorijie and N. Janssen, Toxicity Screening of Potential Bio-Based Polar Aprotic Solvents (PAS). RIVM Memo-December 2018 v1.0. https://www.rivm.nl/sites/default/files/2019-02/Toxicity%20screening%<?pdb_no 20of?>20of<?pdb END?>%20potential%20bio-based%20Polar%20Aprotic%20Solvents.pdf (accessed November 1, 2021).
- National Industrial Chemicals Notification and Assessment Scheme (NICNAS). File No. STD/1052-May2004.
- N. Ismail, M. Essalhi, M. Rahmati, Z. Cui, M. Khayet and N. Tavajohi, Green Chem., 2021, 23, 2130–2147 RSC
.
- A. Kumar, A. Sharma, B. G. de la Torre and F. Albericio, Green Chem. Lett. Rev., 2021, 14, 545–550 CrossRef CAS
.
-
C. M. Hansen, Hansen Solubility Parameters: A User's Handbook, CRC Press, 2007 Search PubMed
.
- R. K. Salman and J. M. Salih, Int. J. Mater. Sci. Appl., 2016, 5, 183–187 CAS
.
- E. Tocci, C. Rizzuto, F. Macedonio and E. Drioli, Ind. Eng. Chem. Res., 2020, 59, 5267–5275 CrossRef CAS
.
- W. B. Hawley and J. Li, J. Energy Storage, 2019, 26, 100994 CrossRef
.
- A. Kraytsberg and Y. Ein-Eli, Adv. Energy Mater., 2016, 6, 1600655 CrossRef
.
- G.-W. Lee, J. H. Ryu, W. Han, K. H. Ahn and S. M. Oh, J. Power Sources, 2010, 195, 6049–6054 CrossRef CAS
.
- W. B. Hawley, A. Parejiya, Y. Bai, H. M. Meyer III, D. L. Wood III and J. Li, J. Power Sources, 2020, 466, 228315 CrossRef CAS
.
- M. Wang, D. Dang, A. Meyer, R. Arsenault and Y.-T. Cheng, J. Electrochem. Soc., 2020, 167, 100518 CrossRef CAS
.
- D. L. Wood III, J. D. Quass, J. Li, S. Ahmed, D. Ventola and C. Daniel, Drying Technol., 2018, 36, 234–244 CrossRef
.
- J. Li, J. Fleetwood, W. B. Hawley and W. Kays, Chem. Rev., 2022, 122, 903–956 CrossRef CAS PubMed
.
- P. Tundo, F. Arico, G. Gauthier, L. Rossi, A. E. Rosamilia, H. S. Bevinakatti, R. L. Sievert and C. P. Newman, ChemSusChem, 2010, 3, 566–570 CrossRef CAS PubMed
.
- F. Arico, A. S. Aldoshin and P. Tundo, ChemSusChem, 2017, 10, 53–57 CrossRef CAS PubMed
.
- M. Annatelli, D. D. Torre, M. Musolino and F. Arico, Catal. Sci. Technol., 2021, 11, 3411–3421 RSC
.
- C. Ursino, F. Russo, R. M. Ferrari, M. P. DeSanto, E. Di Nicolo, T. He, F. Galiano and A. Figoli, J. Membr. Sci., 2020, 608, 118216 CrossRef CAS
.
- F. Campana, B. M. Massaccesi, S. Santoro, O. Piermatti and L. Vaccaro, ACS Sustainable Chem. Eng., 2020, 8, 16441–16450 CrossRef CAS PubMed
.
|
This journal is © The Royal Society of Chemistry 2022 |