DOI:
10.1039/D2VA00091A
(Tutorial Review)
Environ. Sci.: Adv., 2022,
1, 401-425
Significance of anaerobic oxidation of methane (AOM) in mitigating methane emission from major natural and anthropogenic sources: a review of AOM rates in recent publications†
Received
12th May 2022
, Accepted 24th July 2022
First published on 22nd August 2022
Abstract
Methane is estimated to have contributed 20% of postindustrial global warming. Methanotrophs oxidize methane and curb methane emissions into the atmosphere. Anaerobic oxidation of methane (AOM) has been recognized as an important methane sink. Sulfate is the primary electron acceptor of AOM in the marine environment, while nitrite/nitrate is encountered more often in terrestrial water-logged systems, such as rice paddy and wetlands. A key aspect of AOM is the reaction rate, which influences methane fluxes to the oxic zones and eventually the atmosphere. We collated the AOM rates from major natural and anthropogenic sources in recent publications and found that AOM rates are generally lower than the corresponding aerobic methane oxidation rates in wetlands and rice paddy, while the AOM rates are often higher than the corresponding aerobic oxidation rates in freshwater systems and marine environments. Based on the median reaction rates and estimated aerobic and anoxic zone coverages, AOM consumes approximately 71%, 8%, 5%, 13%, and 3% of the methane entering the anoxic zones in oceans, wetlands, paddy systems, lakes/reservoirs, rivers, respectively. These analyses suggest that AOM is a key methane sink in oceans, while aerobic methanotrophs consume more methane in the other studied ecosystems. Finally, the controlling factors of AOM and some issues in the rate quantification were discussed. It is believed that more comprehensive studies of AOM and improved rate quantification would assist in forecasting methane emission, which fosters scientific debate over global warming and eventually affects climate policymaking.
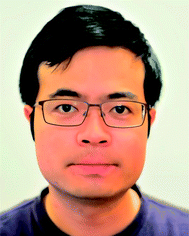 Yaohuan Gao | Dr Gao is currently an Associate Professor in the Department of Environmental Science and Engineering and a member of the Institute of Global Environmental Change at Xi'an Jiaotong University. He holds B.S. and M.S. degrees in Environmental Engineering from Tianjin University and Duke University, respectively, and a Ph.D. in Environmental Engineering from the University of Waterloo. Dr Gao's interests focus on biotechnology development for resource recovery from waste biomass, with particular emphasis on methane generation and oxidation in bioreactors. |
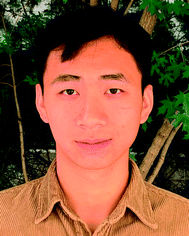 Yong Wang | Yong Wang is a M.S. student in the Department of Environmental Engineering at Xi'an Jiaotong University. He holds a B.S. degree in Environmental Engineering from the Xi'an University of Architecture and Technology. Yong Wang's current research focuses on the response of anaerobic oxidation of methane to co-existing pollutants. |
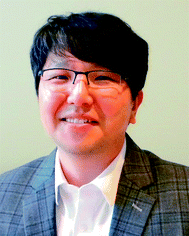 Hyung-Sool Lee | Dr Lee earned his B.S. and M.S. in Environmental Engineering from Inha University in Korea. He holds a Ph.D. in Environmental Engineering from Arizona State University. Dr Lee was an Assistant Professor (2010–2017) and Associate Professor (2017–2022) at the University of Waterloo and is now a Professor at the Korea Institute of Energy Technology (KENTECH). His research interests include resource recovery from bio-waste and wastewater, bioelectrochemistry, microbial ecology, anaerobic oxidation of methane, denitrification, and biosensors. |
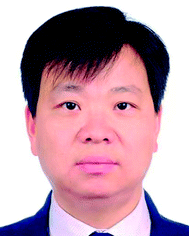 Pengkang Jin | Dr Jin is a Professor in the Department of Environmental Science and Engineering and a member of the Institute of Global Environmental Change at Xi'an Jiaotong University. Dr Jin leads a research team that focuses on wastewater treatment and resource recovery. He is also the Director of the Institute of Water Cycling & Carbon Neutrality at Xi'an Jiaotong University. Before joining Xi'an Jiaotong University, he was a Professor (2010–2021) and the Associate Dean (2018–2021) in the School of Environment and Municipal Engineering at the Xi'an University of Architecture and Technology. His research interests include wastewater treatment and reclamation, pollutant transformation in the sewer system, and industrial pollutant control. |
Environmental significance
Anaerobic oxidation of methane (AOM) has been a focus of study for a few decades but there is still a lack of comparison of AOM rates across different methane sources. Besides, the current methane budget and global methane cycling analyses often do not consider AOM as a methane sink due to insufficient information on the distribution of AOM activity. The simplified analysis of methanotrophic rates in this study showed that anaerobic methanotrophs are sometimes more efficient than aerobic methanotrophs. A generalized model for AOM rate prediction does not exist, and the usually slow growth of anaerobic methanotrophs may be a key reason behind these low rates. A more systematic AOM study and improved AOM rate quantification will help us better understand the cycling of methane in nature and better control the emission of this greenhouse gas.
|
1. Introduction
Methane is a potent greenhouse gas that has 25 to 35 times the global warming potential of CO2 over a century timescale.1 Methane can be released from both natural (e.g., wetlands, lakes/ponds, rivers, and oceans) and anthropogenic sources (e.g., rice paddy).1 The amount of methane entering the atmosphere usually depends on not only methanogenesis (i.e., the microbial formation of methane) but also methanotrophy (i.e., the microbial processes that consume methane, aerobic or anoxic, as shown in Fig. 1). AOM has been reported in all the aforementioned environments as an important methane degradation pathway and a growing number of publications suggest that AOM is an integral part of the methane sink.2–7 Although “anaerobic” is used in AOM, AOM usually occurs under anoxic conditions with common electron acceptors other than oxygen (Fig. 1). To avoid potential misunderstanding by readers from various disciplines, this review used “anoxic” if possible. For example, in marine sediments where methane generated deep in the seabed travels upwards by diffusion, almost all the methane generated is oxidized by anaerobic methanotrophs (ANME) and their bacterial partners before entering the seawater.8,9 The pathway by which ANME utilizes methane is commonly agreed upon to be the reversal of methanogenesis.9 The bacterial partners are often related to sulfate-reducing bacteria (SRB), and these bacteria metabolize sulfur compounds, which serve as electron acceptors (eqn (1); eqn (2) and (3) represent one of the possible reaction pathways, and the sum of the two steps leads to eqn (1)).10 So far, three main marine clades, ANME-1a/b, ANME-2a/b/c, and ANME-3, have been identified.11,12 The reports of AOM coupled with denitrification around 2004 (ref. 2 and 13) imply that AOM can be coupled with the reduction of nitrate/nitrite, not just sulfate. Later, studies showed that methanotrophic bacteria and ANME can also couple the oxidation of methane to the reduction of other electron acceptors such as iron11 and these electron acceptors sometimes decouple methane oxidation from sulfate reduction.8 | CH4 + SO42− → HCO3− + HS− + H2O (ref. 15) | (1) |
| 7CH4 + 8SO42− + 5H+ → 4HS2− + 7HCO3− + 11H2O (ref. 10) | (2) |
| 4HS2− + 4H2O → SO42− + 7HS− + 5H+ (ref. 10) | (3) |
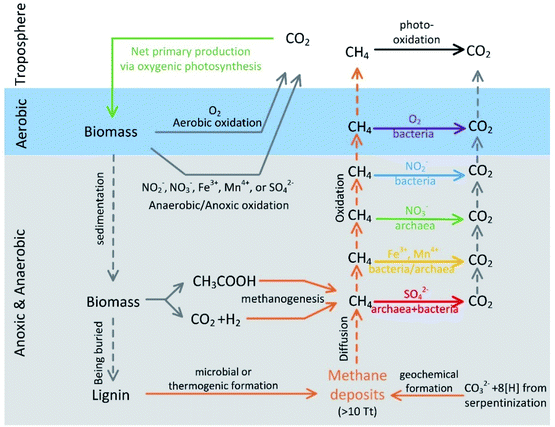 |
| Fig. 1 A sketch of the carbon cycle with a focus on methane generation and oxidation pathways (reproduced from R. K. Thauer14 with permission from Elsevier, copyright [2011]). | |
AOM has also been observed in anoxic freshwater and terrestrial environments, such as lakes, rivers, wetlands, paddy systems, and even deep underground in fractured granitic rocks.4,16–20 DAMO (denitrifying anaerobic methane oxidation) has been demonstrated as a major AOM pathway in terrestrial environments.21–24 DAMO entails two different processes, i.e. nitrite-DAMO and nitrate-DAMO (eqn (4) and (5)). The former is proposed to be intracellular aerobic methane oxidation,2 first found to be catalyzed by the NC10 bacterium “Candidatus Methylomirabilis oxyfera”. Later, some other species from the NC10 phylum have been identified, i.e. M. sinica,25M. limnetica,26 and M. lanthanidiphila.27 The nitrate-DAMO was found to be carried out by anoxic archaea belonging to the ANME-2d clade,28 which is more prevalent in terrestrial environments than other ANME-2 clades.28–30 A frequently reported nitrate-reducing archaeal species, named “Candidatus Methanoperedens nitroreducens”, also applies reverse methanogenesis for AOM.28 Besides, recent studies also indicate that DAMO archaea can couple AOM with the reduction of Fe(III) (eqn (6)) and sulfate,31,32 implying that DAMO may also be active in environments with non-nitrogen-based electron acceptors.
| 2CH4 + 8NO3− → 2CO2 + 8NO2− + 4H2O (ref. 33) | (4) |
| 3CH4 + 8NO2− + 8H+ → 3CO2 + 4N2 + 10H2O (ref. 33) | (5) |
| CH4 + 8Fe3+ + 2H2O → CO2 + 8Fe2+ + 8H+ (ref. 32) | (6) |
The research of AOM has been under development for approximately five decades.29,34 The progress and evolution in the past decade or so can be largely visualized by the co-citation network based on recently published research articles and review papers (Fig. 2). Generally, AOM studies have expanded to environments other than methane seeps or marine sediment. The research topics or keywords that could be considered central to AOM research can be found in Fig. 2B. For example, DAMO is becoming one of the prominent research areas. The interactions between carbon cycling and nitrogen cycling in freshwater ecosystems and engineered systems have been two other research focuses.
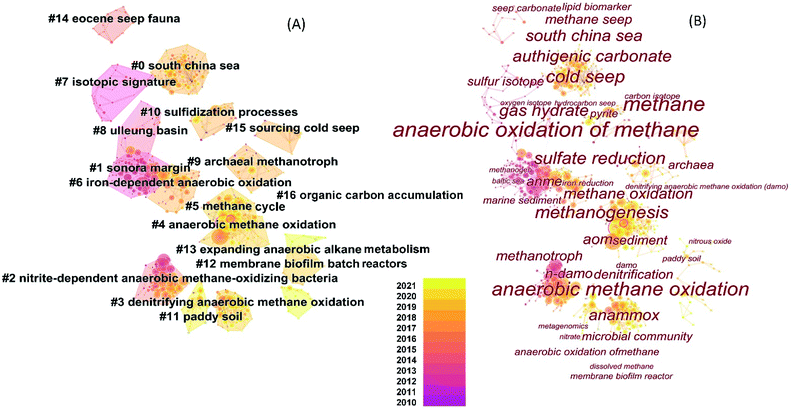 |
| Fig. 2 Clustering analysis (CiteSpace V5.3.R11) of the anaerobic methane oxidation co-citation network (A), generated by the top 30 per slice between 2010 and 2021. In this visualization, the earliest work (i.e., 2010) appeared from the left of the network (purple), whereas the most recent ones are close to the right (gold). This network is decomposed into clusters of references based on the strengths of co-citation links, and the clusters are numbered in the descending order of their size. The largest one is numbered as 0, followed by 1, and so on. Names of the clusters were based on the titles of all studied publications (1384 publications and 46 091 distinct references) with the log-likelihood ratio algorithm. (B) The network with the major nodes labeled by the extracted keywords from the titles and abstracts based on the frequency of usage. The bigger size of a keyword indicates a higher frequency of usage. A node represents an article. A larger node means a higher frequency of citation. | |
Instead of detailing the various reaction pathways, reaction thermodynamics, biochemical mechanisms, microbial physiology, and the various implications in natural and engineered systems like other reviews,7,9,35–44 this review article concentrates on recently published AOM rates in the literature (ca. 2010–2021). The AOM rates were organized according to the environment where the reactions occur. In both major natural and anthropogenic methane sources, the AOM rates are compared with the corresponding aerobic rates of methane oxidation. Additionally, the AOM capabilities of various methanotrophs enriched in biological reactors, mainly DAMO microorganisms,23,33,45–53 are summarized as references for the currently highest possible AOM rates. To reveal the significance of AOM as a methane sink, the annual rates of methane oxidation in major methane sources were estimated. Finally, the controlling factors for AOM and some issues in the rate quantification were briefly discussed.
2. AOM in major methane sources
2.1 Terrestrial and freshwater environments
2.1.1 Wetlands.
Freshwater wetlands account for one-third of total methane emissions to the atmosphere.54 Besides, the methane emission from freshwater wetlands is almost 290 times the total methane emission from coastal wetlands or coastal vegetated ecosystems (e.g., mangroves, seagrasses, and salt marshes).55 Thus, the discussion in this section mainly focused on freshwater wetlands. In addition to aerobic oxidation induced by wetland vegetation, which oxidizes 16% to over 90% of the methane at their roots and/or plant parts in the water-logged soils,56 aerobic methanotrophs in oxic zones oxidize methane at the rates of 0.03 nmolCH4 cm−3 d−1 to 3.6 × 103 μmolCH4 cm−3 d−1 (median = 0.16 μmolCH4 cm−3 d−1, Q1–Q3 [first to third quartiles]: 0.04–0.7 μmolCH4 cm−3 d−1) (Fig. 4), accounting for 2–79% of the methane efflux to the atmosphere.57–61 AOM was found to be another methane sink20,62 in freshwater wetlands and was estimated to reduce methane emissions by around 5.7% to over 50%.4,63 AOM in wetlands has been reported to be mainly catalyzed by DAMO microorganisms, including DAMO bacteria and DAMO archaea. For example, according to the detection of pmoA (the gene targeting the alpha subunit of particulate methane monooxygenase, a gene marker for DAMO bacteria64) and the 16S rRNA genes of DAMO bacteria, 68% and 90% of the studied wetlands in China showed DAMO activity.65 The incubation of soils from the Zoige National Wetland Reserve, on the Tibetan Plateau, indicates that both DAMO bacteria and archaea were active:63 in the warm season, nitrite reduction was high in the 10–30 cm section and nitrate reduction was high in the 30–60 cm section, ranging from 0.36 to 4.33 nmolCO2 g−1 d−1 and 0.89 to 9.51 nmolCO2 g−1 d−1 by DAMO bacteria and archaea, respectively (note: the CH4- and CO2-based rates are considered equivalent here, due to the often unavailable information about carbon assimilation during AOM. The reported values thus potentially underestimated the true rates). Although M. oxyfera and/or M. oxyfera-like bacteria activate methane using oxygen,2 the proliferation of DAMO bacteria requires anoxic conditions as created in water-logged soils65 or deep underground (e.g., 50–100 cm depth).62 The distribution of DAMO microorganisms depends on both the availability of electron acceptors and methane; the latter may be more influential as demonstrated by the difference in the depth distribution of the abundances of nitrate/nitrite and the pmoA gene.66
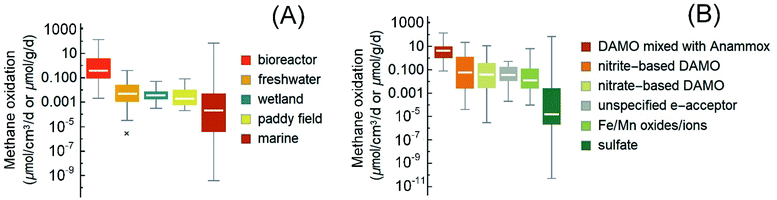 |
| Fig. 3 Box-and-whisker plots of the reported rates of AOM in the literature (2010–2021) and the cited references therein. (A) The rates grouped according to the ecological habitats. Reaction rates in bioreactors are included as a reference for the maximum possible AOM rates. (B) The rates grouped according to reaction type/electron acceptors. In panel B, both rates from short-term incubation with natural samples and these long-term enriched cultures in bioreactors are plotted. The boxes are ranked from left to right according to the medians (marked by white horizontal lines in the boxes). Median values, instead of averages, are used for methane budget estimations in this review to avoid interference from extreme values. Most outliers were removed following a method reported elsewhere.1 Please refer to the ESI† for the rates used in the plots. | |
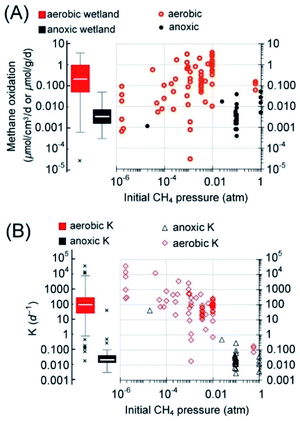 |
| Fig. 4 Rates of aerobic methanotrophy (n = 79) and anoxic methanotrophy (n = 27) in freshwater wetlands (A). The reaction rates and the initial methane partial pressures applied/measured during rate quantification were from the cited references in the text. (B) The fractional turnover rate. The box covers the interquartile interval and the whiskers extend to 1.5 times the interquartile range above Q3 or below Q1. White horizontal line marks the median. | |
In addition to nitrite and nitrate, other electron acceptors such as sulfate and ferric iron have all been shown to stimulate AOM in wetland soils.4,67,68 For instance, iron-mediated AOM may occur in freshwater sediments, where the concentrations of nitrate and sulfate are low.69 Besides, natural organic matter, relying on its redox-active quinone moieties, has also been reported to stimulate AOM in water-logged soils in wetlands, and the enriched water-saturated soils with humic substances derived from Pahokee peat can increase AOM activity to approximately 100 nmolCH4 cm−3 d−1.70 Unclassified marine benthic group B and D families, both belonging to Euryarchaeota, were thought to have carried out AOM, whereas Clostridia and Bacilli were assumed to mediate the reduction of humic substances.70 Humic substances may also serve as electron mediators during AOM driven by the reduction of N2O.71 Hence, the previously observed insignificant enhancement of AOM in several North American peatlands with nitrate/sulfate/ferric iron20 might be explained by the interference caused by natural organic matter.
Recent reports indicate that the rates of AOM in different wetlands span a wide range, 0.31 to 50 nmolCH4 cm−3 d−1 (median = 3.4 nmolCH4 cm−3 d−1 and Q1–Q3: 1.8–7.0 nmolCH4 cm−3 d−1)4,18,20,62,66,70,72,73 (Fig. 4). Ideally, the estimation of methanotrophic contributions to the overall methane sink should be based on depth integration because the reaction rate is not uniform along the depth. Nevertheless, detailed depth-integrated rates or rates with depth are often not available. Hence, the median of commonly reported active depths is used to estimate the areal flux of methane. Based on the commonly reported active depth of AOM, 40 [Q1–Q3: 20–100] cm,62,63,66,74–77 the active depth of aerobic methane oxidation, 7.5 [Q1–Q3: 3.6–15.3] cm,57,78–81 and the estimated global freshwater wetland area (5.69 × 1012 m2),4 the respective methane oxidation rates suggest that anoxic and aerobic methanotrophs have the capability of reducing methane emission by approximately 45 [Q1–Q3: 23–92] TgCH4 per year and 389 [Q1–Q3: 103–1854] TgCH4 per year, respectively (Table 1). The methane oxidation via AOM extrapolated by Segarra et al.4 is 200 TgCH4 per year (data from three wetlands) and AOM solely due to the oxidation by natural organic matter was estimated to be over 1300 TgCH4 per year (data from one wetland).70 Both these studies showed much higher AOM capacities than the estimated median value in this study. The discrepancy could be attributed to the differences in the total number of wetlands considered here (n = 42). Besides, it has to be mentioned that the reported depth of active AOM in freshwater wetlands is often dependent on the tools for core sampling. The DAMO activity has also been detected down to 12–15 m under the surface.65 Nevertheless, such kind of analysis is scarce. The real contribution of AOM to wetland methane oxidation may be larger than the estimation provided here.
Table 1 Methanotrophic reaction rates and the estimated emission potential components
Habitat |
Component |
Reaction rate (Q1, Q3; μmol per cm3 per day) |
Area (m2) |
Median depth (Q1, Q3; m) |
Oxidation/emission capacity (Q1, Q3; TgCH4 per year) |
Region categorization and associated areas are from Egger et al.;15 the original depths from Egger et al. were skewed and here the outliers were removed following the method described in Section 1 (Fig. 2); the processed and expanded depth values can be found in the ESI.
SMTZ stands for the sulfate–methane transition zone as mentioned in the text.
Refer to the text for the estimation related to aerobic methane oxidation in rivers.
|
Marine |
Anoxic oxidationa |
Inner shelf (0–10 m) |
1.2 × 10−3 (4.1 × 10−4, 1.8 × 10−3) |
2.6 × 1012 |
0.4 (0.3, 0.8) (SMTZb) |
7.3 (2.5, 10.6) |
Inner shelf (10–50 m) |
3.5 × 10−4 (1.4 × 10−4, 1.4 × 10−3) |
9.2 × 1012 |
0.9 (0.4, 2.3) (SMTZ) |
16.9 (6.9, 65.7) |
Outer shelf (50–200 m) |
7.1 × 10−6 (3.8 × 10−6, 2 × 10−3) |
1.3 × 1013 |
0.95 (0.6, 1.8) (SMTZ) |
0.5 (0.3, 140.9) |
Seep system |
0.1 (1.6 × 10−2, 1.7) |
2.0 × 1010 |
0.2 (0.2, 0.3) (SMTZ) |
3.3 (0.4, 39.6) |
Slope (200–2000 m) |
4.1 × 10−6 (1.4 × 10−6, 9.5 × 10−5) |
3.0 × 1013 |
3.6 (1.4, 10.3) (SMTZ) |
2.6 (0.9, 59.9) |
Rise (2000–3500 m) |
6.8 × 10−8 (1.3 × 10−8, 7.2 × 10−7) |
6.3 × 1013 |
14.3 (6.1, 30.3) (SMTZ) |
0.4 (0.1, 3.8) |
|
|
Subtotal
|
|
30.9 (10.9, 320.5) |
Aerobic oxidation |
Coastal region |
4.1 × 10−8 (4.0 × 10−9, 2.5 × 10−7) |
3.2 × 1013 (9% of ocean area) |
530.0 (153.5, 975.0) (aerobic surface seawater) |
4.1 (0.4, 25.2) |
Emission |
|
|
|
|
8.4 (4.8, 28.4) |
|
|
|
Total
|
|
43.4 (16.1, 374.1)
|
Freshwater wetlands |
Anoxic oxidation |
3.4 × 10−3 (1.7 × 10−3, 7.0 × 10−3) |
5.7 × 1012 |
0.4 (0.2, 1) |
45.3 (23.3, 92.4) |
Aerobic oxidation |
1.6 × 10−2 (4.1 × 10−2, 7.4 × 10−1) |
7.5 × 10−2 (3.6 × 10−2, 1.5 × 10−1) |
388.8 (102.6, 1854.2) |
|
Subtotal
|
434.1 (125.8, 1946.7) |
Emission |
|
150.1 (138.3, 164.6) |
|
Total
|
584.2 (264.1, 2111.3)
|
Rice paddy |
Anoxic oxidation |
1.85 × 10−3 (6.2 × 10−4, 9.36 × 10−3) |
9.7 × 1011 (60% of total) |
0.5 (0.2, 0.7) |
2.2 (0.7, 11.1) |
Aerobic oxidation |
0.378 (0.072, 8.4) |
0.01 (0.002, 0.015) |
12.5 (2.4, 278.3) |
|
Subtotal
|
14.7 (3.1, 289.3) |
Emission |
|
29.9 (24.9, 32.1) |
|
Total
|
44.6 (28.0, 321.4)
|
Lakes &reservoirs |
Anoxic oxidation |
1.6 × 10−2 (2.2 × 10−3, 1.1 × 10−1) |
2.7 × 1012 |
0.1 (0.08, 0.2) |
34.8 (4.8, 237.9) |
Aerobic oxidation |
1.15 × 10−3 (3.8 × 10−5, 5.6 × 10−3) |
9.5 (5.3, 18.8) |
172.2 (5.6, 831.4) |
|
Subtotal
|
207.0 (10.4, 1069.2) |
Emission |
|
70.9 (32.1, 170.7) |
|
Total
|
277.9 (42.5, 1239.9)
|
Rivers |
Anoxic oxidation |
1.7 × 10−3 (4.0 × 10−4, 5.0 × 10−3) |
5.3 × 1011 (68% of total) |
0.12 (0.10, 0.14) |
0.6 (0.1, 1.8) |
Aerobic oxidation |
0.1 (3.3 × 10−5, 0.4) |
7.7 × 1011 |
— |
11.3 (0.8, 591.2)c |
|
Subtotal
|
11.9 (0.9, 593) |
Emission |
|
5.8 (1.8, 21) |
|
Total
|
17.7 (2.7, 614)
|
Additionally, it seems that the methane oxidation rate is methane-limited, i.e. a higher rate with a higher methane partial pressure (Fig. 4A). The fractional turnover per unit time,82,83K (per day, rate/concentration), of aerobic methanotrophs was generally four orders of magnitude higher than those under anoxic conditions (Fig. 4B and Table 1). The higher K values of aerobic methane oxidation suggest that aerobic methanotrophs are more active than methanotrophs under anoxic conditions or the abundance of aerobic methanotrophs is higher.82
The recent estimate of the median methane emission from freshwater wetlands is 150.1 [Q1–Q3: 138.3–164.6] TgCH4 per year.55 Therefore, based on the estimated median capacity of anoxic and aerobic methanotrophic activities, a significant amount of methane is oxidized before entering the atmosphere. The sum of efflux to the atmosphere and the potential amount of methane oxidized leads to the estimated median methane emission potential of global freshwater wetlands, 584 TgCH4 per year. It is worth mentioning that wetland soils, especially the section in the deep subsurface, still contain a significant amount of dissolved methane in the porewater.84 This portion of methane is treated as the holding capacity of the wetlands, not considered in the methane emission potential.
2.1.2 Rice paddy soils.
Methane released due to rice cultivation represents 10 to 25% of global methane emissions.85 Aerobic methanotrophs in paddy soils demonstrate oxidation rates of 0.0018–17.7 μmolCH4 g−1 d−1 (median = 0.38 μmolCH4 g−1 d−1 and Q1–Q3: 0.07–8.4 μmolCH4 g−1 d−1)86–88 (Fig. 5A). Regarding AOM in paddy soils, DAMO has been a research focus because paddy soils receive large inputs of nitrogen fertilizers, some of which can be converted to either nitrate or nitrite.85,89–91 Paddy soils often harbor both DAMO archaea and DAMO bacteria.53,89,92 For instance, the analysis of paddy soils in Italy showed that 16S rRNA gene copies of potential DAMO bacteria belonging to the NC10 phylum were 104 to 105 copies per g and even more AOM-associated archaea, including M. nitroreducens, were present.93 Although soils collected from 10–50 cm beneath the ground are often used for AOM studies,86,91–95 DAMO microorganisms have been demonstrated to inhabit a great depth in paddy fields.85,89 For example, in rice paddy soil from the Yangtze River Plain, China, a high abundance of DAMO bacteria (8.5 × 107 to 1.0 × 108 copies per g dry soil) was detected in 60–140 cm soil core sections in the summertime and all soil core sections from 40 cm down to 200 cm in wintertime.90 The reported AOM rates in paddy soils are from 0.2 to 79.9 nmolCO2 g−1 d−1 (median = 1.9 nmolCO2 g−1 d−1 and Q1–Q3: 0.6–9.4 nmolCH4 g−1 d−1; rates from bioreactors using paddy soils as inocula were excluded)85,86,93,94,96–98 (Fig. 5). The probably highest reported nitrate-DAMO rate is 79.9 nmolCH4 g−1 d−1, observed with paddy soils at the Italian Rice Research Unit.93 The highest nitrite-DAMO, 3.9 μmolCH4 g−1 d−1, was obtained with soils from an unspecified non-flooded rice paddy field in China.98 Overall, the AOM rates are not significantly different with nitrate and nitrite as the electron acceptor (Mann–Whitney U-test, p = 0.37, Fig. 3B). The AOM rates are generally lower than the aerobic counterpart, so does the corresponding fractional turnover constant, K (per day), as shown in Fig. 5B. The K values of microorganisms in paddy soils are generally comparable to their counterparts in freshwater wetlands (Fig. 4B). It is noteworthy that many of the AOM studies with paddy soils applied microcosm incubation to quantify AOM rates in flooded soils; however, actual AOM rates may depend on the stage during rice cultivation, which is often not explicitly discussed. Besides, carbon assimilation by DAMO microorganisms is often not reported. Solely from the aspect of missing carbon assimilation data, the actual AOM rate should be higher than the apparent AOM rate. For instance, a recent study demonstrates that a significant amount of methane-derived carbon might be assimilated during DAMO, and the AOM rate based on CO2 measurement underestimated the true rate by approximately 31–62%.99
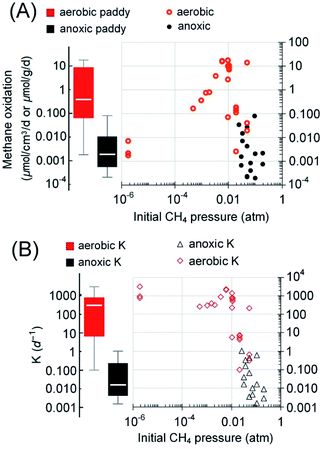 |
| Fig. 5 Rates of aerobic methanotrophy (n = 25) and anoxic methanotrophy (n = 16) in rice paddy soils (A). The reaction rates and the initial methane partial pressures applied/measured during rate quantification were from the cited references in the text. (B) The fractional turnover rate. | |
As aforementioned, the spatial distribution of DAMO microorganisms is in the decimeter to meter range below the ground in paddy soils. In contrast, active aerobic methanotrophy often exists in a millimeter-depth range at the oxic–anoxic interface.100,101 Considering the spatiotemporal variation of both methanogenesis and methanotrophy with the rice cultivation practice, we further limit the estimation to only the irrigated area (paddy soils), which accounts for approximately 60% of the total rice cultivation area,1 and assume that AOM is most active during the growing season (irrigated, max. 152 days1), while the aerobic methanotrophy dominates beyond this period. Using a 50 [Q1–Q3: 20–70] cm depth of active DAMO, 10 [Q1–Q3: 2–15] mm depth of active aerobic methane oxidation,100 an average paddy soil density of 1.3 g cm−3, and the global rice field area (1.62 × 1012 m2),102 AOM and aerobic methanotrophs are capable of reducing methane emission by 2.2 [Q1–Q3: 0.7–11.1] TgCH4 per year and 12.5 [Q1–Q3: 2.4–278.3] TgCH4 per year, respectively. It must be noted that the estimated 75% percentiles based on rates measured under ideal conditions may not be reached during rice cultivation due to the limitation caused by methanogenesis (often not a limiting factor in lab-scale incubations). The lower end of the currently estimated methane oxidation via AOM is close to the estimated values from a recent publication,97 2.2–5.5 TgCH4 per year. Regarding methane emission, the recently estimated efflux from paddy fields in the 2008–2017 decade is 30 [min–max: 25–38] TgCH4 per year1 or 29.9 [Q1–Q3, 24.9–32.1] TgCH4 per year,55 which are comparable. Therefore, based on the comparison with the methane oxidation capabilities, a significant amount of methane is oxidized before entering the atmosphere. If the median methane oxidation capacities were realized, methane oxidized via anoxic and aerobic pathways would be responsible for 5% and 28% of the total methane emission potential, approximately 14.7 [Q1–Q3, 3.1–289.3] TgCH4 per year.
2.1.3 Freshwater.
Recent publications suggest that inland fresh water emits 159 [min–max, 117–212] TgCH4 per year, responsible for approximately 19.5–35.5% of the atmospheric methane budget,1,55,103 much higher than that from the oceans (2–3%). The major sources include lakes/ponds, rivers/streams, and reservoirs, which account for approximately 70%, 17%, and 13% of the total average inland methane efflux from freshwater.1,104,105 Specifically, the estimated median emission is 60.2 [Q1–Q3: 23.7–178.6] TgCH4 per year for lakes and ponds, 5.8 [Q1–Q3: 1.8–21.0] TgCH4 per year for rivers and streams, and 15.1 [Q1–Q3: 8.8–28.4] TgCH4 per year for reservoirs.55 DAMO was found to be a major AOM pathway in freshwater ecosystems.106,107 AOM driven by other electron acceptors has also been reported. For example, metal oxides and sulfates can also be electron acceptors,16,69 but the rates are usually two or three orders of magnitude lower than those of the DAMO pathways.108,109 In this section, AOM and relevant methane emissions from lakes/reservoirs/ponds and rivers/streams are discussed separately because the two water bodies are different in several aspects.
Regarding lakes/ponds, a significant amount of methane may be oxidized before entering the atmosphere, as demonstrated by the almost complete consumption of methane generated deep in the sediment of lakes in high latitudes110 and the high assimilation of carbon derived from methane (47–90%) by microbes.111 Sediments of reservoirs are like that of a lake, and DAMO pathways also exist in reservoir sediments.112,113 For example, the incubation tests with methane and nitrate showed that the sediments from three reservoirs in Poland supported nitrate-DAMO with a rate ranging from 7.2 to 24.96 nmolCH4 g−1 d−1.114 AOM with unknown electron acceptors was also discovered in sediments of a small-scale dam reservoir located in Rzeszów, Poland, where the estimated AOM rate was 8.6–34.1 nmolCH4 g−1 d−1.115 Similar AOM rates were observed in Jiulonghu reservoir in China, ranging from 4.7 to 14.1 nmolCO2 g−1 d−1 with nitrite and 0.8 to 2.6 nmolCO2 g−1 d−1 with nitrate.116 In freshwater lakes/ponds/reservoirs, the rate of aerobic methane oxidation ranges from 0.0008 to 49 nmolCH4 cm−3 d−1 (median = 1.2 nmol cm−3 d−1; Q1–Q3: 0.04–5.6 nmolCH4 cm−3 d−1)117–121 (Fig. 6A). AOM rates range from 0.06 to 400 nmol cm−3 d−1 (median = 16 nmol cm−3 d−1; Q1–Q3: 2–108 nmolCH4 cm−3 d−1).6,31,106,107,110,111,119,122–125 The median AOM rate in lakes/ponds/reservoirs is higher than that of the aerobic methane oxidation (Mood's median test, p < 0.0001; for all freshwater systems, p = 0.002). The reason for this contrast is not clear because the methane oxidation catalyzed by methane monooxygenases from aerobic methanotrophic bacteria is generally 1–2 orders of magnitude higher than that catalyzed by ANME methyl coenzyme M reductase.126
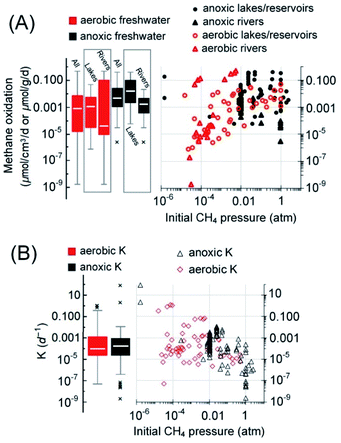 |
| Fig. 6 Rates of aerobic methanotrophy (n = 65) and anoxic methanotrophy (n = 93) in freshwater (A). The reaction rates and the initial methane partial pressures applied/measured during rate quantification were from the cited references in the text. (B) The fractional turnover rate. | |
It must be noted that AOM also exists in the water column of some lakes/reservoirs (8–23 nmolCH4 cm−3 d−1),110,119,127 but insufficient data are available for the estimation of this fraction of AOM. Therefore, only AOM in the sediments and the aerobic methane oxidation in the bulk water phase are considered here in the simplified AOM capacity estimation. The average depth of lakes on Earth is 41.8 m,128 and the top 9.5 [Q1–Q3: 5.2–18.7] m in the water of lakes/reservoirs worldwide is usually found to be oxic.104,117,120,129 Taking this oxic layer depth and the global area of lakes and reservoirs, 2.7 × 1012 m2,130 for estimation, the aerobic methanotrophs are capable of oxidizing approximately 172 [Q1–Q3: 6–831] TgCH4 per year (Table 1). Similarly, if the median thickness of sediment with active AOM in lakes/reservoirs, 14 [Q1–Q3: 8–18] cm (ref. 6, 108, 123 and 131–134), is taken for estimation, AOM solely in the sediments is capable of reducing methane emission by 35 [Q1–Q3: 5–238] TgCH4 per year. Therefore, if the median capacities of methane oxidation were realized, aerobic methane oxidation is the major methane sink (Fig. 7, approximately 62%), stronger than AOM (approximately 13%), similar to previous conclusions.6,111
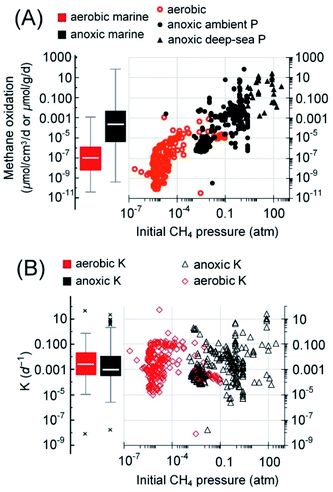 |
| Fig. 7 Rates of anoxic methanotrophy and the rates of aerobic methanotrophy in the marine environment (A). Reaction rates of anaerobic oxidation of methane (n = 386) and aerobic methanotrophy (n = 223) and the initial methane partial pressures were collected from the cited references and online datasets. (B) The fractional turnover rate. | |
Rivers/streams are another major freshwater body on Earth. Partly due to the difficulties in the methane flux quantification, methanotrophic activity in rivers/streams was once ignored during methane flux analysis.135 According to recent studies, significant methanotrophic activity (at Tg per year level, mainly aerobic) exists in riverine systems such as the Amazon River.103,136,137 The methane consumed aerobically accounts for 28–96% of the methane content in the bulk water in the Amazon basin.103 Regarding AOM, DAMO has also been reported as a major AOM pathway in river sediments.31,138,139 Available studies indicate that the methanotrophy in rivers depends on the riverbed sediment types. For example, a previous study of chalk rivers indicates that fine sediments can support strong methanotrophic activity that even surpasses methanogenesis,140 and nitrate/nitrite-DAMO, ranging from 1.2 to 53.6 nmolCO2 g−1 d−1, was only detected in sandy sediments of four representative rivers in southeast England but not in more oxic gravel sediments.31 These authors further extrapolated that DAMO could account for 35% of all the methane oxidized in sandy riverbeds before entering the atmosphere in the UK.30,31 In a study of the sediments from Jordan River in Salt Lake City, USA, DAMO activity 0.044 to 0.101 nmolCH4 g−1 d−1 was detected, and the rate per NC10 phylum gene copy was independent of the depth of sediment.122 The methanotrophs are active from 2 to 15 cm in the sediment, and the rates generally peaked at 4–8 cm below the interface.30 Overall, the AOM rates range from 0.003 to 21 nmolCH4 g−1 d−1 (median = 1.7 nmolCH4 g−1 d−1; Q1–Q3: 0.4–5 nmolCH4 g−1 d−1).31,122,141
Based on these previous studies, it is conceivable that for rivers contaminated by agricultural run-off, the DAMO process may be more active. However, compared with AOM in lakes and wetlands, information about the AOM rates and distribution of methanotrophs in rivers is still insufficient.5 Taking the average global area of rivers and streams,142 7.73 × 1011 m2, and a median depth of the active AOM zone of 12 [Q1–Q3: 10–14] cm, further restricts the estimate to only sandy riverbeds (estimated median = 68%),31,143,144 solely AOM in the sediment of rivers/streams is capable of reducing methane emission by 0.6 [Q1–Q3: 0.1–1.8] TgCH4 per year. As for the aerobic methane oxidation in rivers and streams, the reported rates span a wide range, from 2 × 10−6 nmolCH4 g−1 d−1 to 0.48 μmolCH4 g−1 d−1.140,145 Here, similar issues exist and hinder the estimation of methanotrophic capacity in aerobic zones, such as the lack of a suitable physical model for accurate estimation. Hence, the authors conjectured that aerobic methanotrophs are responsible for 62% (average of 28–96% in the Amazon basin103) of methane oxidized in the river water above the sediment on a global scale. In this case, if the median capacity potentials of methanotrophs were realized, AOM and aerobic pathways can reduce approximately 3% and 64% of the methane released from the sandy sediment globally (Table 1 and Fig. 8).
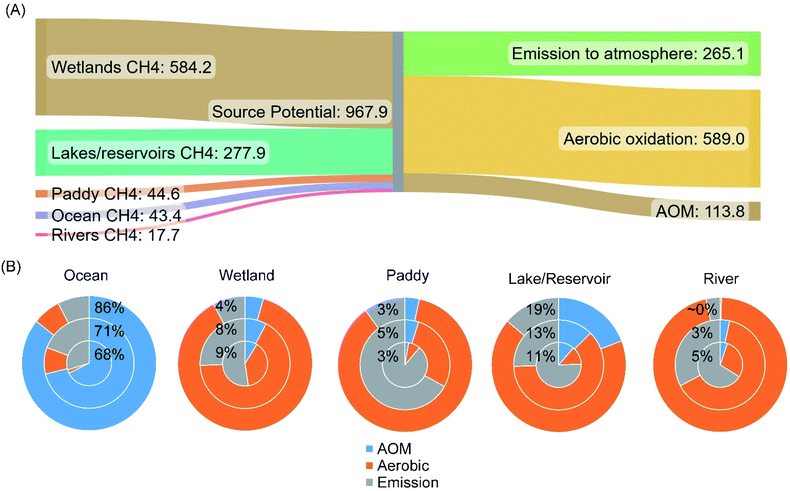 |
| Fig. 8 Estimated methane emission potentials (aerobically and anoxically oxidized + efflux to the atmosphere) from the studied methane sources and the approximate methane budgets in TgCH4 per year (A). Estimated medians as shown in Table 1 were plotted. Panel (B) shows the relative proportions of methane consumed by anoxic (labeled with percentages) and aerobic oxidation in estimated methane release potential from individual natural ecosystems. The inner circles, middle circles, and outer circles sequentially show the results calculated with 25% percentile, median, and 75% percentile aerobic/anoxic methane oxidation rates, respectively. Each level was paired with the corresponding level of methane efflux to the atmosphere. | |
Overall, in freshwater systems, the fractional turnover per unit time, K (per day), of aerobic and anoxic methanotrophs was not significantly different (Mann–Whitney U-test, p = 0.85, Fig. 6B). Although the median AOM rate (0.0048 nmolCH4 g−1 d−1) in freshwater is of the same order of magnitude as these of the wetlands (0.0034 nmolCH4 cm−3 d−1) and paddy fields (0.0018 nmolCH4 g−1 d−1), the median K value of AOM in freshwater (0.00016 per day) is two orders of magnitude lower than their counterparts in wetlands and paddy fields (Fig. 4B and 5B). Since DAMO is usually the dominant AOM pathway in freshwater, wetlands, and paddy fields, and the K value is assumed to be scaled with the population size of AOM microorganisms,82 the generally smaller K value in freshwater suggests that the DAMO microorganisms are not as abundant as these in wetlands and paddy fields.
2.1.4 Terrestrial mud volcanoes, hot springs, and natural gas sites.
AOM activity has also been detected in some other terrestrial environments, such as mud volcanoes, hot springs, and natural gas sites, where both methane and suitable electron acceptors are available. The microorganisms active in AOM were found to be not limited to DAMO microorganisms. For example, Methanoperedenaceae (5.7% in total archaea), ANME-3 (0.6%), ANME-2a/b (0.5%), and ANME-1 (0.3%) were identified as the main anoxic methanotrophs in a terrestrial mud volcano from the Bulganak mud volcano in Crimea.146 Furthermore, a recently enriched microorganism, named “Candidatus Methanodesulfokores washburnensis”, was found to carry genes for both methane oxidation and sulfur reduction in the thermal sediments from Washburn Hot Springs.19 In addition, sulfate-driven AOM has been detected in freshwater natural gas sources with ANME-2a/b and AOM-associated archaea as methane oxidizers.147 However, the rates (pmolCH4 cm−3 d−1 to nmolCH4 cm−3 d−1) are generally much lower than the rates compiled in previous sections or the rates were not reported.19,148,149 Since the research evidence of AOM in these terrestrial environments is still scarce, the reported AOM rates are incorporated in Fig. 3, but the AOM capacities in these environments are not calculated.
2.2 Marine environment
2.2.1 Overall significance of AOM in the marine environment.
The AOM activity has been shown to primarily occur in sediments,29 and the overall rates of AOM in marine sediments, including those driven by major reported electron acceptors (i.e., sulfate, metal oxides, and nitrate/nitrite), range from 5.1 × 10−8 to 7.0 × 104 nmolCH4 cm−3 d−1 (n = 387, median = 0.2 nmolCH4 cm−3 d−1, and rates obtained after prolonged incubation were excluded) (Fig. 7).150–167 Sulfate-AOM is the dominant methane oxidation pathway because sulfate is the major reactive anion in seawater. Recent data suggest that iron/manganese oxides are only responsible for approximately 2–3% of the methane oxidized around and below the sulfate–methane transition zones (SMTZ),165 where the sulfate-driven AOM is usually detected. Besides, within the SMTZ, sulfate-AOM is usually more significant than the background organoclastic sulfate reduction.168 Since the data regarding the depth of sediment harboring metal oxides and nitrate/nitrite that are actively involved in AOM are not as abundant as that for sulfate-AOM,154,169 and the coverage of these non-sulfate-dominant niches is also likely to be limited, as mentioned in Section 2.2.2, the estimation of the contribution of AOM in the marine environment is therefore based solely on sulfate-AOM in the sediment. It is thus obvious that the estimate will be conservative.
Note that the so-called cryptic sulfur cycle (i.e., sulfate-AOM and sulfide oxidation occur simultaneously in a confined space) is a special phenomenon because other electron acceptors, often metal oxides, are thought to be involved in the cycling of sulfur (i.e., sulfate replenishment via sulfide oxidation).131 For example, a study of marine sediment on the Alaskan Beaufort Sea continental margin indicates that AOM, involving the cryptic sulfur cycle, occurs below the SMTZ, and the rates were 2.4–8 nmolCH4 cm−3 d−1.170 Rates from this kind of reaction or suspected reaction were considered sulfate-AOM because the details of sulfur cycling reactions are often unclear.
Specifically, the reported rate of sulfate-AOM from the SMTZ ranges from 5.1 × 10−8 to 7.0 × 104 nmolCH4 cm−3 d−1 (n = 370 and median = 0.15 nmolCH4 cm−3 d−1).150,152,153,160,171–176 Based on the reported depth of the SMTZ in different regions and the corresponding area of each (Table 1),12,15,161,165,171,177 solely sulfate mediated AOM in the SMTZ is capable of reducing the methane emission by approximately 28 [Q1–Q3: 11–281] TgCH4 per year (Table 1). Additionally, the analysis of the AOM at simulated ocean bottom pressures with samples collected from various cold seep systems and coastal marine basins suggests that AOM is capable of oxidizing 3 [Q1–Q3: 0.4–39.6] TgCH4 per year (Table 1) in cold seep systems (refer to the ESI for details†). In total, AOM in the SMTZ and cold seep systems can reduce approximately 31 [11–321] TgCH4 per year of methane from the seabed. Although the estimation here is only a rough extrapolation based on the compiled reaction rates, this estimated median AOM capacity is close to the estimated average AOM capacity (45 TgCH4 per year) with a regression model using diffusive fluxes of methane to the SMTZ.15 Besides, the higher end of the current estimation is of the same order of magnitude as the most frequently cited global AOM estimate, which is 382 TgCH4 per year.15,34 As mentioned in the previous sections, since the AOM rates are mostly obtained in incubation studies under ideal laboratory conditions, the AOM capacity here should be treated as a reference value or more as an approximate number for the ability of methanotrophs in anaerobic and anoxic zones in the marine environment. The further validation of these estimations is currently difficult or even impossible because it is not clear whether the methanogenesis in marine sediment15,178 (approximately 92–101 TgCH4 per year) and the still unknown amount of methane released from seep systems179 can support such high sulfate-driven methanotrophic activity (i.e., a few hundred TgCH4 per year). Therefore, the estimated median value is used in the discussion in the following sections to reduce the uncertainties associated with the data extrapolation.
It is noteworthy that some of the seemingly low AOM rates may be subjected to modification in the future because some earlier AOM rates were obtained at ambient pressure, which is much lower than the in situ pressure. For instance, a substantial difference between measured AOM rates at ambient pressure and estimated/measured values from deep water sites or ocean bottoms has been reported.8,158,180 Specifically, the incubation of the Eckernförde Bay sediment at 10.1 MPa of methane atmosphere enriched ANME-2a/b/c and SRB, yielding an AOM rate of 0.046 μmolCO2 cm−3 d−1, a few times higher than that at ambient pressure.181 Similarly, incubations simulating the in situ pressure in the deep sea with sediments collected from a cold seep in the Gulf of Mexico and a hydrothermal site in the Guaymas Basin yielded 50 mM of dissolved methane, which supported AOM at 3.6–4.8 μmolCH4 cm−3 d−1.8 These results suggest that sulfate-AOM in the marine environment may be limited by methane.
Regarding marine aerobic methanotrophs, most of the rates are from the continental margins as well, ranging from 3.4 × 10−8 to 1.1 nmolCH4 cm−3 d−1 with a median of 9.1 × 10−5 nmolCH4 cm−3 d−1 (Q1–Q3: 4 × 10−6 to 2.5 × 10−4 nmolCH4 cm−3 d−1).83,153,171,182–194 Based on the rates of aerobic methane oxidation, the median depths of oxic surface seawater, 530 [Q1–Q3: 153–975] m, and the area of the coastal ocean and continental shelf (9% of total ocean area), aerobic methanotrophs solely in the water column of this region are capable of oxidizing 4 [Q1–Q3: 0.4–25] TgCH4 per year (Table 1). Alternatively, considering the pertinent estimates that (a) methane efflux from coastal and open sea ranges from 9 to 22 TgCH4 per year (mean: 13 TgCH4 per year),1 (b) coastal ocean accounts for approximately 75% of the global marine methane efflux to the atmosphere,195 and (c) aerobic methanotrophs in the coastal ocean water column can consume 1.2–19 times (median = 2.5) of methane as compared to methane efflux to the atmosphere,195 the aerobic methanotrophs in the coastal ocean water column oxidize 17 to 41 Tg per year (mean: 28 Tg per year) of methane. The results from both approaches suggest that aerobic methanotrophy is a significant methane sink. Besides, it is also speculated that aerobic methane oxidation also exists under the vast open sea because aerobic methanotrophic activity was detected in the oxygen minimum zone and the water column beneath (approximately 1000–2500 m under the sea surface).83,196,197 However, data from such areas are limited, as compared to the relatively well-studied continental margins. Therefore, more methane may be oxidized by aerobic methanotrophs.
Overall, the methane oxidation rate seems to be weakly correlated with the initial methane partial pressure during the incubation for rate quantification (R2 = 0.52–0.54, linear regression lines not shown, Fig. 7). This trend for AOM rates, albeit weak, is consonant with the observed increased methane oxidation rates at higher methane partial pressures.8,158,180 Regarding the fractional turnover per unit time, K (per day), those of aerobic (median = 0.0025 per day, Q1–Q3: 0.00035–0.022 per day) and anoxic methanotrophs (median = 0.00091 per day, Q1–Q3: 0.0003–0.011 per day) are not significantly different (Mann–Whitney U-test, p = 0.07, Fig. 7B). Comparable K values of aerobic and anaerobic methane oxidation in the marine environment have been observed previously,82 indicating that aerobic and anaerobic methanotrophs in the marine environment are similarly active if both methane oxidation rates follow first-order kinetics. If the estimated median capacity of AOM was realized, the AOM accounts for approximately 71% of the methane emission potential in the marine sediment, close to previously reported values, approximately 75–90%.198,199
2.2.2 AOM driven mainly by metal oxides.
Despite the prevalence of sulfate-AOM in marine sediment, other electron acceptors can also support AOM in marine environments. The concentrations of electron acceptors and the energy yields of the redox reactions eventually determine the distinct dominant oxidant profiles along the depth of marine sediment.200 Studies that report the presence of non-sulfate-based electron acceptors in marine AOM often show the decoupling of sulfate reduction and AOM.10,75,166,170,175,201 For example, the incubation of sediments from cold seeps and hydrothermal sites showed that the AOM rate is constantly higher than the sulfate-reduction rate when the methane concentration was 5–50 mmol L−1, suggesting that other electron acceptors participated in AOM.8 In another study, AOM by ANME-1d from deep Black seawater was enhanced when sodium molybdate was added, implying that sulfate reduction was not coupled to AOM.202
Among various non-sulfate electron acceptors, iron oxide is a frequently reported one. The AOM driven by metal oxides is generally faster than sulfate-AOM (Fig. 3). Recent studies demonstrated that iron oxides can induce AOM below the SMTZ where sulfate is less available or in places where iron does not precipitate as iron sulfide.165,203 For example, in the Helgoland Mud Area of the North Sea, incubation studies of sediments below the SMTZ indicate that iron oxide-driven AOM occurred at a rate of 0.095 ± 0.03 nmol cm−3 d−1.165 Additionally, in estuaries and coastal regions with a high inflow of iron, iron oxides can also serve as an electron acceptor.154,204 For instance, ferrihydrite-driven AOM has been reported in an incubation study of brackish coastal sediments from the Bothnian Sea, and the AOM reached 3.6 ± 0.2 nmol cm−3 d−1.205 In some other cases, non-sulfate-based electron acceptors become important due to the inactivation of SRB. For example, in metalliferous hydrothermal sediments where the temperature is high (e.g., 90 °C) and the activity of SRB was found to be greatly inhibited, ANME-1a may couple the reduction of ferric iron to AOM, reaching 0.152 μmolCH4 cm−3 d−1.166 Furthermore, ferric iron-driven AOM has also been proposed to explain the high dissolved ferrous iron concentrations in the pore water in some marine sediments, such as that in the Bothnian sea, an oligotrophic and low salinity coastal basin206 and the oligotrophic sediment from the southeastern Mediterranean continental shelf.207 Although some non-sulfate-based electron acceptors, such as metal oxides, are thermodynamically more favorable electron acceptors than sulfate and tend to compete with sulfate for methane, they do sometimes facilitate sulfate-AOM, such as in the case of the cryptic sulfur cycle.208,209
2.2.3 DAMO in estuaries and intertidal zones.
Coastal seas account for approximately 15% of the global ocean surface area but are responsible for approximately 75% of the marine methane efflux to the atmosphere.195 Research in the past decade indicates that non-sulfate-AOM exists in estuaries and intertidal zones.210,211 For instance, the incubation of sediments collected from various sites along the riverbank in the Yangtze Estuary in China showed active nitrite-DAMO at 0.2–84.3 nmolCO2 g−1 d−1 and nitrate-DAMO at 0.4–32.6 nmolCO2 g−1 d−1.74 The incubation and analysis of sediments from marine coastal ecosystems found that DAMO bacteria are active.22,163 These observations corroborated the previous results from the analysis of the 16S rRNA gene and pmoA sequences of DAMO bacteria, which indicate that their richest community is from marine and coastal environments.155 Seawater enriched subgroup A cells of the NC10 phylum showed an affinity coefficient of 9.8 ± 2.2 μmolCH4 L−1 and this value is in the range of measured affinity coefficients in freshwater systems,212 suggesting that the DAMO rates may be similar to those observed in terrestrial ecosystems. The DAMO activities by M. oxyfera-like and M. sinica-like bacteria detected in the Zhoushan islands intertidal zone yielded a similar AOM rate (nitrite-DAMO: 0.6–5.7 nmolCO2 g−1 d−1) to sulfate-AOM by the same sediment.213 Similarly, DAMO archaea detected in the intertidal zone also support a similar AOM rate (nitrate-DAMO: 0.16–1.49 nmolCO2 g−1 d−1) to sulfate-AOM.214 Temperature and nitrogen compound concentration were found to be the two most influential factors that affect the DAMO bacteria abundance.213 Comparable AOM rates were detected in another intertidal zone in the Chongming eastern intertidal flat in China, where DAMO bacteria and archaea showed methane oxidation rates of 0.1–39.9 nmolCO2 g−1 d−1 and 0.1–46.7 nmolCO2 g−1 d−1, respectively.162,215 Similar to sulfate-AOM, the rate of DAMO tends to increase after a long term of cultivation,212 but AOM rates from such systems were excluded from this review.
2.2.4 Sulfate-AOM in marine mud volcanoes and hydrothermal vents.
Several recent publications also reported AOM, nmolCH4 cm−3 d−1 to a few μmolCH4 cm−3 d−1, at marine mud volcanoes and hydrothermal vents,167,171,216,217 which have been recognized as the major geological formations that release methane to the hydrosphere and atmosphere.218 ANME-1, ANME-2, and/or ANME-3 have been reported to be responsible for the observed AOM in these environments.167,217 For example, ANME-1 and ANME-2 were detected in mud volcano deposits in Ginsburg MV,167 while ANME-2c and ANME-3 were found to participate in sulfate-AOM in mud volcanoes in the Canadian Beaufort Sea.219 Besides, the cultivation of the sediments (854 m below the water level) from the mud volcano Peschanka enriched ANME-2 and bacteria belonging to the NC10 phylum,220 implying that AOM may be active in this sediment. Incubation studies showed that the AOM in hydrothermal vents is usually temperature-dependent, and AOM activity persists in a wide range of temperatures up to 90 °C.166,217 However, with limited information on geographical coverage and reaction rates, these AOM rates at elevated temperatures are not considered during the AOM capacity estimation.
3. Some of the possibly highest AOM rates
The publications on nitrite/nitrate-DAMO raised the interest in engineering this AOM pathway for denitrification, which is currently a main nutrient removal step in the biological wastewater treatment process.47,48,221 Besides, DAMO may also be applied for methane emission reduction from ruminant livestock,222 which accounts for almost one-third of total anthropogenic methane emissions.1 DAMO (nitrogen mainly as NO2−/NO3−) and DAMO coupled with anaerobic ammonium oxidation (DAMO-Anammox, NO2−/NO3− together with a significant concentration of NH4+) are two of the commonly studied AOM pathways for denitrification. The sources of inoculum for these studies include paddy soils, freshwater sediments, wastewater, and marine sediments.21,53,223,224 Overall, with an enriched microbial consortium in a pure or close-to-pure methane atmosphere (usually balanced with 5% CO2), lab-scale reactors generally demonstrated much higher unit-volume-based AOM rates than those measured with the original inocula. Hence, the AOM rates obtained with bioreactors are compiled here as references for the maximum possible AOM rates (Fig. 3), with the caveat that these high rates may not exist in nature.
Specifically, nitrite-based DAMO in bioreactors usually shows methane oxidation at 0.00004–22.31 μmolCH4 cm−3 d−1,22,49,53,62,63,98,110,224–229 often higher than nitrite-DAMO observed in terrestrial and marine environments. Besides, unlike sulfate-AOM, it seems that the nitrite-DAMO is not limited by methane.230 For systems with both nitrate-DAMO and nitrite-DAMO, similar AOM rates of 3 × 10−6 to 11.5 μmolCH4 cm−3 d−1 (ref. 28, 50, 53, 92, 122 and 231–234) were reported. Currently, the highest AOM rate observed in bioreactors with nitrogen removal as the objective was from coculturing of DAMO archaea and Anammox bacteria.28,45,46,235 Overall, the methane consumption rate of DAMO-Anammox ranges from 0.078 to 138 μmol cm−3 d−1,23,24,33,45,46,50,235–239 and it seems that dissolved methane is not a limiting factor during DAMO-Anammox as well because elevated methane partial pressure had little effect on the activity of DAMO microorganisms.51
4. Factors that influence the AOM rates and issues in rate measurement
4.1 Factors that influence the AOM rates
The AOM rate in a studied environment can be reasonably estimated according to the measured biogeochemical parameters and the concentration-depth profiles of major reactants.4,31,83 The AOM rate reflects the activities of local microbiota, which are under the control of multiple environmental factors. In addition to the availability of methane and a suitable electron acceptor, other environmental factors, such as temperature,97,216 pressure,171,181,216,240 accessibility of essential metal ions,241 the turnover rate of electron acceptors,200 organic content,172,175etc., have all been documented to affect the AOM rate. It seems that no straightforward model can explain all the observations across different environments due to the different concentrations/levels of these above factors. In a natural ecosystem, the effect of one factor can often be compounded with the effects of other factors. For example, the incubation of slurries of the sediments from rivers with sandy riverbeds suggests that the AOM microbes were limited by electron acceptors because only the addition of both methane and the electron acceptors, nitrite for DAMO bacteria and nitrate for DAMO archaea, significantly stimulated the growth of the respective microorganisms.31 While in a study of the AOM rate of coastal freshwater and brackish wetland sediments with dissolved methane close to saturation, the added electron acceptors mostly inhibited AOM, as opposed to boosting the activity,242 and the results, together with the increased utilization of added electron acceptors, suggest that organic matter contained in the sediments was oxidized instead of methane. Thus, unknown heterotrophic microorganisms competed with methanotrophs for the electron acceptors, and methanogenesis might have also been inhibited in the presence of excessive electron acceptors, leading to limited methane supply for AOM and the eventually decreased methane oxidation.
A potential limiting factor reported in many of the cited references is the commonly encountered slow growth of the methanotrophs. Specifically, the doubling time for ANME, including ANME-1 and ANME-2a/c, from marine sediment ranges from 33–225 days according to 15N and 13CO2 assimilation tests.10,201,243 The doubling time of the freshwater bacterium M. oxyfera from the NC10 phylum was estimated to be around 7 to 14 days,2 while the marine NC10 bacteria showed a longer doubling time of 38.7 to 48.9 days.244 Compared to the NC10 phylum bacteria, the DAMO archaea grow faster.92 The doubling times for DAMO microorganisms are close to the reported values of Anammox bacteria, 18–46 days (29 °C) and 24–79 days (down to 12.5 °C),245 although some highly active Anammox bacteria showed a much shorter doubling time (around 2 days).246 The generally long doubling times explain why AOM microorganisms require months or years to be enriched247 and the low biomass can limit the overall rate of AOM.
Carbon assimilation, one aspect of cell growth, seems to be a reason behind the slow growth of anaerobic methanotrophs. One previously observed methanotrophic consortium for sulfate-AOM showed over 20% of 13C (from 13CH4) incorporation by solely archaea,248 while another showed less than 10% incorporation by the whole methanotrophic consortium:8 the AOM rate of the former (286 μmol cm−3 d−1) is over 500 times that of the latter (approximately 0.5 μmol cm−3 d−1), indicating that a higher rate of organic carbon assimilation is a key determinant of the AOM rate. Nevertheless, many studies reported that ANME prefers the energy-consuming pathways involving inorganic carbon reduction and incorporation. For ANME, the assimilated carbon (e.g., 96% (ref. 10)) can be from bicarbonate as opposed to carbon from methane. In a study of AOM communities enriched from the Guaymas Basin and the Elba seep, methane-derived carbon only accounted for 3–15% of the total biomass, in which ANME-1 or ANME-2 was the dominant methanotroph.249 It is conceivable that the preferred assimilation of bicarbonate over reduced carbon from methane limited the growth of these methanotrophs.
From the perspective of methane utilization, the low solubility of methane at ambient pressure and the high activation energy (439 kJ mol−1 CH4) required to break the first C–H bond in the methane molecule by methyl coenzyme M reductase have been thought to partly explain the generally slow AOM rate.250,251 However, as shown in the previous sections, higher dissolved methane concentrations at higher partial methane pressure do not always lead to increased methane oxidation. Besides, the dissociation of the C–O bond in acetate by coenzyme A is in a similar range, 452 kJ mol−1 acetate,252 but acetate can be utilized by microorganisms at a much faster rate than methane. Thus, these factors alone cannot explain the low AOM rates. The list of references in this review is not exhaustive; however, the compiled AOM rates indicate that the median AOM rates (less influenced by the extreme values) in wetlands, paddy fields, and freshwater systems are comparable (Fig. 3A, Mood's median test, p = 0.395). The median AOM rates from these environments are statistically higher than that of the marine environment (Fig. 3A, Mood's median test, p < 0.005) in which sulfate-AOM is the main AOM pathway. Since the dominant methanotrophs in the studied freshwater, wetlands, and paddy fields are often DAMO microorganisms, this contrast in AOM rates may be partly attributed to the complicated syntrophic interactions between the methanotrophic archaea and SRB in the marine environment.35,253 Hence, it is likely that other environmental factors are secondary to the microorganisms and the AOM pathway in determining the overall rate.
4.2 Some issues in the rate measurement
In contrast to the more standardized methods for methane efflux measurement (e.g., static chamber, floating chamber, and core incubation) and modeling55 or even direct methane emission monitoring via a satellite-based survey,254 microbiological methane oxidation rates usually are obtained from incubation studies and multiple rate quantification methods are available. Therefore, caution must be taken that the spatiotemporal variance of the methane oxidation rates collected from both natural and anthropogenic environments, the overestimated, and sometimes underestimated oxidation rates may introduce uncertainties to the AOM capacity estimations in Section 2.
The techniques applied and the quality control of measurement are two of the crucial factors for accurate rate measurement. In addition to the lack of carbon assimilation information as mentioned in Section 2, some common issues are listed here as potential sources of uncertainties as well as opportunities for future research. First, likely, some of the methane oxidation rates measured in laboratory conditions may not represent the in situ methane oxidation rate. The common use of well-mixed slurries or microcosms with excessive liquid medium might disturb the original physical structures of the soil/sediments and influence the microbiological community. In these cases, the differences in terms of the availability of methane and the transport of the other nutrient and ions to and from the microbial cells may cause discrepancies between these estimated rates and the in situ rates. For example, during batch cultivation, it was demonstrated that shaking of bottles during incubation can lead to inaccurate estimation of either AOM or aerobic methane oxidation rates.86,255 Besides, as shown in Section 2, AOM rates in some environments may be methane-limited due to the low methane partial pressure, while the often higher methane partial pressure in lab-scale microcosms often tends to yield a higher AOM rate during incubation. Therefore, the determination of in situ methane concentration or partial pressure is necessary to adjust the measured rate. On the other hand, some studies of AOM in marine sediment suggest that the AOM rates measured at ambient pressure may have greatly underestimated the in situ AOM rates. As mentioned in Section 2.2.1, there are a few of studies in which increased methane partial pressure induced higher AOM rates. Another obvious issue is the reporting of AOM rates. Most studies normalized the rates by the mass of dried sediment/soil or the volume of either the enrichment slurry or the cell suspension, although the normalization of rates by biomass is better for the evaluation of the actual AOM activity.
5. Conclusions and potential directions for future research
Methane is the most abundant hydrocarbon in the atmosphere and important greenhouse gas. Current biogeochemical models and analyses of methane sources and sinks often consider only aerobic methane oxidation.256–258 Here, the estimated significance of AOM in various methane sources indicates that it is necessary to incorporate AOM in future methane budget analysis. Based on the estimated median methane oxidation capacities, AOM can reduce approximately 3–71% of the methane entering the anoxic and anaerobic zones in the studied environments (Fig. 8). For example, approximately 68–86% of the generated methane in oceans can be oxidized anoxically. In contrast, AOM is generally secondary to aerobic methane oxidation in the other studied environments, including wetlands, paddy systems, lakes/reservoirs, and rivers. The back-calculated median methane emission potentials (i.e., total oxidized plus efflux to the atmosphere) suggest that approximately 72.6% of the methane from the studied sources is oxidized by methanotrophs before entering the atmosphere, and AOM accounts for 11.7% in the total source potential. Meanwhile, it has to be acknowledged that the insufficient information on the geographic coverage of each ecosystem as well as the spatiotemporal variation in methanotrophic activities1,15,55,135 all contribute to the wide range of estimated AOM capacities. Therefore, to assist in adjusting the total strength of methane sources and in improving the accuracy of the output from various biogeochemical models, which eventually would lead to a better forecast of methane emission potentials in various environments, more systematic research of the AOM in anaerobic and anoxic zones is warranted.
Moreover, during the 2008–2017 decade, approximately 60% of atmospheric methane is attributed to anthropogenic sources.1 According to the assessment published by the United Nations Environment Programme, reducing methane emissions from human activities is one of the most cost-effective strategies to reduce the rate of global warming.259 The study of various AOM pathways in nature not only reforms our understanding of this methane sink, but the knowledge gained also expands our repertoire of methane control strategies. With the discovery of AOM activities in more natural environments and a better understanding of the control factors in regulating the rates of AOM, AOM may be engineered shortly as a tool for mitigating methane emission from both human activities and natural environments.
Abbreviations
AOM | Anaerobic oxidation of methane |
ANME | Anaerobic methanotrophs |
Anammox | Anaerobic ammonium oxidation |
DAMO | Denitrifying anaerobic methane oxidation |
SMTZ | Sulfate–methane transition zone |
SRB | Sulfate-reducing bacteria |
Author contributions
Yaohuan Gao: conceptualization, methodology, data curation, visualization, writing, and editing; Yong Wang: data curation; Hyung-Sool Lee: reviewing; Pengkang Jin: reviewing and editing.
Conflicts of interest
There are no conflicts to declare.
Acknowledgements
We would like to thank all anonymous reviewers for their valuable suggestions. This work was supported by the Fundamental Research Funds for the Central Universities in China [XXJ032020003] and the Natural Science Foundation of Shaanxi Province [2021JQ-053].
References
- M. Saunois, A. R. Stavert, B. Poulter, P. Bousquet, J. G. Canadell, R. B. Jackson, P. A. Raymond, E. J. Dlugokencky, S. Houweling, P. K. Patra, P. Ciais, V. K. Arora, D. Bastviken, P. Bergamaschi, D. R. Blake, G. Brailsford, L. Bruhwiler, K. M. Carlson, M. Carrol, S. Castaldi, N. Chandra, C. Crevoisier, P. M. Crill, K. Covey, C. L. Curry, G. Etiope, C. Frankenberg, N. Gedney, M. I. Hegglin, L. Höglund-Isaksson, G. Hugelius, M. Ishizawa, A. Ito, G. Janssens-Maenhout, K. M. Jensen, F. Joos, T. Kleinen, P. B. Krummel, R. L. Langenfeld, G. G. Laruelle, L. Liu, T. Machida, S. Maksyutov, K. C. McDonald, J. McNorton, P. A. Miller, J. R. Melton, I. Morino, J. Müller, F. Murguia-Flores, V. Naik, Y. Niwa, S. Noce, S. O'Doherty, R. J. Parker, C. Peng, S. Peng, G. P. Peters, C. Prigent, R. Prinn, M. Ramonet, P. Regnier, W. J. Riley, J. A. Rosentreter, A. Seger, I. J. Simpson, H. Shi, S. J. Smith, L. P. Steel, B. F. Thornton, H. Tian, Y. Tohjima, F. N. Tubille, A. Tsuruta, N. Viovy, A. Voulgarakis, T. S. Weber, M. van Weele, G. R. van der Werf, R. F. Weiss, D. Worthy, D. Wunch, Y. Yin, Y. Yoshida, W. Zhang, Z. Zhang, Y. Zhao, B. Zheng, Q. Zhu, Q. Zhu and Q. Zhuang, The Global Methane Budget 2000–2017, Earth Syst. Sci. Data, 2020, 12, 1561–1623 CrossRef.
- K. F. Ettwig, M. K. Butler, D. Le Paslier, E. Pelletier, S. Mangenot, M. M. M. Kuypers, F. Schreiber, B. E. Dutilh, J. Zedelius, D. De Beer, J. Gloerich, H. J. C. T. Wessels, T. Van Alen, F. Luesken, M. L. Wu, K. T. Van De Pas-Schoonen, H. J. M. Op Den Camp, E. M. Janssen-Megens, K. J. Francoijs, H. Stunnenberg, J. Weissenbach, M. S. M. Jetten and M. Strous, Nitrite-driven anaerobic methane oxidation by oxygenic bacteria, Nature, 2010, 464, 543–548 CrossRef CAS PubMed.
- S. J. Blazewicz, D. G. Petersen, M. P. Waldrop and M. K. Firestone, Anaerobic oxidation of methane in tropical and boreal soils: Ecological significance in terrestrial methane cycling, J. Geophys. Res.: Biogeosci., 2012, 117, 1–9 Search PubMed.
- K. E. A. Segarra, F. Schubotz, V. Samarkin, M. Y. Yoshinaga, K. U. Hinrichs and S. B. Joye, High rates of anaerobic methane oxidation in freshwater wetlands reduce potential atmospheric methane emissions, Nat. Commun., 2015, 6, 7477 CrossRef CAS PubMed.
- L.-d. Shen, H.-s. Wu and Z.-q. Gao, Distribution and environmental significance of nitrite-dependent anaerobic methane-oxidising bacteria in natural ecosystems, Appl. Microbiol. Biotechnol., 2015, 99, 133–142 CrossRef CAS PubMed.
- K. Martinez-Cruz, A. Sepulveda-Jauregui, P. Casper, K. W. Anthony, K. A. Smemo and F. Thalasso, Ubiquitous
and significant anaerobic oxidation of methane in freshwater lake sediments, Water Res., 2018, 144, 332–340 CrossRef CAS PubMed.
- S. Guerrero-Cruz, A. Vaksmaa, M. A. Horn, H. Niemann, M. Pijuan and A. Ho, Methanotrophs: Discoveries, Environmental Relevance, and a Perspective on Current and Future Applications, Front. Microbiol., 2021, 12, 678057 CrossRef PubMed.
- M. W. Bowles, V. A. Samarkin, K. S. Hunter, N. Finke, A. P. Teske, P. R. Girguis and S. B. Joye, Remarkable Capacity for Anaerobic Oxidation of Methane at High Methane Concentration, Geophys. Res. Lett., 2019, 46, 12192–12201 CrossRef CAS.
- M. Cui, A. Ma, H. Qi, X. Zhuang and G. Zhuang, Anaerobic oxidation of methane: an “active” microbial process, MicrobiologyOpen, 2015, 4, 1–11 CrossRef CAS PubMed.
- J. Milucka, T. G. Ferdelman, L. Polerecky, D. Franzke, G. Wegener, M. Schmid, I. Lieberwirth, M. Wagner, F. Widdel and M. M. M. Kuypers, Zero-valent sulphur is a key intermediate in marine methane oxidation, Nature, 2012, 491, 541–546 CrossRef CAS PubMed.
- K. E. Miller, C.-T. Lai, R. A. Dahlgren and D. A. Lipson, Anaerobic Methane Oxidation in High-Arctic Alaskan Peatlands as a Significant Control on Net CH4 Fluxes, Soil Syst., 2019, 3, 7 CrossRef CAS.
- S. Bhattarai, C. Cassarini, G. Gonzalez-Gil, M. Egger, C. P. Slomp, Y. Zhang, G. Esposito and P. N. L. Lens, Anaerobic Methane-Oxidizing Microbial Community in a Coastal Marine Sediment: Anaerobic Methanotrophy Dominated by ANME-3, Microb. Ecol., 2017, 74, 608–622 CrossRef CAS PubMed.
- S. Islas-Lima, F. Thalasso and J. Gómez-Hernandez, Evidence of anoxic methane oxidation coupled to denitrification, Water Res., 2004, 38, 13–16 CrossRef CAS PubMed.
- R. K. Thauer, Anaerobic oxidation of methane with sulfate: on the reversibility of the reactions that are catalyzed by enzymes also involved in methanogenesis from CO2, Curr. Opin. Microbiol., 2011, 14, 292–299 CrossRef CAS PubMed.
- M. Egger, N. Riedinger, J. M. Mogollón and B. B. Jørgensen, Global diffusive fluxes of methane in marine sediments, Nat. Geosci., 2018, 11, 421–425 CrossRef CAS.
- J. F. Kleint, Y. Wellach, M. Schroll, F. Keppler and M. Isenbeck-Schröter, The impact of seasonal sulfate–methane transition zones on methane cycling in a sulfate-enriched freshwater environment, Limnol. Oceanogr., 2021, 66, 2290–2308 CrossRef CAS.
- K. Ino, A. W. Hernsdorf, U. Konno, M. Kouduka, K. Yanagawa, S. Kato, M. Sunamura, A. Hirota, Y. S. Togo, K. Ito, A. Fukuda, T. Iwatsuki, T. Mizuno, D. D. Komatsu, U. Tsunogai, T. Ishimura, Y. Amano, B. C. Thomas, J. F. Banfield and Y. Suzuki, Ecological and genomic profiling of anaerobic methane-oxidizing archaea in a deep granitic environment, ISME J., 2018, 12, 31–47 CrossRef CAS PubMed.
- M. Gauthier, R. L. Bradley and M. Šimek, More evidence that anaerobic oxidation of methane is prevalent in soils: Is it time to upgrade our biogeochemical models?, Soil Biol. Biochem., 2015, 80, 167–174 CrossRef CAS.
- L. J. McKay, M. Dlakić, M. W. Fields, T. O. Delmont, A. M. Eren, Z. J. Jay, K. B. Klingelsmith, D. B. Rusch and W. P. Inskeep, Co-occurring genomic capacity for anaerobic methane and dissimilatory sulfur metabolisms discovered in the Korarchaeota, Nat. Microbiol., 2019, 4, 614–622 CrossRef CAS PubMed.
- V. Gupta, K. A. Smemo, J. B. Yavitt, D. Fowle, B. Branfireun and N. Basiliko, Stable Isotopes Reveal Widespread Anaerobic Methane Oxidation Across Latitude and Peatland Type, Environ. Sci. Technol., 2013, 47, 8273–8279 CAS.
- Z. He, C. Cai, L. Shen, L. Lou, P. Zheng, X. Xu and B. Hu, Effect of inoculum sources on the enrichment of nitrite-dependent anaerobic methane-oxidizing bacteria, Appl. Microbiol. Biotechnol., 2015, 99, 939–946 CrossRef CAS PubMed.
- Z. He, S. Geng, C. Cai, S. Liu, Y. Liu, Y. Pan, L. Lou, P. Zheng, X. Xu, B. Hu and K. E. Wommack, Anaerobic Oxidation of Methane Coupled to Nitrite Reduction by Halophilic Marine NC10 Bacteria, Appl. Environ. Microbiol., 2015, 81, 5538–5545 CrossRef CAS PubMed.
- F. A. Luesken, J. Sánchez, T. A. van Alen, J. Sanabria, H. J. M. Op denCamp, M. S. M. Jetten and B. Kartal, Simultaneous nitrite-dependent anaerobic methane and ammonium oxidation processes, Appl. Environ. Microbiol., 2011, 77, 6802–6807 CrossRef CAS PubMed.
- L. Fu, J. Ding, Y.-Z. Lu, Z.-W. Ding and R. J. Zeng, Nitrogen source effects on the denitrifying anaerobic methane oxidation culture and anaerobic ammonium oxidation bacteria enrichment process, Appl. Microbiol. Biotechnol., 2017, 101, 3895–3906 CrossRef CAS PubMed.
- Z. He, C. Cai, J. Wang, X. Xu, P. Zheng, M. S. M. Jetten and B. Hu, A novel denitrifying methanotroph of the NC10 phylum and its microcolony, Sci. Rep., 2016, 6, 32241 CrossRef CAS PubMed.
- J. S. Graf, M. J. Mayr, H. K. Marchant, D. Tienken, P. F. Hach, A. Brand, C. J. Schubert, M. M. M. Kuypers and J. Milucka, Bloom of a denitrifying methanotroph, ‘Candidatus Methylomirabilis limnetica’, in a deep stratified lake, Environ. Microbiol., 2018, 20, 2598–2614 CrossRef CAS PubMed.
- W. Versantvoort, S. Guerrero-Cruz, D. R. Speth, J. Frank, L. Gambelli, G. Cremers, T. van Alen, M. S. M. Jetten, B. Kartal, H. J. M. Op den Camp and J. Reimann, Comparative Genomics of Candidatus Methylomirabilis Species and Description of Ca. Methylomirabilis Lanthanidiphila, Front. Microbiol., 2018, 9, 1672 CrossRef PubMed.
- M. F. Haroon, S. Hu, Y. Shi, M. Imelfort, J. Keller, P. Hugenholtz, Z. Yuan and G. W. Tyson, Anaerobic oxidation of methane coupled to nitrate reduction in a novel archaeal lineage, Nature, 2013, 500, 567–570 CrossRef CAS PubMed.
- K. Knittel and A. Boetius, Anaerobic oxidation of methane: Progress with an unknown process, Annu. Rev. Microbiol., 2009, 63, 311–334 CrossRef CAS PubMed.
- L. Shen, L. Ouyang, Y. Zhu and M. Trimmer, Spatial separation of anaerobic ammonium oxidation and nitrite-dependent anaerobic methane oxidation in permeable riverbeds, Environ. Microbiol., 2019, 21, 1185–1195 CrossRef CAS PubMed.
- L.-d. Shen, L. Ouyang, Y. Zhu and M. Trimmer, Active pathways of anaerobic methane oxidation across contrasting riverbeds, ISME J., 2019, 13, 752–766 CrossRef CAS PubMed.
- K. F. Ettwig, B. Zhu, D. Speth, J. T. Keltjens, M. S. M. Jetten and B. Kartal, Archaea catalyze iron-dependent anaerobic oxidation of methane, Proc. Natl. Acad. Sci. U. S. A., 2016, 113, 12792–12796 CrossRef CAS PubMed.
- C. Cai, S. Hu, J. Guo, Y. Shi, G.-J. Xie and Z. Yuan, Nitrate reduction by denitrifying anaerobic methane oxidizing microorganisms can reach a practically useful rate, Water Res., 2015, 87, 211–217 CrossRef CAS PubMed.
-
K. U. Hinrichs and A. Boetius, in Ocean Margin Systems, ed. G. Wefer, D. Billett, D. Hebbeln, B. B. Jørgensen, M. Schlüter and T. C. E. van Weering, Springer Berlin Heidelberg, Berlin, Heidelberg, 2003, pp. 457–477, DOI:10.1007/978-3-662-05127-6_28.
- C. Cai, X. Zhang, M. Wu, T. Liu, C.-Y. Lai, J. Frank, B. He, E. Marcellin, J. Guo, S. Hu and Z. Yuan, Roles and opportunities for microbial anaerobic oxidation of methane in natural and engineered systems, Energy Environ. Sci., 2021, 14, 4803–4830 RSC.
- C. U. Welte, O. Rasigraf, A. Vaksmaa, W. Versantvoort, A. Arshad, H. J. M. Op den Camp, M. S. M. Jetten, C. Lüke and J. Reimann, Nitrate- and nitrite-dependent anaerobic oxidation of methane, Environ. Microbiol. Rep., 2016, 8, 941–955 CrossRef CAS PubMed.
- D. L. Valentine and W. S. Reeburgh, New perspectives on anaerobic methane oxidation, Environ. Microbiol., 2000, 2, 477–484 CrossRef CAS PubMed.
- A. J. Wallenius, P. Dalcin Martins, C. P. Slomp and M. S. M. Jetten, Anthropogenic and Environmental Constraints on the Microbial Methane Cycle in Coastal Sediments, Front. Microbiol., 2021, 12, 678057 CrossRef PubMed.
- L.-D. Shi, Z. Wang, T. Liu, M. Wu, C.-Y. Lai, B. E. Rittmann, J. Guo and H.-P. Zhao, Making good use of methane to remove oxidized contaminants from wastewater, Water Res., 2021, 197, 117082 CrossRef CAS PubMed.
- X. Zhang, Z. Yuan and S. Hu, Anaerobic oxidation of methane mediated by microbial extracellular respiration, Environ. Microbiol. Rep., 2021, 13, 790–804 CrossRef CAS PubMed.
- H. Yang, S. Yu and H. Lu, Iron-Coupled Anaerobic Oxidation of Methane in Marine Sediments: A Review, J. Mar. Sci. Eng., 2021, 9, 875 CrossRef.
- Q. Zhao, Y. Wang, Z. Xu and Z. Yu, How does biochar amendment affect soil methane oxidation? A review, J. Soils Sediments, 2021, 21, 1575–1586 CrossRef CAS.
- J. Ding and R. J. Zeng, Fundamentals and potential environmental significance of denitrifying anaerobic methane oxidizing archaea, Sci. Total Environ., 2021, 757, 143928 CrossRef CAS PubMed.
- R. B. Costa, P. N. L. Lens and E. Foresti, Methanotrophic denitrification in wastewater treatment: microbial aspects and engineering strategies, Crit. Rev. Biotechnol., 2022, 42, 145–161 CrossRef CAS PubMed.
- Y. Shi, S. Hu, J. Lou, P. Lu, J. Keller and Z. Yuan, Nitrogen removal from wastewater by coupling anammox and methane-dependent denitrification in a membrane biofilm reactor, Environ. Sci. Technol., 2013, 47, 11577–11583 CrossRef CAS PubMed.
- Z.-W. Ding, J. Ding, L. Fu, F. Zhang and R. J. Zeng, Simultaneous enrichment of denitrifying methanotrophs and anammox bacteria, Appl. Microbiol. Biotechnol., 2014, 98, 10211–10221 CrossRef CAS PubMed.
- S.-Q. Fan, G.-J. Xie, Y. Lu, B.-F. Liu, D.-F. Xing, J. Ding, H.-J. Han and N.-Q. Ren, Nitrate/nitrite dependent anaerobic methane oxidation coupling with anammox in membrane biotrickling filter for nitrogen removal, Environ. Res., 2021, 193, 110533 CrossRef CAS PubMed.
- W.-B. Nie, J. Ding, G.-J. Xie, L. Yang, L. Peng, X. Tan, B.-F. Liu, D.-F. Xing, Z. Yuan and N.-Q. Ren, Anaerobic Oxidation of Methane Coupled with Dissimilatory Nitrate Reduction to Ammonium Fuels Anaerobic Ammonium Oxidation, Environ. Sci. Technol., 2021, 55, 1197–1208 CrossRef CAS PubMed.
- T. Liu, Z. K. Lim, H. Chen, Z. Wang, S. Hu, Z. Yuan and J. Guo, Biogas-driven complete nitrogen removal from wastewater generated in side-stream partial nitritation, Sci. Total Environ., 2020, 745, 141153 CrossRef CAS PubMed.
- W.-B. Nie, G.-J. Xie, J. Ding, L. Peng, Y. Lu, X. Tan, H. Yue, B.-F. Liu, D.-F. Xing, J. Meng, H.-J. Han and N.-Q. Ren, Operation strategies of n-DAMO and Anammox process based on microbial interactions for high rate nitrogen removal from landfill leachate, Environ. Int., 2020, 139, 105596 CrossRef CAS PubMed.
- C. Cai, S. Hu, X. Chen, B.-J. Ni, J. Pu and Z. Yuan, Effect of methane partial pressure on the performance of a membrane biofilm reactor coupling methane-dependent denitrification and anammox, Sci. Total Environ., 2018, 639, 278–285 CrossRef CAS PubMed.
- L. Fu, J. Ding, Y.-Z. Lu, Z.-W. Ding, Y.-N. Bai and R. J. Zeng, Hollow fiber membrane bioreactor affects microbial community and morphology of the DAMO and Anammox co-culture system, Bioresour. Technol., 2017, 232, 247–253 CrossRef CAS PubMed.
- M. Hatamoto, M. Kimura, T. Sato, M. Koizumi, M. Takahashi, S. Kawakami, N. Araki and T. Yamaguchi, Enrichment of denitrifying methane-oxidizing microorganisms using up-flow continuous reactors and batch cultures, PLoS One, 2014, 9, e115823 CrossRef PubMed.
- S. D. Bridgham, H. Cadillo-Quiroz, J. K. Keller and Q. Zhuang, Methane emissions from wetlands: biogeochemical, microbial, and modeling perspectives from local to global scales, Global Change Biol., 2013, 19, 1325–1346 CrossRef PubMed.
- J. A. Rosentreter, A. V. Borges, B. R. Deemer, M. A. Holgerson, S. Liu, C. Song, J. Melack, P. A. Raymond, C. M. Duarte, G. H. Allen, D. Olefeldt, B. Poulter, T. I. Battin and B. D. Eyre, Half of global methane emissions come from highly variable aquatic ecosystem sources, Nat. Geosci., 2021, 14, 225–230 CrossRef CAS.
- H. J. Laanbroek, Methane emission from natural wetlands: interplay between emergent macrophytes and soil microbial processes. A mini-review, Ann. Bot., 2010, 105, 141–153 CrossRef CAS PubMed.
- S. C. Whalen, Biogeochemistry of Methane Exchange between Natural Wetlands and the Atmosphere, Environ. Eng. Sci., 2005, 22, 73–94 CrossRef CAS.
- R. Segers, Methane production and methane consumption: a review of processes underlying wetland methane fluxes, Biogeochemistry, 1998, 41, 23–51 CrossRef CAS.
- Y. Mo, X.-e. Qi, A. Li, X. Zhang and Z. Jia, Active Methanotrophs in Suboxic Alpine Swamp Soils of the Qinghai–Tibetan Plateau, Front. Microbiol., 2020, 11, 580866 CrossRef PubMed.
- J. A. Amaral and R. Knowles, Methane Metabolism in a Temperate Swamp, Appl. Environ. Microbiol., 1994, 60, 3945–3951 CrossRef CAS PubMed.
- T. Roy Chowdhury, W. J. Mitsch and R. P. Dick, Seasonal methanotrophy across a hydrological gradient in a freshwater wetland, Ecol. Eng., 2014, 72, 116–124 CrossRef.
- B.-l. Hu, L.-d. Shen, X. Lian, Q. Zhu, S. Liu, Q. Huang, Z.-f. He, S. Geng, D.-q. Cheng, L.-p. Lou, X.-y. Xu, P. Zheng and Y.-f. He, Evidence for nitrite-dependent anaerobic methane oxidation as a previously overlooked microbial methane sink in wetlands, Proc. Natl. Acad. Sci. U. S. A., 2014, 111, 4495 CrossRef CAS PubMed.
- F. Xie, A. Ma, H. Zhou, Y. Liang, J. Yin, K. Ma, X. Zhuang and G. Zhuang, Niche differentiation of denitrifying anaerobic methane oxidizing bacteria and archaea leads to effective methane filtration in a Tibetan alpine wetland, Environ. Int., 2020, 140, 105764 CrossRef CAS PubMed.
- F. A. Luesken, B. Zhu, T. A. van Alen, M. K. Butler, M. R. Diaz, B. Song, H. J. M. Op den Camp, M. S. M. Jetten and K. F. Ettwig, pmoA primers for detection of anaerobic methanotrophs, Appl. Environ. Microbiol., 2011, 77, 3877–3880 CrossRef CAS PubMed.
- G. Zhu, L. Zhou, Y. Wang, S. Wang, J. Guo, X.-E. Long, X. Sun, B. Jiang, Q. Hou, M. S. M. Jetten and C. Yin, Biogeographical distribution of denitrifying anaerobic methane oxidizing bacteria in Chinese wetland ecosystems, Environ. Microbiol. Rep., 2015, 7, 128–138 CrossRef CAS PubMed.
- L.-d. Shen, Q. Huang, Z.-f. He, X. Lian, S. Liu, Y.-f. He, L.-p. Lou, X.-y. Xu, P. Zheng and B.-l. Hu, Vertical distribution of nitrite-dependent anaerobic methane-oxidising bacteria in natural freshwater wetland soils, Appl. Microbiol. Biotechnol., 2015, 99, 349–357 CrossRef CAS PubMed.
- Y. Zhang, X. Zhang, F. Wang, W. Xia and Z. Jia, Exogenous nitrogen addition inhibits sulfate-mediated anaerobic oxidation of methane in estuarine coastal sediments, Ecol. Eng., 2020, 158, 106021 CrossRef.
- K. E. A. Segarra, C. Comerford, J. Slaughter and S. B. Joye, Impact of electron acceptor availability on the anaerobic oxidation of methane in coastal freshwater and brackish wetland sediments, Geochim. Cosmochim. Acta, 2013, 115, 15–30 CrossRef CAS.
- H. S. Weber, K. S. Habicht and B. Thamdrup, Anaerobic Methanotrophic Archaea of the ANME-2d Cluster Are Active in a Low-sulfate, Iron-rich Freshwater Sediment, Front. Microbiol., 2017, 8, 619 Search PubMed.
- E. I. Valenzuela, A. Prieto-Davó, N. E. López-Lozano, A. Hernández-Eligio, L. Vega-Alvarado, K. Juárez, A. S. García-González, M. G. López and F. J. Cervantes, Anaerobic Methane Oxidation Driven by Microbial Reduction of Natural Organic Matter in a Tropical Wetland, Appl. Environ. Microbiol., 2017, 83 CrossRef CAS PubMed.
- E. I. Valenzuela, C. Padilla-Loma, N. Gómez-Hernández, N. E. López-Lozano, S. Casas-Flores and F. J. Cervantes, Humic Substances Mediate Anaerobic Methane Oxidation Linked to Nitrous Oxide Reduction in Wetland Sediments, Front. Microbiol., 2020, 11, 587 CrossRef PubMed.
- L.-D. Shi, P.-L. Lv, M. Wang, C.-Y. Lai and H.-P. Zhao, A mixed consortium of methanotrophic archaea and bacteria boosts methane-dependent selenate reduction, Sci. Total Environ., 2020, 732, 139310 CrossRef CAS PubMed.
- Y.-F. Wang, R. P. Dick, N. Lorenz and N. Lee, Interactions and responses of n-damo archaea, n-damo bacteria and anammox bacteria to various electron acceptors in natural and constructed wetland sediments, Int. Biodeterior. Biodegrad., 2019, 144, 104749 CrossRef CAS.
- F. Chen, Y. Zheng, L. Hou, Y. Niu, D. Gao, Z. An, J. Zhou, G. Yin, H. Dong, P. Han, X. Liang and M. Liu, Microbial abundance and activity of nitrite/nitrate-dependent anaerobic methane oxidizers in estuarine and intertidal wetlands: Heterogeneity and driving factors, Water Res., 2021, 190, 116737 CrossRef CAS PubMed.
- E. I. Valenzuela, K. A. Avendaño, N. Balagurusamy, S. Arriaga, C. Nieto-Delgado, F. Thalasso and F. J. Cervantes, Electron shuttling mediated by humic substances fuels anaerobic methane oxidation and carbon burial in wetland sediments, Sci. Total Environ., 2019, 650, 2674–2684 CrossRef CAS PubMed.
- F. Liu, Y. Zhang, H. Liang and D. Gao, Resilience of methane cycle and microbial functional genes to drought and flood in an alkaline wetland: A metagenomic analysis, Chemosphere, 2021, 265, 129034 CrossRef CAS PubMed.
- L.-d. Shen, S. Liu, Z.-f. He, X. Lian, Q. Huang, Y.-f. He, L.-p. Lou, X.-y. Xu, P. Zheng and B.-l. Hu, Depth-specific distribution and importance of nitrite-dependent anaerobic ammonium and methane-oxidising bacteria in an urban wetland, Soil Biol. Biochem., 2015, 83, 43–51 CrossRef CAS.
- S. Frei, K. H. Knorr, S. Peiffer and J. H. Fleckenstein, Surface micro-topography causes hot spots of biogeochemical activity in wetland systems: A virtual modeling experiment, J. Geophys. Res.: Biogeosci., 2012, 117, G00N12 Search PubMed.
- P. Roslev and G. M. King, Regulation of methane oxidation in a freshwater wetland by water table changes and anoxia, FEMS Microbiol. Ecol., 1996, 19, 105–115 CrossRef CAS.
- G. M. King, Dynamics and controls of methane oxidation in a Danish wetland sediment, FEMS Microbiol. Lett., 1990, 74, 309–323 CAS.
- B. Elberling, L. Askaer, C. J. Jørgensen, H. P. Joensen, M. Kühl, R. N. Glud and F. R. Lauritsen, Linking Soil O2, CO2, and CH4 Concentrations in a Wetland Soil: Implications for CO2 and CH4 Fluxes, Environ. Sci. Technol., 2011, 45, 3393–3399 CrossRef CAS PubMed.
- B. Thamdrup, H. G. R. Steinsdóttir, A. D. Bertagnolli, C. C. Padilla, N. V. Patin, E. Garcia-Robledo, L. A. Bristow and F. J. Stewart, Anaerobic methane oxidation is an important sink for methane in the ocean's largest oxygen minimum zone, Limnol. Oceanogr., 2019, 64, 2569–2585 CrossRef CAS.
- M. A. Pack, M. B. Heintz, W. S. Reeburgh, S. E. Trumbore, D. L. Valentine, X. Xu and E. R. M. Druffel, Methane oxidation in the eastern tropical North Pacific Ocean water column, J. Geophys. Res.: Biogeosci., 2015, 120, 1078–1092 CrossRef CAS.
- B. Zhu, G. van Dijk, C. Fritz, J. P. Smolders Alfons, A. Pol, S. M. Jetten Mike and F. Ettwig Katharina, Anaerobic Oxidization of Methane in a Minerotrophic Peatland: Enrichment of Nitrite-Dependent Methane-Oxidizing Bacteria, Appl. Environ. Microbiol., 2012, 78, 8657–8665 CrossRef CAS PubMed.
- L.-D. Shen, S. Liu, Q. Huang, X. Lian, Z.-F. He, S. Geng, R.-C. Jin, Y.-F. He, L.-P. Lou, X.-Y. Xu, P. Zheng and B.-L. Hu, Evidence for the cooccurrence of nitrite-dependent anaerobic ammonium and methane oxidation processes in a flooded paddy field, Appl. Environ. Microbiol., 2014, 80, 7611–7619 CrossRef PubMed.
- L. Fan, M. Shahbaz, T. Ge, J. Wu, M. Dippold, V. Thiel, Y. Kuzyakov and M. Dorodnikov, To shake or not to shake: 13C-based evidence on anaerobic methane oxidation in paddy soil, Soil Biol. Biochem., 2019, 133, 146–154 CrossRef CAS.
- A. S. K. Chan and T. B. Parkin, Methane Oxidation and Production Activity in Soils from Natural and Agricultural Ecosystems, J. Environ. Qual., 2001, 30, 1896–1903 CrossRef CAS PubMed.
-
R. Conrad, in Advances in Agronomy, Academic Press, 2007, vol. 96, pp. 1–63 Search PubMed.
- J. Ding, L. Fu, Z.-W. Ding, Y.-Z. Lu, S. H. Cheng and R. J. Zeng, Environmental evaluation of coexistence of denitrifying anaerobic methane-oxidizing archaea and bacteria in a paddy field, Appl. Microbiol. Biotechnol., 2016, 100, 439–446 CrossRef CAS PubMed.
- L. Zhou, Y. Wang, X.-E. Long, J. Guo and G. Zhu, High abundance and diversity of nitrite-dependent anaerobic methane-oxidizing bacteria in a paddy field profile, FEMS Microbiol. Lett., 2014, 360, 33–41 CrossRef CAS PubMed.
- L. Fan, D. Schneider, M. A. Dippold, A. Poehlein, W. Wu, H. Gui, T. Ge, J. Wu, V. Thiel, Y. Kuzyakov and M. Dorodnikov, Active metabolic pathways of anaerobic methane oxidation in paddy soils, Soil Biol. Biochem., 2021, 156, 108215 CrossRef CAS.
- A. Vaksmaa, S. Guerrero-Cruz, T. A. van Alen, G. Cremers, K. F. Ettwig, C. Lüke and M. S. M. Jetten, Enrichment of anaerobic nitrate-dependent methanotrophic ‘Candidatus Methanoperedens nitroreducens’ archaea from an Italian paddy field soil, Appl. Microbiol. Biotechnol., 2017, 101, 7075–7084 CrossRef CAS PubMed.
- A. Vaksmaa, C. Lüke, T. van Alen, G. Valè, E. Lupotto, M. S. M. Jetten and K. F. Ettwig, Distribution and activity of the anaerobic methanotrophic community in a nitrogen-fertilized
Italian paddy soil, FEMS Microbiol. Ecol., 2016, 92, fiw181 CrossRef PubMed.
- L. Fan, M. A. Dippold, T. Ge, J. Wu, V. Thiel, Y. Kuzyakov and M. Dorodnikov, Anaerobic oxidation of methane in paddy soil: Role of electron acceptors and fertilization in mitigating CH4 fluxes, Soil Biol. Biochem., 2020, 141, 107685 CrossRef CAS.
- Z. He, Y. Zhu, J. Feng, Q. Ji, X. Chen and X. Pan, Long-term effects of four environment-related iron minerals on microbial anaerobic oxidation of methane in paddy soil: A previously overlooked role of widespread goethite, Soil Biol. Biochem., 2021, 161, 108387 CrossRef CAS.
- Y. Zhang, F. Wang and Z. Jia, Electron shuttles facilitate anaerobic methane oxidation coupled to nitrous oxide reduction in paddy soil, Soil Biol. Biochem., 2021, 153, 108091 CrossRef CAS.
- L. Fan, M. A. Dippold, V. Thiel, T. Ge, J. Wu, Y. Kuzyakov and M. Dorodnikov, Temperature sensitivity of anaerobic methane oxidation versus methanogenesis in paddy soil: Implications for the CH4 balance under global warming, Global Change Biol., 2022, 28, 654–664 CrossRef CAS PubMed.
- Z. Hu and R. Ma, Distribution and characteristic of nitrite-dependent anaerobic methane oxidation bacteria by comparative analysis of wastewater treatment plants and agriculture fields in northern China, PeerJ, 2016, 4, e2766 CrossRef PubMed.
- Y. Zhang, F. Wang, W. Xia, W. Cao and Z. Jia, Anaerobic methane oxidation sustains soil organic carbon accumulation, Appl. Soil Ecol., 2021, 167, 104021 CrossRef.
- A. Reim, C. Lüke, S. Krause, J. Pratscher and P. Frenzel, One millimetre makes the difference: high-resolution analysis of methane-oxidizing bacteria and their specific activity at the oxic–anoxic interface in a flooded paddy soil, ISME J., 2012, 6, 2128–2139 CrossRef CAS PubMed.
- W. Liesack, S. Schnell and N. P. Revsbech, Microbiology of flooded rice paddies, FEMS Microbiol. Rev., 2000, 24, 625–645 CrossRef CAS PubMed.
- S. M. Haefele, A. Nelson and R. J. Hijmans, Soil quality and constraints in global rice production, Geoderma, 2014, 235–236, 250–259 CrossRef CAS.
- H. O. Sawakuchi, D. Bastviken, A. O. Sawakuchi, N. D. Ward, C. D. Borges, S. M. Tsai, J. E. Richey, M. V. R. Ballester and A. V. Krusche, Oxidative mitigation of aquatic methane emissions in large Amazonian rivers, Global Change Biol., 2016, 22, 1075–1085 CrossRef PubMed.
- P. K. Zigah, K. Oswald, A. Brand, C. Dinkel, B. Wehrli and C. J. Schubert, Methane oxidation pathways and associated methanotrophic communities in the water column of a tropical lake, Limnol. Oceanogr., 2015, 60, 553–572 CrossRef.
- D. Bastviken, J. Cole, M. Pace and L. Tranvik, Methane emissions from lakes: Dependence of lake characteristics, two regional assessments, and a global estimate, Global Biogeochem. Cycles, 2004, 18 CrossRef CAS.
- Y. Yang, J. Chen, B. Li, Y. Liu and S. Xie, Anaerobic methane oxidation potential and bacteria in freshwater lakes: Seasonal changes and the influence of trophic status, Syst. Appl. Microbiol., 2018, 41, 650–657 CrossRef CAS PubMed.
- J. S. Deutzmann, P. Stief, J. Brandes and B. Schink, Anaerobic methane oxidation coupled to denitrification is the dominant methane sink in a deep lake, Proc. Natl. Acad. Sci. U. S. A., 2014, 111, 18273 CrossRef CAS PubMed.
- G. Su, J. Zopfi, H. Yao, L. Steinle, H. Niemann and M. F. Lehmann, Manganese/iron-supported sulfate-dependent anaerobic oxidation of methane by archaea in lake sediments, Limnol. Oceanogr., 2020, 65, 863–875 CrossRef CAS.
- A. O. Leu, C. Cai, S. J. McIlroy, G. Southam, V. J. Orphan, Z.-G. Yuan, S.-H. Hu and G. W. Tyson, Anaerobic methane oxidation coupled to manganese reduction by members of the Methanoperedenaceae, ISME J., 2020, 14, 1030–1041 CrossRef CAS PubMed.
- L. Cabrol, F. Thalasso, L. Gandois, A. Sepulveda-Jauregui, K. Martinez-Cruz, R. Teisserenc, N. Tananaev, A. Tveit, M. M. Svenning and M. Barret, Anaerobic oxidation of methane and associated microbiome in anoxic water of Northwestern Siberian lakes, Sci. Total Environ., 2020, 736, 139588 CrossRef CAS PubMed.
- A. Sturm, D. A. Fowle, C. Jones, K. Leslie, S. Nomosatryo, C. Henny, D. E. Canfield and S. A. Crowe, Rates and pathways of CH4 oxidation in ferruginous Lake Matano, Indonesia, Geobiology, 2019, 17, 294–307 CrossRef CAS PubMed.
- Y. Long, Q. Guo, N. Li, B. Li, T. Tong and S. Xie, Spatial change of reservoir nitrite-dependent methane-oxidizing microorganisms, Ann. Microbiol., 2017, 67, 165–174 CrossRef CAS.
- A. Lomakina, T. Pogodaeva, G. Kalmychkov, S. Chernitsyna and T. Zemskaya, Diversity of NC10 Bacteria and ANME-2d Archaea in Sediments of Fault Zones at Lake Baikal, Diversity, 2020, 12, 10 CrossRef CAS.
- D. Szal and R. Gruca-Rokosz, Denitrification-Dependent Anaerobic Oxidation of Methane in Freshwater Sediments of Reservoirs in SE Poland, J. Ecol. Eng., 2019, 20, 218–227 CrossRef.
- D. Szal and R. Gruca-Rokosz, Anaerobic Oxidation of Methane in Freshwater Sediments of Rzeszów Reservoir, Water, 2020, 12, 398 CrossRef CAS.
- L.-d. Shen, M.-h. Tian, H.-x. Cheng, X. Liu, Y.-l. Yang, J.-q. Liu, J.-b. Xu, Y. Kong, J.-h. Li and Y. Liu, Different responses of nitrite- and nitrate-dependent anaerobic methanotrophs to increasing nitrogen loading in a freshwater reservoir, Environ. Pollut., 2020, 263, 114623 CrossRef CAS PubMed.
- F. Guérin and G. Abril, Significance of pelagic aerobic methane oxidation in the methane and carbon budget of a tropical reservoir, J. Geophys. Res.: Biogeosci., 2007, 112, G03006 Search PubMed.
- K. Martinez-Cruz, A. Sepulveda-Jauregui, K. Walter Anthony and F. Thalasso, Geographic and seasonal variation of dissolved methane and aerobic methane oxidation in Alaskan lakes, Biogeosciences, 2015, 12, 4595–4606 CrossRef.
- F. A. E. Roland, C. Morana, F. Darchambeau, S. A. Crowe, B. Thamdrup, J.-P. Descy and A. V. Borges, Anaerobic methane oxidation and aerobic methane production in an east African great lake (Lake Kivu), J. Great Lakes Res., 2018, 44, 1183–1193 CrossRef CAS.
- S. Carini, N. Bano, G. LeCleir and S. B. Joye, Aerobic methane oxidation and methanotroph community composition during seasonal stratification in Mono Lake, California (USA), Environ. Microbiol., 2005, 7, 1127–1138 CrossRef CAS PubMed.
- A. J. Rissanen, A. Karvinen, H. Nykänen, S. Peura, M. Tiirola, A. Mäki and P. Kankaala, Effects of alternative electron acceptors on the activity and community structure of methane-producing and consuming microbes in the sediments of two shallow boreal lakes, FEMS Microbiol. Ecol., 2017, 93, fix078 CrossRef PubMed.
- A. S. Bhattacharjee, A. M. Motlagh, M. S. M. Jetten and R. Goel, Methane dependent denitrification- from ecosystem to laboratory-scale enrichment for engineering applications, Water Res., 2016, 99, 244–252 CrossRef CAS PubMed.
- K. Martinez-Cruz, M.-C. Leewis, I. C. Herriott, A. Sepulveda-Jauregui, K. W. Anthony, F. Thalasso and M. B. Leigh, Anaerobic oxidation of methane by aerobic methanotrophs in sub-Arctic lake sediments, Sci. Total Environ., 2017, 607–608, 23–31 CrossRef CAS PubMed.
- O. Sivan, M. Adler, A. Pearson, F. Gelman, I. Bar-Or, S. G. John and W. Eckert, Geochemical evidence for iron-mediated anaerobic oxidation of methane, Limnol. Oceanogr., 2011, 56, 1536–1544 CrossRef CAS.
- I. Bar-Or, M. Elvert, W. Eckert, A. Kushmaro, H. Vigderovich, Q. Zhu, E. Ben-Dov and O. Sivan, Iron-Coupled Anaerobic Oxidation of Methane Performed by a Mixed Bacterial-Archaeal Community Based on Poorly Reactive Minerals, Environ. Sci. Technol., 2017, 51, 12293–12301 CrossRef CAS PubMed.
- T. J. Lawton and A. C. Rosenzweig, Methane-Oxidizing Enzymes: An Upstream Problem in Biological Gas-to-Liquids Conversion, J. Am. Chem. Soc., 2016, 138, 9327–9340 CrossRef CAS PubMed.
- S. van Grinsven, J. S. Sinninghe Damsté, A. Abdala Asbun, J. C. Engelmann, J. Harrison and L. Villanueva, Methane oxidation in anoxic lake water stimulated by nitrate and sulfate addition, Environ. Microbiol., 2020, 22, 766–782 CrossRef CAS PubMed.
- B. B. Cael, A. J. Heathcote and D. A. Seekell, The volume and mean depth of Earth's lakes, Geophys. Res. Lett., 2017, 44, 209–218 CrossRef.
- S. D. Thottathil, P. C. J. Reis and Y. T. Prairie, Methane oxidation kinetics in northern freshwater lakes, Biogeochemistry, 2019, 143, 105–116 CrossRef CAS.
- B. Lehner and P. Döll, Development and validation of a global database of lakes, reservoirs and wetlands, J. Hydrol., 2004, 296, 1–22 CrossRef.
- K. à. Norði, B. Thamdrup and C. J. Schubert, Anaerobic oxidation of methane in an iron-rich Danish freshwater lake sediment, Limnol. Oceanogr., 2013, 58, 546–554 CrossRef.
- W. Li, Y. Chen, M. Luo, C. Li, Y. Zhao, H. Chen, D. Feng and B. Hu, Comparison of Anaerobic Methane Oxidation in Different Sediment Habitats of Dianchi Lake, Water, Air, Soil Pollut., 2020, 231, 491 CrossRef CAS.
- A. A. Raghoebarsing, A. Pol and K. T. van de Pas-Schoonen, et al., A microbial consortium couples anaerobic methane oxidation to denitrification, Nature, 2006, 440, 918–921 CrossRef CAS PubMed.
- F. Einsiedl, A. Wunderlich, M. Sebilo, Ö. K. Coskun, W. D. Orsi and B. Mayer, Biogeochemical evidence of anaerobic methane oxidation and anaerobic ammonium oxidation in a stratified lake using stable isotopes, Biogeosciences, 2020, 17, 5149–5161 CrossRef CAS.
- E. H. Stanley, N. J. Casson, S. T. Christel, J. T. Crawford, L. C. Loken and S. K. Oliver, The ecology of methane in streams and rivers: patterns, controls, and global significance, Ecol. Monogr., 2016, 86, 146–171 CrossRef.
- M. Trimmer, F. C. Shelley, K. J. Purdy, S. T. Maanoja, P.-M. Chronopoulou and J. Grey, Riverbed methanotrophy sustained by high carbon conversion efficiency, ISME J., 2015, 9, 2304–2314 CrossRef CAS PubMed.
- M. Trimmer, S. Maanoja, A. G. Hildrew, J. L. Pretty and J. Grey, Potential carbon fixation via methane oxidation in well-oxygenated river bed gravels, Limnol. Oceanogr., 2010, 55, 560–568 CrossRef CAS.
- Y. Long, X. Jiang, Q. Guo, B. Li and S. Xie, Sediment nitrite-dependent methane-oxidizing microorganisms temporally and spatially shift in the Dongjiang River, Appl. Microbiol. Biotechnol., 2017, 101, 401–410 CrossRef CAS PubMed.
- L.-d. Shen, S. Liu, Q. Zhu, X.-y. Li, C. Cai, D.-q. Cheng, L.-p. Lou, X.-y. Xu, P. Zheng and B.-l. Hu, Distribution and Diversity of Nitrite-Dependent Anaerobic Methane-Oxidising Bacteria in the Sediments of the Qiantang River, Microb. Ecol., 2014, 67, 341–349 CrossRef CAS PubMed.
- F. Shelley, F. Abdullahi, J. Grey and M. Trimmer, Microbial methane cycling in the bed of a chalk river: oxidation has the potential to match methanogenesis enhanced by warming, Freshwater Biol., 2015, 60, 150–160 CrossRef CAS.
- X. Li, D. Y. F. Lai and D. Gao, Anaerobic oxidation of methane with denitrification in sediments of a subtropical estuary: Rates,
controlling factors and environmental implications, J. Environ. Manage., 2020, 273, 111151 CrossRef CAS PubMed.
- G. H. Allen and T. M. Pavelsky, Global extent of rivers and streams, Science, 2018, 361, 585–588 CrossRef CAS PubMed.
- G. N. Zaimes and R. C. Schultz, Stream bed substrate composition adjacent to different riparian land-uses in Iowa, USA, Ecol. Eng., 2011, 37, 1692–1699 CrossRef.
- M. Fejerskov, K. Kristensen and N. Friberg, Re-Meandering of Lowland Streams: Will Disobeying the Laws of Geomorphology Have Ecological Consequences?, PLoS One, 2014, 9, e108558 CrossRef PubMed.
- A. Matoušů, M. Rulík, M. Tušer, A. Bednařík, K. Šimek and I. Bussmann, Methane dynamics in a large river: a case study of the Elbe River, Aquat. Sci., 2018, 81, 12 CrossRef.
- A. V. Mardanov, V. V. Kadnikov, A. V. Beletsky and N. V. Ravin, Sulfur and Methane-Oxidizing Microbial Community in a Terrestrial Mud Volcano Revealed by Metagenomics, Microorganisms, 2020, 8, 1333 CrossRef CAS PubMed.
- P. H. A. Timmers, D. A. Suarez-Zuluaga, M. van Rossem, M. Diender, A. J. M. Stams and C. M. Plugge, Anaerobic oxidation of methane associated with sulfate reduction in a natural freshwater gas source, ISME J., 2016, 10, 1400–1412 CrossRef CAS PubMed.
- G. Ren, A. Ma, Y. Zhang, Y. Deng, G. Zheng, X. Zhuang, G. Zhuang and D. Fortin, Electron acceptors for anaerobic oxidation of methane drive microbial community structure and diversity in mud volcanoes, Environ. Microbiol., 2018, 20, 2370–2385 CrossRef CAS PubMed.
- H. Yoshioka, M. Takeuchi, S. Sakata, H. A. Takahashi, M. Takahashi, S. Tanabe, T. Hayashi, A. Inamura and M. Yasuhara, Microbial methane production and oxidation in the Holocene mud beneath the Kanto Plain of central Japan, Geochem. J., 2020, 54, 243–254 CrossRef CAS.
-
S. B. Joye and G. Zhuang, Carbon and sulfur cycling rate measurements and geochemistry from three sites in the northern South China Sea, Distributed by: Gulf of Mexico Research Initiative Information and Data Cooperative (GRIIDC), Harte Research Institute, Texas A&M University–Corpus Christi, 2018, DOI:10.7266/N74B2ZR9.
-
P. Girguis, Sediment geochemistry and alkane oxidation rates from sediments collected November 2010 and experimental incubations, Distributed by: Gulf of Mexico Research Initiative Information and Data Cooperative (GRIIDC), Harte Research Institute, Texas A&M University–Corpus Christi., 2016, DOI:10.7266/N7668B7N.
-
S. Joye, Sediment geochemistry and process rate measurements aboard the R/V Endeavor cruise EN586 in the northern Gulf of Mexico from 2016-07-26 to 2016-08-14, Distributed by: Gulf of Mexico Research Initiative Information and Data Cooperative (GRIIDC), Harte Research Institute, Texas A&M University–Corpus Christi, 2018, DOI:10.7266/N7B56H4H.
-
S. Joye, R/V Endeavor: EN528 Water and Sediment Chemistry, Gulf of Mexico, July 7-22, 2013, Distributed by: Gulf of Mexico Research Initiative Information and Data Cooperative (GRIIDC), Harte Research Institute, Texas A&M University–Corpus Christi, 2016, DOI:10.7266/N7G44N83.
- J. Rooze, M. Egger, I. Tsandev and C. P. Slomp, Iron-dependent anaerobic oxidation of methane in coastal surface sediments: Potential controls and impact, Limnol. Oceanogr., 2016, 61, S267–S282 CrossRef.
- X. Zhang, Y. Liu and J.-D. Gu, A global analysis on the distribution pattern of the bacteria coupling simultaneous methane oxidation to nitrite reduction, Int. Biodeterior. Biodegrad., 2018, 129, 123–132 CrossRef CAS.
- R. J. Parkes, B. A. Cragg, N. Banning, F. Brock, G. Webster, J. C. Fry, E. Hornibrook, R. D. Pancost, S. Kelly, N. Knab, B. B. Jørgensen, J. Rinna and A. J. Weightman, Biogeochemistry and biodiversity of methane cycling in subsurface marine sediments (Skagerrak, Denmark), Environ. Microbiol., 2007, 9, 1146–1161 CrossRef CAS PubMed.
- J. W. Pohlman, M. Riedel, J. E. Bauer, E. A. Canuel, C. K. Paull, L. Lapham, K. S. Grabowski, R. B. Coffin and G. D. Spence, Anaerobic methane oxidation in low-organic content methane seep sediments, Geochim. Cosmochim.
Acta, 2013, 108, 184–201 CrossRef CAS.
- J. J. Marlow, D. Hoer, S. P. Jungbluth, L. M. Reynard, A. Gartman, M. S. Chavez, M. Y. El-Naggar, N. Tuross, V. J. Orphan and P. R. Girguis, Carbonate-hosted microbial communities are prolific and pervasive methane oxidizers at geologically diverse marine methane seep sites, Proc. Natl. Acad. Sci. U. S. A., 2021, 118, e2006857118 CrossRef CAS PubMed.
- M. Egger, P. Kraal, T. Jilbert, F. Sulu-Gambari, C. J. Sapart, T. Röckmann and C. P. Slomp, Anaerobic oxidation of methane alters sediment records of sulfur, iron and phosphorus in the Black Sea, Biogeosciences, 2016, 13, 5333–5355 CrossRef CAS.
- N. J. Knab, A. W. Dale, K. Lettmann, H. Fossing and B. B. Jørgensen, Thermodynamic and kinetic control on anaerobic oxidation of methane in marine sediments, Geochim. Cosmochim. Acta, 2008, 72, 3746–3757 CrossRef CAS.
- P. Regnier, A. W. Dale, S. Arndt, D. E. LaRowe, J. Mogollón and P. Van Cappellen, Quantitative analysis of anaerobic oxidation of methane (AOM) in marine sediments: A modeling perspective, Earth-Sci. Rev., 2011, 106, 105–130 CrossRef CAS.
- F. Chen, Y. Zheng, L. Hou, J. Zhou, G. Yin and M. Liu, Denitrifying anaerobic methane oxidation in marsh sediments of Chongming eastern intertidal flat, Mar. Pollut. Bull., 2020, 150, 110681 CrossRef CAS PubMed.
- L.-d. Shen, B.-l. Hu, S. Liu, X.-p. Chai, Z.-f. He, H.-x. Ren, Y. Liu, S. Geng, W. Wang, J.-l. Tang, Y.-m. Wang, L.-p. Lou, X.-y. Xu and P. Zheng, Anaerobic methane oxidation coupled to nitrite reduction can be a potential methane sink in coastal environments, Appl. Microbiol. Biotechnol., 2016, 100, 7171–7180 CrossRef CAS PubMed.
- T. M. Hoehler, M. J. Alperin, D. B. Albert and C. S. Martens, Field and laboratory studies of methane oxidation in an anoxic marine sediment: Evidence for a methanogen-sulfate reducer consortium, Global Biogeochem. Cycles, 1994, 8, 451–463 CrossRef CAS.
- D. A. Aromokeye, A. C. Kulkarni, M. Elvert, G. Wegener, S. Henkel, S. Coffinet, T. Eickhorst, O. E. Oni, T. Richter-Heitmann, A. Schnakenberg, H. Taubner, L. Wunder, X. Yin, Q. Zhu, K.-U. Hinrichs, S. Kasten and M. W. Friedrich, Rates and Microbial Players of Iron-Driven Anaerobic Oxidation of Methane in Methanic Marine Sediments, Front. Microbiol., 2020, 10, 3041 CrossRef PubMed.
- S. D. Wankel, M. M. Adams, D. T. Johnston, C. M. Hansel, S. B. Joye and P. R. Girguis, Anaerobic methane oxidation in metalliferous hydrothermal sediments: influence on carbon flux and decoupling from sulfate reduction, Environ. Microbiol., 2012, 14, 2726–2740 CrossRef CAS PubMed.
- Y. Zhang, L. Maignien, A. Stadnitskaia, P. Boeckx, X. Xiao and N. Boon, Stratified Community Responses to Methane and Sulfate Supplies in Mud Volcano Deposits: Insights from an In Vitro Experiment, PLoS One, 2014, 9, e113004 CrossRef PubMed.
- L. Holmkvist, T. G. Ferdelman and B. B. Jørgensen, A cryptic sulfur cycle driven by iron in the methane zone of marine sediment (Aarhus Bay, Denmark), Geochim. Cosmochim. Acta, 2011, 75, 3581–3599 CrossRef CAS.
- N. Riedinger, M. J. Formolo, T. W. Lyons, S. Henkel, A. Beck and S. Kasten, An inorganic geochemical argument for coupled anaerobic oxidation of methane and iron reduction in marine sediments, Geobiology, 2014, 12, 172–181 CrossRef CAS PubMed.
- T. Treude, S. Krause, J. Maltby, A. W. Dale, R. Coffin and L. J. Hamdan, Sulfate reduction and methane oxidation activity
below the sulfate-methane transition zone in Alaskan Beaufort Sea continental margin sediments: Implications for deep sulfur cycling, Geochim. Cosmochim. Acta, 2014, 144, 217–237 CrossRef CAS.
- M. Krüger, T. Treude, H. Wolters, K. Nauhaus and A. Boetius, Microbial methane turnover in different marine habitats, Palaeogeogr., Palaeoclimatol., Palaeoecol., 2005, 227, 6–17 CrossRef.
- M. W. Bowles, V. A. Samarkin, K. M. Bowles and S. B. Joye, Weak coupling between sulfate reduction and the anaerobic oxidation of methane in methane-rich seafloor sediments during ex situ incubation, Geochim. Cosmochim. Acta, 2011, 75, 500–519 CrossRef CAS.
- A. S. Savvichev, V. V. Kadnikov, M. D. Kravchishina, S. V. Galkin, A. N. Novigatskii, P. A. Sigalevich, A. Y. Merkel, N. V. Ravin, N. V. Pimenov and M. V. Flint, Methane as an Organic Matter Source and the Trophic Basis of a Laptev Sea Cold Seep Microbial Community, Geomicrobiol. J., 2018, 35, 411–423 CrossRef.
- S. Bhattarai, C. Cassarini, E. R. Rene, Y. Zhang, G. Esposito and P. N. L. Lens, Enrichment of sulfate reducing anaerobic methane oxidizing community dominated by ANME-1 from Ginsburg Mud Volcano (Gulf of Cadiz) sediment in a biotrickling filter, Bioresour. Technol., 2018, 259, 433–441 CrossRef CAS PubMed.
- I. Y. Tarnovetskii, A. Y. Merkel, T. A. Kanapatskiy, E. A. Ivanova, M. B. Gulin, S. Toshchakov and N. V. Pimenov, Decoupling between sulfate reduction and the anaerobic oxidation of methane in the shallow methane seep of the Black sea, FEMS Microbiol. Lett., 2018, 365, fny235 CrossRef PubMed.
- N. V. Pimenov, T. A. Kanapatskii, P. A. Sigalevich, I. I. Rusanov, E. F. Veslopolova, A. G. Grigor’ev and V. A. Zhamoida, Sulfate reduction, methanogenesis, and methane oxidation in the Holocene sediments of the Vyborg Bay, Baltic Sea, Microbiology, 2012, 81, 79–89 CAS.
- W. S. Reeburgh, Oceanic Methane Biogeochemistry, Chem. Rev., 2007, 107, 486–513 CrossRef CAS PubMed.
- J. A. Bradley, S. Arndt, J. P. Amend, E. Burwicz, A. W. Dale, M. Egger and D. E. LaRowe, Widespread energy limitation to life in global subseafloor sediments, Sci. Adv., 2020, 6, eaba0697 CrossRef CAS PubMed.
- A. Skarke, C. Ruppel, M. Kodis, D. Brothers and E. Lobecker, Widespread methane leakage from the sea floor on the northern US Atlantic margin, Nat. Geosci., 2014, 7, 657–661 CrossRef CAS.
- S. D. Wankel, S. B. Joye, V. A. Samarkin, S. R. Shah, G. Friederich, J. Melas-Kyriazi and P. R. Girguis, New constraints on methane fluxes and rates of anaerobic methane oxidation in a Gulf of Mexico brine pool via in situ mass spectrometry, Deep Sea Res., Part II, 2010, 57, 2022–2029 CrossRef CAS.
- P. H. A. Timmers, J. Gieteling, H. C. A. Widjaja-Greefkes, C. M. Plugge, A. J. M. Stams, P. N. L. Lens and R. J. W. Meulepas, Growth of Anaerobic Methane-Oxidizing Archaea and Sulfate-Reducing Bacteria in a High-Pressure Membrane Capsule Bioreactor, Appl. Environ. Microbiol., 2015, 81, 1286–1296 CrossRef PubMed.
- C. Kelley, Methane oxidation potential in the water column of two diverse coastal marine sites, Biogeochemistry, 2003, 65, 105–120 CrossRef CAS.
- L. Valentine David, D. Kessler John, C. Redmond Molly, D. Mendes Stephanie, B. Heintz Monica, C. Farwell, L. Hu, S. Kinnaman Franklin, S. Yvon-Lewis, M. Du, W. Chan Eric, G. Tigreros Fenix and J. Villanueva Christie, Propane Respiration Jump-Starts Microbial Response to a Deep Oil Spill, Science, 2010, 330, 208–211 CrossRef CAS PubMed.
-
M.-K. Rogener, Methane concentration and oxidation rates in water column data collected aboard the R/V Sikuliaq in the northern Chukchi Sea from 2017-08-11 to 2017-08-20, Distributed by: Gulf of Mexico Research Initiative Information and Data Cooperative (GRIIDC), Harte Research Institute, Texas A&M University–Corpus Christi, 2019, DOI:10.7266/n7-38jp-7p37.
-
S. Joye and M. Saxton, R/V Endeavor: EN559 Water Column Chemistry and Microbial Process Rate Measurements, Gulf of Mexico, May 29 - June 20, 2015, Distributed by: Gulf of Mexico Research Initiative Information and Data Cooperative (GRIIDC), Harte Research Institute, Texas A&M University–Corpus Christi, 2017, DOI:10.7266/N7QR4V7H.
-
S. Joye, Water Column Chemistry Data Collected on Board the R/V Endeavor Cruise EN586 in the Northern Gulf of Mexico from 2016-07-24 to 2016-08-14, Distributed by: Gulf of Mexico Research Initiative Information and Data Cooperative (GRIIDC), Harte Research Institute, Texas A&M University–Corpus Christi, 2018, DOI:10.7266/N7VH5M5X.
- P. L. Tavormina, W. Ussler, S. B. Joye, B. K. Harrison and V. J. Orphan, Distributions of putative aerobic methanotrophs in diverse pelagic marine environments, ISME J., 2010, 4, 700–710 CrossRef PubMed.
- B. B. Ward, K. A. Kilpatrick, A. E. Wopat, E. C. Minnich and M. E. Lidstrom, Methane oxidation in Saanich inlet during summer stratification, Cont. Shelf Res., 1989, 9, 65–75 CrossRef.
- M. K. Rogener, R. E. Sipler, K. S. Hunter, D. A. Bronk and S. B. Joye, Pelagic methane oxidation in the northern Chukchi Sea, Limnol. Oceanogr., 2020, 65, 96–110 CrossRef CAS.
- L. Tavormina Patricia, W. Ussler and J. Orphan Victoria, Planktonic and Sediment-Associated Aerobic Methanotrophs in Two Seep Systems along the North American Margin, Appl. Environ. Microbiol., 2008, 74, 3985–3995 CrossRef CAS PubMed.
-
L. I. Steinle, PhD thesis, University of Basel, Faculty of Science, 2016.
- M. Crespo-Medina, C. D. Meile, K. S. Hunter, A. R. Diercks, V. L. Asper, V. J. Orphan, P. L. Tavormina, L. M. Nigro, J. J. Battles, J. P. Chanton, A. M. Shiller, D. J. Joung, R. M. W. Amon, A. Bracco, J. P. Montoya, T. A. Villareal, A. M. Wood and S. B. Joye, The rise and fall of methanotrophy following a deepwater oil-well blowout, Nat. Geosci., 2014, 7, 423–427 CrossRef CAS.
- M. Leonte, J. D. Kessler, M. Y. Kellermann, E. C. Arrington, D. L. Valentine and S. P. Sylva, Rapid rates of aerobic methane oxidation at the feather edge of gas hydrate stability in the waters of Hudson Canyon, US Atlantic Margin, Geochim. Cosmochim. Acta, 2017, 204, 375–387 CrossRef CAS.
- A. Boetius and F. Wenzhöfer, Seafloor oxygen consumption fuelled by methane from cold seeps, Nat. Geosci., 2013, 6, 725–734 CrossRef CAS.
- L. Steinle, J. Maltby, T. Treude, A. Kock, H. W. Bange, N. Engbersen, J. Zopfi, M. F. Lehmann and H. Niemann, Effects of low oxygen concentrations on aerobic methane oxidation in seasonally hypoxic coastal waters, Biogeosciences, 2017, 14, 1631–1645 CrossRef CAS.
- P.-M. Chronopoulou, F. Shelley, W. J. Pritchard, S. T. Maanoja and M. Trimmer, Origin and fate of methane in the Eastern Tropical North Pacific oxygen minimum zone, ISME J., 2017, 11, 1386–1399 CrossRef CAS PubMed.
- S. Mau, T.-H. Tu, M. Becker, C. dos Santos Ferreira, J.-N. Chen, L.-H. Lin, P.-L. Wang, S. Lin and G. Bohrmann, Methane Seeps and Independent Methane Plumes in the South China Sea Offshore Taiwan, Front. Mar. Sci., 2020, 7, 543 CrossRef.
-
W. S. Reeburgh, S. C. Whalen and M. J. Alperin, in Microbial Growth on C1 Compounds, ed. J. C. Murrell and D. P. Kelly, Intercept, Andover, UK, 1993, pp. 1–14 Search PubMed.
-
W. S. Reeburgh, in Microbial Growth on C1 Compounds: Proceedings of the 8th International Symposium on Microbial Growth on C1 Compounds, held in San Diego, U.S.A., 27 August – 1 September 1995, ed. M. E. Lidstrom and F. R. Tabita, Springer Netherlands, Dordrecht, 1996, pp. 334–342, DOI:10.1007/978-94-009-0213-8_44.
-
B. B. Jørgensen and S. Kasten, in Marine Geochemistry, ed. H. D. Schulz and M. Zabel, Springer Berlin Heidelberg, Berlin, Heidelberg, 2006, pp. 271–309, DOI:10.1007/3-540-32144-6_8.
- S. Scheller, H. Yu, G. L. Chadwick, S. E. McGlynn and V. J. Orphan, Artificial electron acceptors decouple archaeal methane oxidation from sulfate reduction, Science, 2016, 351, 703–707 CrossRef CAS PubMed.
- S. van Grinsven, J. S. Sinninghe Damsté and L. Villanueva, Assessing the Effect of Humic Substances and Fe(III) as Potential Electron Acceptors for Anaerobic Methane Oxidation in a Marine Anoxic System, Microorganisms, 2020, 8, 1288 CrossRef CAS PubMed.
- J.-P. Myllykangas, A. J. Rissanen, S. Hietanen and T. Jilbert, Influence of electron acceptor availability and microbial community structure on sedimentary methane oxidation in a boreal estuary, Biogeochemistry, 2020, 148, 291–309 CrossRef.
- C. E. G. Fernandes, M. J. B. D. Gonsalves, D. R. Nazareth, L. Nagarchi and S. A. Kamaleso, Microbial iron reduction and methane oxidation in subsurface sediments of the Arabian Sea, Mar. Pet. Geol., 2015, 67, 327–335 CrossRef CAS.
- M. Egger, O. Rasigraf, C. J. Sapart, T. Jilbert, M. S. M. Jetten, T. Röckmann, C. van der Veen, N. Bândă, B. Kartal, K. F. Ettwig and C. P. Slomp, Iron-Mediated Anaerobic Oxidation of Methane in Brackish Coastal Sediments, Environ. Sci. Technol., 2015, 49, 277–283 CrossRef CAS PubMed.
- C. P. Slomp, H. P. Mort, T. Jilbert, D. C. Reed, B. G. Gustafsson and M. Wolthers, Coupled Dynamics of Iron and Phosphorus in Sediments of an Oligotrophic Coastal Basin and the Impact of Anaerobic Oxidation of Methane, PLoS One, 2013, 8, e62386 CrossRef CAS PubMed.
- H. Vigderovich, L. Liang, B. Herut, F. Wang, E. Wurgaft, M. Rubin-Blum and O. Sivan, Evidence for microbial iron reduction in the methanic sediments of the oligotrophic southeastern Mediterranean continental shelf, Biogeosciences, 2019, 16, 3165–3181 CrossRef CAS.
- M. Egger, M. Hagens, C. J. Sapart, N. Dijkstra, N. A. G. M. van Helmond, J. M. Mogollón, N. Risgaard-Petersen, C. van der Veen, S. Kasten, N. Riedinger, M. E. Böttcher, T. Röckmann, B. B. Jørgensen and C. P. Slomp, Iron oxide reduction in methane-rich deep Baltic Sea sediments, Geochim. Cosmochim. Acta, 2017, 207, 256–276 CrossRef CAS.
- A. J. Findlay, A. Pellerin, K. Laufer and B. B. Jørgensen, Quantification of sulphide oxidation rates in marine sediment, Geochim. Cosmochim. Acta, 2020, 280, 441–452 CrossRef CAS.
- J. Chen, Z. Zhou and J.-D. Gu, Complex community of nitrite-dependent anaerobic methane oxidation bacteria in coastal sediments of the Mai Po wetland by PCR amplification of both 16S rRNA and pmoA genes, Appl. Microbiol. Biotechnol., 2015, 99, 1463–1473 CrossRef CAS PubMed.
- Y. Niu, Y. Zheng, L. Hou, D. Gao, F. Chen, C. Pei, H. Dong, X. Liang and M. Liu, Microbial dynamics and activity of denitrifying anaerobic methane oxidizers in China's estuarine and coastal wetlands, Sci. Total Environ., 2022, 806, 150425 CrossRef CAS PubMed.
- Z. He, S. Geng, C. Cai, S. Liu, Y. Liu, Y. Pan, L. Lou, P. Zheng, X. Xu and B. Hu, Anaerobic Oxidation of Methane Coupled to Nitrite Reduction by Halophilic Marine NC10 Bacteria, Appl. Environ. Microbiol., 2015, 81, 5538–5545 CrossRef CAS PubMed.
- J. Wang, L. Shen, Z. He, J. Hu, Z. Cai, P. Zheng and B. Hu, Spatial and temporal distribution of nitrite-dependent anaerobic methane-oxidizing bacteria in an intertidal zone of the East China Sea, Appl. Microbiol. Biotechnol., 2017, 101, 8007–8014 CrossRef CAS PubMed.
- J. Wang, C. Cai, Y. Li, M. Hua, J. Wang, H. Yang, P. Zheng and B. Hu, Denitrifying Anaerobic Methane Oxidation: A Previously Overlooked Methane Sink in Intertidal Zone, Environ. Sci. Technol., 2019, 53, 203–212 CrossRef CAS PubMed.
- Y. Zheng, L. Hou, F. Chen, J. Zhou, M. Liu, G. Yin, J. Gao and P. Han, Denitrifying anaerobic methane oxidation in intertidal marsh soils: Occurrence and environmental significance, Geoderma, 2020, 357, 113943 CrossRef CAS.
- S. Bhattarai, Y. Zhang and P. N. L. Lens, Effect of pressure and temperature on anaerobic methanotrophic activities of a highly enriched ANME-2a community, Environ. Sci. Pollut. Res., 2018, 25, 30031–30043 CrossRef CAS PubMed.
- T. Holler, F. Widdel, K. Knittel, R. Amann, M. Y. Kellermann, K.-U. Hinrichs, A. Teske, A. Boetius and G. Wegener, Thermophilic anaerobic oxidation of methane by marine microbial consortia, ISME J., 2011, 5, 1946–1956 CrossRef CAS PubMed.
- H. Niemann, T. Lösekann, D. de Beer, M. Elvert, T. Nadalig, K. Knittel, R. Amann, E. J. Sauter, M. Schlüter, M. Klages, J. P. Foucher and A. Boetius, Novel microbial communities of the Haakon Mosby mud volcano and their role as a methane sink, Nature, 2006, 443, 854–858 CrossRef CAS PubMed.
- D. H. Lee, J. H. Kim, Y. M. Lee, A. Stadnitskaia, Y. K. Jin, H. Niemann, Y. G. Kim and K. H. Shin, Biogeochemical evidence of anaerobic methane oxidation on active submarine mud volcanoes on the continental slope of the Canadian Beaufort Sea, Biogeosciences, 2018, 15, 7419–7433 CrossRef.
- A. V. Lomakina, E. V. Mamaeva, T. V. Pogodaeva, G. V. Kalmychkov, I. A. Khal'zov and T. I. Zemskaya, Anaerobic Methane Oxidation in Enrichment Cultures from Deep Sediments of a Mud Volcano Peschanka (South Baikal), Microbiology, 2018, 87, 317–325 CAS.
- G.-J. Xie, T. Liu, C. Cai, S. Hu and Z. Yuan, Achieving high-level nitrogen removal in mainstream by coupling anammox with denitrifying anaerobic methane oxidation in a membrane biofilm reactor, Water Res., 2018, 131, 196–204 CrossRef CAS PubMed.
- J. Pang, L. Liu, X. Liu, Y. Wang, B. Chen, S. Wu, J. Yao and X. Xu, A novel identified Pseudomonas aeruginosa, which exhibited nitrate- and nitrite-dependent methane oxidation abilities, could alleviate the disadvantages caused by nitrate supplementation in rumen fluid fermentation, Microb. Biotechnol., 2021, 14, 1397–1408 CrossRef CAS PubMed.
- S. Hu, R. J. Zeng, L. C. Burow, P. Lant, J. Keller and Z. Yuan, Enrichment of denitrifying anaerobic methane oxidizing microorganisms, Environ. Microbiol. Rep., 2009, 1, 377–384 CrossRef CAS PubMed.
- C. Kampman, H. Temmink, T. L. G. Hendrickx, G. Zeeman and C. J. N. Buisman, Enrichment of denitrifying methanotrophic bacteria from municipal wastewater sludge in a membrane bioreactor at 20 °C, J. Hazard. Mater., 2014, 274, 428–435 CrossRef CAS PubMed.
- M. Hatamoto, T. Sato, S. Nemoto and T. Yamaguchi, Cultivation of denitrifying anaerobic methane-oxidizing microorganisms in a continuous-flow sponge bioreactor, Appl. Microbiol. Biotechnol., 2017, 101, 5881–5888 CrossRef CAS PubMed.
- W. Li, P. Lu, L. Zhang, A. Ding, X. Wang, H. Yang and D. Zhang, Long-term performance of denitrifying anaerobic methane oxidation under stepwise cooling and ambient temperature conditions, Sci. Total Environ., 2020, 713, 136739 CrossRef CAS PubMed.
- Z. He, S. Geng, L. Shen, L. Lou, P. Zheng, X. Xu and B. Hu, The short- and long-term effects of environmental conditions on anaerobic methane oxidation coupled to nitrite reduction, Water Res., 2015, 68, 554–562 CrossRef CAS PubMed.
- Z. Hu, D. Ru, Y. Wang, J. Zhang, L. Jiang, X. Xu and L. Nie, Optimization of a nitrite-dependent anaerobic methane oxidation (n-damo) process by enhancing methane availability, Bioresour. Technol., 2019, 275, 101–108 CrossRef CAS PubMed.
- J. Chang, Q. Wu, X. Yan, H. Wang, L. W. Lee, Y. Liu, P. Liang, Y. Qiu and X. Huang, Enhancement of nitrite reduction and enrichment of Methylomonas via conductive materials in a nitrite-dependent anaerobic methane oxidation system, Environ. Res., 2021, 193, 110565 CrossRef CAS PubMed.
- Z. He, C. Cai, S. Geng, L. Lou, X. Xu, P. Zheng and B. Hu, Modelling a nitrite-dependent anaerobic methane oxidation process: Parameters identification and model evaluation, Bioresour. Technol., 2013, 147, 315–320 CrossRef CAS PubMed.
- P. Lu, X. Wang, Y. Tang, A. Ding, H. Yang, J. Guo, Y. Cui and C. Ling, Granular activated carbon assisted nitrate-dependent anaerobic methane oxidation-membrane bioreactor: Strengthening effect and mechanisms, Environ. Int., 2020, 138, 105675 CrossRef CAS PubMed.
- W. Li, P. Lu, F. Chai, L. Zhang, X. Han and D. Zhang, Long-term nitrate removal through methane-dependent denitrification microorganisms in sequencing batch reactors fed with only nitrate and methane, AMB Express, 2018, 8, 108 CrossRef PubMed.
- L. Liu, X. Xu, Y. Cao, C. Cai, H. Cui and J. Yao, Nitrate decreases methane production also by increasing methane oxidation through stimulating NC10 population in ruminal culture, AMB Express, 2017, 7, 76 CrossRef PubMed.
- L. Gambelli, S. Guerrero-Cruz, R. J. Mesman, G. Cremers, M. S. M. Jetten, H. J. M. O. d. Camp, B. Kartal, C. Lueke, L. v. Niftrik and H. L. Drake, Community Composition and Ultrastructure of a Nitrate-Dependent Anaerobic Methane-Oxidizing Enrichment Culture, Appl. Environ. Microbiol., 2018, 84, e02186-17 CrossRef PubMed.
- S. Hu, R. J. Zeng, M. F. Haroon, J. Keller, P. A. Lant, G. W. Tyson and Z. Yuan, A laboratory investigation of interactions between denitrifying anaerobic methane oxidation (DAMO) and anammox processes in anoxic environments, Sci. Rep., 2015, 5, 8706 CrossRef CAS PubMed.
- S.-Q. Fan, G.-J. Xie, Y. Lu, B.-F. Liu, D.-F. Xing, H.-J. Han, Z. Yuan and N.-Q. Ren, Granular Sludge Coupling Nitrate/Nitrite Dependent Anaerobic Methane Oxidation with Anammox: from Proof-of-Concept to High Rate Nitrogen Removal, Environ. Sci. Technol., 2020, 54, 297–305 CrossRef CAS PubMed.
- L. Fu, F. Zhang, Y.-N. Bai, Y.-Z. Lu, J. Ding, D. Zhou, Y. Liu and R. J. Zeng, Mass transfer affects reactor performance, microbial morphology, and community succession in the methane-dependent denitrification and anaerobic ammonium oxidation co-culture, Sci. Total Environ., 2019, 651, 291–297 CrossRef CAS PubMed.
- W.-B. Nie, G.-J. Xie, J. Ding, Y. Lu, B.-F. Liu, D.-F. Xing, Q. Wang, H.-J. Han, Z. Yuan and N.-Q. Ren, High performance nitrogen removal through integrating denitrifying anaerobic methane oxidation and Anammox: from enrichment to application, Environ. Int., 2019, 132, 105107 CrossRef CAS PubMed.
- K. Stultiens, S. G. Cruz, M. A. H. J. van Kessel, M. S. M. Jetten, B. Kartal and H. J. M. Op den, Camp, Interactions between anaerobic ammonium- and methane-oxidizing microorganisms in a laboratory-scale sequencing batch reactor, Appl. Microbiol. Biotechnol., 2019, 103, 6783–6795 CrossRef CAS PubMed.
- C. Cassarini, Y. Zhang and P. N. L. Lens, Pressure Selects Dominant Anaerobic Methanotrophic Phylotype and Sulfate Reducing Bacteria in Coastal Marine Lake Grevelingen Sediment, Front. Environ. Sci., 2019, 6, 162 CrossRef.
- J. B. Glass, H. Yu, J. A. Steele, K. S. Dawson, S. Sun, K. Chourey, C. Pan, R. L. Hettich and V. J. Orphan, Geochemical, metagenomic and metaproteomic insights into trace metal utilization by methane-oxidizing microbial consortia in sulphidic marine sediments, Environ. Microbiol., 2014, 16, 1592–1611 CrossRef CAS PubMed.
- K. E. A. Segarra, C. Comerford, J. Slaughter and S. B. Joye, Impact of electron acceptor availability on the anaerobic oxidation of methane in coastal freshwater and brackish wetland sediments, Geochim. Cosmochim. Acta, 2013, 115, 15–30 CrossRef CAS.
- Y. Zhang, L. Maignien, X. Zhao, F. Wang and N. Boon, Enrichment of a microbial community performing anaerobic oxidation of methane in a continuous high-pressure bioreactor, BMC Microbiol., 2011, 11, 137 CrossRef CAS PubMed.
- Z. He, S. Geng, C. Cai, S. Liu, Y. Liu, Y. Pan, L. Lou, P. Zheng, X. Xu and B. Hu, Anaerobic Oxidation of Methane Coupled to Nitrite Reduction by Halophilic Marine NC10 Bacteria, Appl. Environ. Microbiol., 2015, 81, 5538–5545 CrossRef CAS PubMed.
- M. Laureni, D. G. Weissbrodt, I. Szivák, O. Robin, J. L. Nielsen, E. Morgenroth and A. Joss, Activity and growth of anammox biomass on aerobically pre-treated municipal wastewater, Water Res., 2015, 80, 325–336 CrossRef CAS PubMed.
- G. Wu, T. Zhang, M. Gu, Z. Chen and Q. Yin, Review of characteristics of anammox bacteria and strategies for anammox start-up for sustainable wastewater resource management, Water Sci. Technol., 2020, 82, 1742–1757 CrossRef CAS PubMed.
- K. Stultiens, M. A. H. J. van Kessel, J. Frank, P. Fischer, C. Pelzer, T. A. van Alen, B. Kartal, H. J. M. Op den Camp and M. S. M. Jetten, Diversity, enrichment, and genomic potential of anaerobic methane- and ammonium-oxidizing microorganisms from a brewery wastewater treatment plant, Appl. Microbiol. Biotechnol., 2020, 104, 7201–7212 CrossRef CAS PubMed.
- G. C. Jagersma, R. J. W. Meulepas, I. Heikamp-De Jong, J. Gieteling, A. Klimiuk, S. Schouten, J. S. Sinninghe Damsté, P. N. L. Lens and A. J. M. Stams, Microbial diversity and community structure of a highly active anaerobic methane-oxidizing sulfate-reducing enrichment, Environ. Microbiol., 2009, 11, 3223–3232 CrossRef CAS PubMed.
- G. Wegener, V. Krukenberg, S. E. Ruff, M. Y. Kellermann and K. Knittel, Metabolic Capabilities of Microorganisms Involved in and Associated with the Anaerobic Oxidation of Methane, Front. Microbiol., 2016, 7, 46 Search PubMed.
- S. L. Caldwell, J. R. Laidler and E. A. Brewer,
et al., Anaerobic oxidation of methane: mechanisms, bioenergetics, and the ecology of associated microorganisms, Environ. Sci. Technol., 2008, 42, 6791–6799 CrossRef CAS PubMed.
- S. Scheller, M. Goenrich, R. Boecher, R. K. Thauer and B. Jaun, The key nickel enzyme of methanogenesis catalyses the anaerobic oxidation of methane, Nature, 2010, 465, 606–608 CrossRef CAS PubMed.
-
B. d. Darwent, Bond Dissociation Energies in Simple Molecules, U.S. National Bureau of Standards; for Sale by the Superintendent of Documents, U.S. Government Printing Office, Washington, 1970 Search PubMed.
- S. Scheller, H. Yu, L. Chadwick Grayson, E. McGlynn Shawn and J. Orphan Victoria, Artificial electron acceptors decouple archaeal methane oxidation from sulfate reduction, Science, 2016, 351, 703–707 CrossRef CAS PubMed.
- I. Irakulis-Loitxate, L. Guanter, Y.-N. Liu, D. J. Varon, J. D. Maasakkers, Y. Zhang, A. Chulakadabba, S. C. Wofsy, A. K. Thorpe, R. M. Duren, C. Frankenberg, D. R. Lyon, B. Hmiel, D. H. Cusworth, Y. Zhang, K. Segl, J. Gorroño, E. Sánchez-García, M. P. Sulprizio, K. Cao, H. Zhu, J. Liang, X. Li, I. Aben and D. J. Jacob, Satellite-based survey of extreme methane emissions in the Permian basin, Sci. Adv., 2021, 7, eabf4507 CrossRef CAS PubMed.
- P. Dunfield, R. knowles, R. Dumont and T. R. Moore, Methane production and consumption in temperate and subarctic peat soils: Response to temperature and pH, Soil Biol. Biochem., 1993, 25, 321–326 CrossRef CAS.
- S. Kirschke, P. Bousquet, P. Ciais, M. Saunois, J. G. Canadell, E. J. Dlugokencky, P. Bergamaschi, D. Bergmann, D. R. Blake, L. Bruhwiler, P. Cameron-Smith, S. Castaldi, F. Chevallier, L. Feng, A. Fraser, M. Heimann, E. L. Hodson, S. Houweling, B. Josse, P. J. Fraser, P. B. Krummel, J.-F. Lamarque, R. L. Langenfelds, C. Le Quéré, V. Naik, S. O'Doherty, P. I. Palmer, I. Pison, D. Plummer, B. Poulter, R. G. Prinn, M. Rigby, B. Ringeval, M. Santini, M. Schmidt, D. T. Shindell, I. J. Simpson, R. Spahni, L. P. Steele, S. A. Strode, K. Sudo, S. Szopa, G. R. van der Werf, A. Voulgarakis, M. van Weele, R. F. Weiss, J. E. Williams and G. Zeng, Three decades of global methane sources and sinks, Nat. Geosci., 2013, 6, 813–823 CrossRef CAS.
- N. Gedney, P. M. Cox and C. Huntingford, Climate feedback from wetland methane emissions, Geophys. Res. Lett., 2004, 31, L20503 CrossRef.
- A. V. Eliseev, I. I. Mokhov, M. M. Arzhanov, P. F. Demchenko and S. N. Denisov, Interaction of the methane cycle and processes in wetland ecosystems in a climate model of intermediate complexity, Izv., Atmos. Oceanic Phys., 2008, 44, 139 CrossRef.
-
United Nations Environment Programme and Climate and Clean Air Coalition, Global Methane Assessment: Benefits and Costs of Mitigating Methane Emissions, United Nations Environment Programme, Nairobi, 2021 Search PubMed.
Footnote |
† Electronic supplementary information (ESI) available: Text on CiteSpace analysis, statistical data analysis, AOM information for cold seep systems, and data used for Table 1 and Fig. 3–7. See https://doi.org/10.1039/d2va00091a |
|
This journal is © The Royal Society of Chemistry 2022 |