Magnetic MOF-808 as a novel adsorbent for toxic metal removal from aqueous solutions†
Received
18th January 2022
, Accepted 29th March 2022
First published on 7th April 2022
Abstract
Mercury (Hg) in water is a global concern due to its high toxicity and bioaccumulation in the food chain, which severely affects the environment and human health. In this work, a magnetic zirconium metal–organic framework (Zr-MOF@Fe3O4) has been synthesized for Hg(II) removal. The Zr-MOF@Fe3O4 demonstrated the maximum adsorption capacity of 303 mg g−1 at pH 6. The possible mechanism was confirmed by spectroscopic analysis, which indicated that free –COOH groups and Zr–OH were responsible for adsorption. The adsorbent showed high uptake capacity for Hg(II), Pb(II), and Cd(II) ions in a multi-element solution. The regeneration of the material was successfully carried out with NaOH solution for five cycles. Thus, the study has reported a highly efficient adsorbent for the removal of toxic heavy metals from aqueous solutions.
Environmental significance
Researchers are concerned about water contamination with toxic heavy metals, especially, by lead, mercury, and cadmium poisoning, which is risking millions of lives in rural and semi-urbanized regions of the world. Thus, the decontamination of water for its safe use in drinking and domestic applications needs to be prioritized. Here, we have successfully developed a facile magnetic zirconium-based metal–organic framework composite for simultaneous removal of divalent lead, mercury, and cadmium ions at a near-neutral pH for its application in the treatment of industrial wastewater and groundwater. Imparting magnetism into the metal–organic framework made the phase separation process easy and efficient. The entire water decontamination process was found to be energy-efficient and reversible with the use of affordable NaOH solution as a stripping agent.
|
Introduction
Heavy metals are considered one of the most hazardous pollutants in water due to their high toxicity to humans and low biodegradability.1 One of these metals is mercury, which is a widespread global concern. This pollutant causes serious environmental problems and dangers to human health due to its high toxicity, bio-accumulation, and ability to cause memory loss, Alzheimer's, nephrotic syndrome with high levels of protein in the urine, paralysis coma, and congenital deformities affecting foetuses.2 The main chemical species of mercury in the environment are the elemental Hg (Hg0), inorganic Hg (Hg(II)), and organic forms, primarily methyl-Hg (MeHg). The inorganic Hg has poor degradability in natural water (or wastewater) and long-range transport in the atmosphere. Also, the Hg(II) species have become the main factor in giving rise to mercury pollution worldwide. For that reason, the World Health Organization (WHO) has set the maximum level of mercury contamination in drinking water at 1 ppb.3 Therefore, it is a significant challenge for water safety to explore more efficient materials to remove the ultra-low concentration of Hg(II) from contaminated water. The traditional treatment technologies for the removal of mercury include ion exchange,4 amalgamation,5 membrane separation,6 and chemical precipitation.7 Among these, the most studied technique is adsorption due to its simplicity, high removal efficiency, and affordability.8–10
Metal–organic frameworks (MOFs) are materials formed by the coordination of metal ions or metal clusters with organic ligands. MOFs are good candidates for wastewater adsorption treatments, increasing their use in the past decades. Besides, MOFs have important characteristics, such as large specific surface area, designable frame structure, controllable pore size, metal unsaturation sites, and ease of modification.11,12
These properties make these materials excellent candidates for metal removal from contaminated water. Modified Zr-MOFs have been showing promising results in the adsorption of highly toxic chemical species such as heavy metal ions like Hg(II), Cd(II), and Pb(II). Liu et al. proved the adsorption capacity of MOF-808 and amidoxime-functionalized MOF-808 in the removal of Hg(II) with an adsorption capacity of 343.6 and 383.8 mg g−1, respectively.13 Also, the modification of MOF-808 with ethylenediaminetetraacetate made it possible to capture 22 metals, including soft, hard, and edge metals ions.14 Efome et al. prepared a PAN/MOF-808 membrane with high carboxylate density adsorbed Hg ions by electrostatic interaction.15 The previous studies provide the possible mechanism, optimization parameters, and adsorption capacity of those materials. However, many studies lack detailed investigation on the adsorption mechanism and the reusability of the materials. Moreover, the most important aspect of magnetic phase separation of spent adsorbents has not been reported in these studies.
Herein, we report a novel magnetic Zr-MOF with a low-cost synthesis procedure. The material has functional groups and characteristics that allow the efficient adsorptive removal of Hg(II) and other heavy metals, with easy separation after the removal process. The structure and functional characterization of the Zr-MOF@Fe3O4 was carried out using multiple analytical techniques. The adsorption kinetics, isotherms, thermodynamics, and reusability of the Zr-MOF@Fe3O4 were also studied. The mechanism of adsorption was determined. The reusability of the adsorbent was evaluated over five cycles with NaOH and HCl as eluents. The study confirmed the development of a novel magnetic MOF with high adsorption capacity, good reusability, and an efficient separation process for wastewater treatment.
Materials and methods
Chemicals and reagents
Zirconyl chloride octahydrate (ZrOCl2·8H2O, reagent grade 98%), trimesic acid (H3BTC, purity 95%), anhydrous dimethylformamide (DMF, purity 99.8%), iron chloride hexahydrate (FeCl3·6H2O, ACS reagent ≥99%), iron sulfate heptahydrate (FeSO4·7H2O, ACS reagent, ≥99.0%), sodium hydroxide pellets (NaOH, purity ≥97.0%), nitric acid (HNO3 ACS reagent, 70%), mercury(II) sulfate (HgSO4, ACS reagent ≥99%), ICP standards of Hg(II), Cd(II), and Pb(II) cations (1000 ± 2 mg L−1) were supplied by Sigma-Aldrich, Germany. Methanol (ACS reagent chemical, anhydrous, 99.8%) was purchased from Baker®. The reagents were used without further purification.
Fabrication of the magnetic Zr-MOF
The MOF was synthesized by the solvothermal method. Exactly 2.57 g of ZrOCl2·8H2O and 1.68 g of trimesic acid (H3BTC) were stirred and sonicated in 40 mL of DMF solvent. The solution was transferred to a Teflon-lined autoclave reactor and heated in an oven at 180 °C for 24 h. The supernatant solid was separated by centrifugation and washed three times with DMF and three times with methanol. The solid product (Zr-MOF) was dried at 50 °C for 24 h. Fe3O4 nanoparticles were synthesized using the co-precipitation method reported in our previously published work.16 The synthesis of the magnetic Zr-MOF was carried out by a simple mixing and stirring process. Firstly, 1.0 g of Zr-MOF in 50 mL of water and 0.5 g of Fe3O4 in 50 mL of water were separately sonicated. Then both suspensions were mixed and stirred for 3 h. After that, the magnetic solid phase was separated employing a magnet and then washed with water. The solid obtained (magnetic Zr-MOF) was dried at 50 °C for 24 h.
Analytical instruments
The detailed information on the instrumental techniques is available in Section S1.†
Adsorption methodology
The experiments to determine the optimized parameters (pH, contact time, adsorbent dosage, initial Hg(II) concentration, and reusability) were performed at room temperature by soaking 30 mg of the magnetic MOF in a 30 mL aqueous metal ion solution (50 mg L−1) for 12 h at pH 5.5. For each experiment, the conditions were changed accordingly to understand the effect of experimental parameters on the Hg(II) adsorption capacity of the magnetic MOF (Table S1†). The pH-dependent study was carried out by adjusting the pH using NaOH/HNO3 solutions (0.1 mol L−1) and soaking 30 mg of the magnetic MOF in a 30 mL of Hg(II) solution (50 mg L−1) for 3 h. The pH measurement was conducted on a Thermo Scientific pH meter. Exactly 90 mg of magnetic MOF was soaked in 90 mL of Hg(II) (50 mg L−1) solution for 0–24 h for the time-dependent study. The regeneration of the Hg-loaded composite was done using 30 mL of 0.1 mol L−1 NaOH/HCl aqueous solution using 30 mg of the magnetic MOF for 3 h. After 6 h of drying, the regenerated composite was studied for the next cycle. The adsorption capacity (qe, mg g−1) at equilibrium was calculated using eqn (1) for each experiment. | 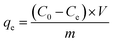 | (1) |
where V, m, C0, and Ce are the volume of aqueous solution (L), amount of the adsorbent (g), initial concentration (mg L−1), and equilibrium concentration (mg L−1), respectively.
Results and discussion
Characterization of the adsorbents
To study the morphologies, topography, and the chemical composition of the samples, the SEM micrograph analysis and the corresponding EDAX mapping of Fe3O4, Zr-MOF, and Zr-MOF@Fe3O4 were carried out (Fig. 1). As observed in the SEM micrographs, the morphology of the material is not well defined (Fig. 1b), however after Fe3O4 incorporation it is possible to observe small particle aggregates (morphology observed for Fe3O4 nanoparticles, Fig. 1a) on the MOF surface. This evidences that magnetite nanoparticles have been incorporated onto the MOF material (Fig. 1c). The elemental analysis of Fe3O4 shows peaks of O and Fe in the spectrum (Fig. 1a.1). For Zr-MOF, peaks of Zr, O, and C could be observed in the EDAX spectrum (Fig. 1b.1). The formation of the Zr-MOF@Fe3O4 composite and its purity were confirmed by the well-defined peaks of C, O, Fe, and Zr in the spectrum (Fig. 1c.1). The 2D elemental map of Zr-MOF and Zr-MOF@Fe3O4 is available in Fig. S1.† A uniform distribution of O, C, and Zr was confirmed in the Zr-MOF. In Zr-MOF@Fe3O4, a large fraction of the ‘Fe’ density could be observed over the ‘Zr’ density in the MOF, which further confirmed the formation of the composite of magnetite and the Zr-MOF.
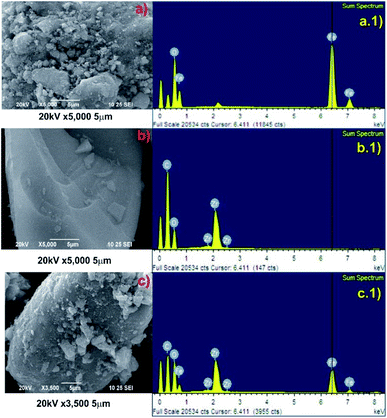 |
| Fig. 1 SEM micrographs and EDAX analysis of (a) Fe3O4; (b) Zr-MOF; (c) Zr-MOF@Fe3O4. | |
The PXRD technique provides us with information about the crystallinity and phase purity of the materials (Fig. 2a). The PXRD pattern of the Zr-MOF has a high-intensity peak in the 5–15° range centred at 2θ = 8.2° (311 reflection) as opposed to two peaks observed at 2θ = 8.3° and 8.7° in the reported Zr-trimesate MOF (MOF-808).17 The broadening of the diffraction peaks was due to the low crystallinity or amorphous nature of the synthesized MOF. In the present study, the use of ZrOCl2·8H2O as the Zr precursor was responsible for the low crystallinity of the MOF, which matched with the previously reported work of Ardila-Suárez and co-workers.18 In the PXRD pattern of Fe3O4, the peaks observed at 2θ = 30.2°, 35.5°, 43.2°, 53.3°, and 57.1° were assigned to the 220, 311, 400, 422, and 511 reflections, respectively, of the cubic inverse spinel structure of the Fe3O4 phase (JCPDS card#19-0629).19 The peak for Zr-MOF (2θ = 7.9°), the amorphous feature, and crystalline Bragg reflections of Fe3O4 is distinctly visible in the diffraction pattern of Zr-MOF@Fe3O4, which further confirmed the formation of the magnetic Zr-MOF composite.
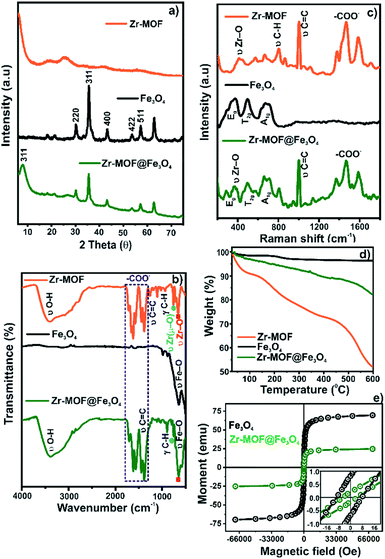 |
| Fig. 2 (a) PXRD patterns; (b) Raman spectra; (c) FTIR spectra; (d) TGA profiles; (e) VSM curves of Zr-MOF, Fe3O4, and Zr-MOF@Fe3O4. | |
The FTIR spectra of Zr-MOF, Fe3O4, and Zr-MOF@Fe3O4 are shown in Fig. 2b. The spectrum of the Zr-MOF shows a broad band at 3397 cm−1 for OH stretching vibrations of Zr–OH and adsorbed water molecules. The band at 1707 cm−1 was due to the unreacted –COOH groups in the MOF. The bands at 1619 and 1387 cm−1 were assigned to the asymmetric and symmetric stretching vibrations of Zr-bound carboxylate groups after the coordination process. The band at 1658 cm−1 was attributed to the O–H bending mode of adsorbed water molecules and carbonyl stretching vibrations of guest DMF molecules. The band at 1446 cm−1 was the characteristic band for C
C stretching in the aromatic rings of the ligand.20,21 Bands observed in the 1200–1000 and 1000–700 cm−1 range were assigned to the in-plane and out-of-plane C–H bending modes, respectively.22 The bands at 647 and 460 cm−1 were attributed to the stretching modes of the Zr–(μ3-O) bridges and Zr–O (carboxylate) bonds in the MOF structural framework, respectively.23 The intense band at 582 cm−1 in the FTIR spectrum of Fe3O4 was assigned to the O–Fe stretching vibrations.24 These characteristic bands of Zr-MOF and Fe3O4 were distinctly visible in the composite, with a slight shift in the energy for carboxylate bands probably due to the weak interaction between the constituents, which confirmed the formation of the magnetic composite.
The Raman spectra of the Zr-MOF, Fe3O4, and Zr-MOF@Fe3O4 are shown in Fig. 2c. In the spectrum of the Zr-MOF, the peak at 413 cm−1 was related to the Zr–O (carboxylate) stretching modes. The peaks at 803 and 868 cm−1 were assigned to the C–H bending modes, and the intense 1005 cm−1 peak was associated with the C
C stretching vibrations in the aromatic rings. The peaks at 1589 and 1469 cm−1 were characteristic of asymmetric and symmetric stretching modes of carboxylate bridges, respectively.25,26 In Fe3O4, three bands were observed, where the bands at 374 and 498 cm−1 were assigned to the Eg and T2g(2) modes for the symmetric and asymmetric bending of oxygen (concerning Fe) in the tetrahedral void, respectively. The band at 675 cm−1 was due to the A1g mode for the symmetric stretching of the oxygen atoms in the tetrahedral FeO4 groups.16 The spectral features of Fe3O4 and Zr-MOF were observed in the Raman spectrum of the Zr-MOF@Fe3O4 composite.
The TGA profile of materials was recorded in the range of 30–600 °C (Fig. 2d). In the TGA profile of Zr-MOF, three stages of mass loss were noticed in the studied temperature range. The first mass loss, recorded between 30 and 110 °C, was due to the evaporation of physically adsorbed water molecules (9.4% of mass loss). The second mass loss range (110–400 °C, 17.8% of mass loss) was described as the loss of chemisorbed water and physisorbed solvent (DMF/methanol) molecules. The third stage of mass loss (>400 °C) was associated with the thermal degradation of the MOF due to the breakdown of metal–carboxylate coordination bonds.27,28 Thus, the Zr-MOF was thermally stable up to 400 °C. In the TGA profile of Fe3O4, an ∼1.5% mass loss below 100 °C was associated with the evaporation of water molecules. In the entire temperature range, an insignificant change in the mass loss confirmed the high thermal stability of the magnetite.29 The TGA profile of Zr-MOF@Fe3O4 showed a significantly low mass loss in the entire temperature range compared to the pristine Zr-MOF, which confirmed that the composite was more stable than the Zr-MOF.16
The VSM curves of Fe3O4 and Zr-MOF@Fe3O4 at 300 K are shown in Fig. 2e. Both materials showed superparamagnetic behaviour with a sigmoidal form, where the remanence was close to zero. The magnetization saturation (μs) value of 69.29 emu g−1 for Fe3O4 decreased to 25.02 emu g−1 for Zr-MOF@Fe3O4 (Table S2†). The decrease in the μs value of Fe3O4 after Zr-MOF addition was due to the increased thickness of the non-magnetic component.16 Though the μs value of Zr-MOF@Fe3O4 was 25.02 emu g−1, it was sufficient for rapid phase separation from large volumes of water by a strong permanent magnet.
The surface elemental composition and the oxidation states of constituent elements in the materials were confirmed by XPS analysis. The XPS surveys confirmed Zr, C, O, and Fe in the synthesized samples (Fig. 3a and Table S3†). The HRXPS C 1s spectrum of Zr-MOF@Fe3O4 has four peaks at 284.7, 285.9, 288.6, and 290.7 eV, which were assigned to the sp2 C
C (55.8%), C–O (23.4%), O–C
O (16.4), and π–π* bonding moieties (4.4%) (Fig. 3b and Table S4†).30 The HRXPS O 1s spectrum has three contributions at 530.2, 531.7, and 533.1 eV, which were due to O–Zr/O–Fe (50.4%), O–C (37.7%), and Zr–OH (11.9%), respectively (Fig. 3c and Table S5†).31,32 The HRXPS Zr 3d spectrum has two peaks at 182.9 and 185.6 eV for 3d5/2 and 3d3/2, respectively, which were assigned to the Zr4+ oxidation state in the Zr-MOF structure (Fig. 3d).33 The Zr 3d5/2 peak in Zr salt observed at 183.2 eV shifted to 182.6 eV in the Zr-MOF due to an increase in the electron density around the Zr ions after coordination with the carboxylate groups. This peak in the Zr-MOF shifted to a higher value by 0.3 eV after composite formation, suggesting the possible interaction of Zr-sites with the magnetite surface (Fig. S2 and Table S6†). The high-resolution Fe 2p2/3 spectrum of the composite was fitted using the Gupta and Sen multiplets. The resulting fit for Fe 2p3/2 confirmed the presence of Fe3O4 and FeOOH components. The Fe3+/Fe2+ ratio of 2.2
:
1 in the Zr-MOF@Fe3O4 was close to the expected ratio of 2.1
:
1 observed for the Fe3O4 phase (Fig. 3e and Table S7†).33
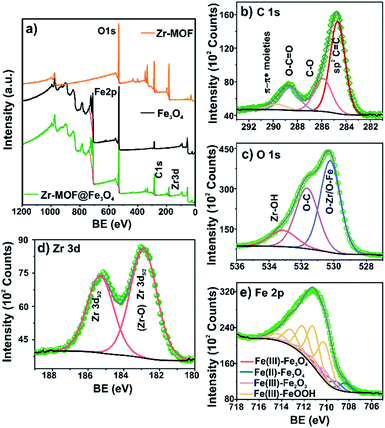 |
| Fig. 3 (a) Survey spectra of Zr-MOF, Fe3O4, and Zr-MOF@Fe3O4; high-resolution spectra of (b) C 1s; (c) O 1s; (d) Zr 3d; (e) Fe 2p for Zr-MOF@Fe3O4. | |
Parameter optimization
The Hg(II) species adsorption was studied with each component of the final material to find if the magnetic MOF has better performance than each of its components. As observed in Fig. S3,† Zr-MOF@Fe3O4 showed the best removal efficiency for Hg(II), 97.01%, while Fe3O4 and Zr-MOF showed 39.35% and 69.71% removal efficiency, respectively. The effect of pH on the Hg(II) adsorption capacity of the composite was studied in the pH 2–6 range (Fig. 4a). The adsorption capacity of 42.1 mg g−1 at pH 2 increased to 48.9 mg g−1 at pH 6. The zeta potential measurements conducted in the pH 2–6 range showed that the surface of the composite was negatively charged. The composite surface becomes more negative with the increasing pH, favouring the adsorption of positively charged Hg(II) ions,34 with the involvement of physical forces. The adsorption capacity was significantly high in the entire pH range, which suggested its wider applicability in the treatment of Hg-contaminated wastewater under different acidic/basic conditions. The adsorption capacity of the composite as a function of time is reflected in Fig. 4b. The results suggested that the Hg adsorption process occurred in two distinct steps. In the first 4 h of soaking, the adsorption capacity rapidly reached 38.4 mg g−1. This phenomenon was due to the availability of abundant binding sites for Hg(II) interaction on the adsorbent surface. This fast step was followed by a slow step where the adsorption capacity increased to 49.9 mg g−1 in the next 20 h of soaking, where 12 h of soaking was sufficient for a near-complete removal of Hg ions. The slow adsorption step was due to the non-availability of surface binding sites, which prompted diffusion of Hg(II) ions (and increased the mass transfer resistance with time) through the MOF pores for accessibility of the internal binding sites.35,36
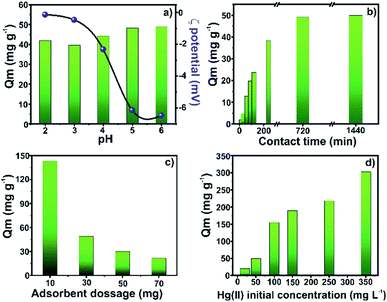 |
| Fig. 4 Effect of (a) pH; (b) contact time; (c) adsorbent dosage; (d) Hg(II) concentration on the adsorption capacity of Zr-MOF@Fe3O4. Conditions: mass of Zr-MOF@Fe3O4 = 30 mg, [Hg(II)] = 50 mg L−1, volume = 30 mL, time = 12 h (change accordingly). | |
The relationship between the adsorbent dosage and adsorption efficiency was investigated by varying the adsorbent mass (10–70 mg). As shown in Fig. 4c, the adsorption capacity of 143.1 mg g−1 for 10 mg adsorbent dropped to 21.5 mg g−1 for 70 mg of the adsorbent. The consistent fall in the adsorption capacity with increasing adsorbent mass was due to the insufficient number of Hg(II) ions for the saturation of binding sites at a higher adsorbent mass. Though the increasing mass provides more adsorption sites for high % removal, the limited concentration of Hg(II) ions at a higher dosage results in incomplete saturation of the adsorbent and low adsorption capacity.37,38 The effect of Hg(II) concentration on the adsorption capacity was studied in the range of 20–350 mg L−1 (Fig. 4d). The adsorption capacity periodically increased from 19.4 mg g−1 (20 mg L−1 solution) to 302.5 mg g−1 for an initial concentration of 350 mg L−1. The increasing concentration of Hg(II) ions in the aqueous phase for a limited number of binding sites increased the Hg(II) concentration gradient near the solid–liquid interface and improved the mass transfer process. This large Hg(II) ion availability resulted in a rapid mass transfer and improved adsorption capacity.39
Hg, Cd and Pb are known to be highly toxic to human health. The co-adsorption of Hg(II), Cd(II), and Pb(II) from a ternary metal solution was performed to evaluate the applicability of the adsorbent in removing multiple toxic metals from waste solutions. The adsorption capacity of these heavy metals in a multi-elemental study with the optimized parameters is reported in Fig. 5. The composite has a high adsorption capacity of 45.1 (90.2%), 42.9 (85.8%), and 44.2 mg g−1 (88.4%) for Hg(II), Cd(II), and Pb(II), respectively. Thus, the fabricated magnetic MOF composite is efficient in the simultaneous removal of multiple heavy metal ions.
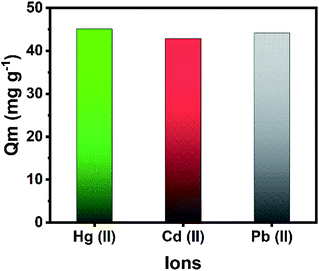 |
| Fig. 5 Simultaneous removal of Hg(II), Cd(II), and Pb(II) from a ternary metal solution. Conditions: mass of Zr-MOF@Fe3O4 = 30 mg, [ion] = 50 mg L−1 each, volume = 30 mL, time = 12 h. | |
The Hg(II) adsorption capacity of the Zr-MOF/Fe3O4 composite was compared with those of the reported Zr-based MOFs42–46 (Table S8†). In the literature, the adsorption capacity of 700–800 mg g−1 has been reported for thiol functionalized MOFs.43 But, in the present study, we have adopted a comparatively inexpensive ligand for the fabrication of a non-functionalized MOF, which could adsorb 303 mg g−1 of Hg(II) ions just upon soaking in Hg(II)-contaminated water. Moreover, easy phase separation of the magnetic Zr-MOF was an additional advantage to the material and methodology developed for the Hg(II)-remediation process.
Kinetics and isotherms
Four different kinetic models, pseudo-first (PFO), pseudo-second (PSO), Elovich, and intra-particle diffusion (IPD) models, were employed to study the kinetic mechanism and the adsorption process of Hg(II) on the Zr-MOF@Fe3O4 composite. The kinetic model equations are shown in Table S9.†Fig. 6 shows the kinetic fitting curves, and the calculated kinetic parameters are presented in Table S10.† The correlation coefficient (R2) for the Elovich model (R2 = 0.96) was higher than those for the PFO (R2 = 0.72) and PSO (R2 = 0.95) models. Although the Elovich equation provided the best correlation for the sorption process, the experimental data were also fitted in the PSO model. Thus, the adsorption kinetics could be explained by both the Elovich and the PSO kinetic models. Furthermore, the correlation with the PSO model indicated that the adsorption process was mainly controlled by chemisorption, involving valency forces through sharing or exchange of electrons between the metal ions and the adsorbent sites.40,47 Besides, the Elovich model gave a good correlation for the adsorption on highly heterogeneous surfaces. Moreover, this model showed that chemisorption was a dominant phenomenon along with surface adsorption. But, in a highly heterogeneous system with surface adsorption, chemisorption, ion exchange, precipitation, and intra-particle diffusion processes occur concurrently.47 Since the modified Zr-based MOF is a porous material with a heterogeneous surface and various active adsorption sites, the kinetic data were fitted to the IPD model to study the role of diffusion in the Hg(II) adsorption process. It is well known that the intra-particle diffusion process consists of three steps: mass transfer, adsorption, and diffusion.16,44 The data were fitted in two regions, which were regarded as two adsorption processes (Fig. 6d). The calculated rate constant (Kip) was in the order of Kip1 > Kip2 (Table S10†). In the first step (Kip1), the adsorption process started with Hg(II) ions migrating from the bulk aqueous phase to the Zr-MOF@Fe3O4 surface. The second step (Kip2) occurred after the saturation of the external surface. After saturation of external sites, Hg(II) ions entered the MOF pores with increasing resistance to diffusion. The Hg(II) diffusion into the pores of the Zr-MOF was limited or partially hindered due to Zr-MOF cavity modification with Fe3O4 nanoparticles. In this study, the mass transfer process was completed in a short time; thus, the ion migration process (first step) and adsorption process (second step) were the main steps of intra-particle diffusion. The data showed that the restrictive step of Hg ion removal by Zr-MOF@Fe3O4 was the adsorption process, not the diffusion process.
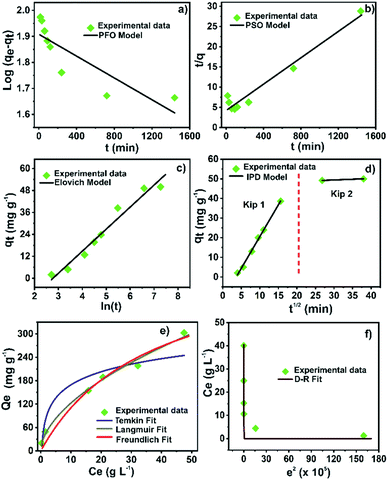 |
| Fig. 6 (a) PFO; (b) PSO; (c) Elovich; (d) IPD kinetic model, (e) Langmuir, Freundlich, and Temkin isotherm fits; (f) Dubinin–Radushkevich isotherm for Hg(II) adsorption onto Zr-MOF@Fe3O4. | |
The trend between the adsorption amount and the metal ion concentration and how the metal ions interact with the adsorbent were studied using adsorption isotherms. Langmuir, Freundlich, Dubinin–Radushkevich and Temkin isotherm models were applied to analyze the experimental adsorption data. The non-linear adsorption isotherm equations are presented in Table S11.† The curves fitted by the models are shown in Fig. 6e and f. The calculated parameters from models are summarized in Table S12.† The correlation coefficient (R2) of the Freundlich model (0.99) was higher than those of the Langmuir (0.97), D–R (0.95), and Temkin (0.88) models, indicating that the adsorption process was multilayer adsorption.16,41 This suggested that the physicochemical forces over the MOF surface play the primary role in the Hg(II) adsorption. The separation factor (RL) computed from the Langmuir model remained between 0 and 1, suggesting a favourable Hg(II) adsorption process over Zr-MOF@Fe3O4. Moreover, the free Gibbs energy value was negative, which confirmed the spontaneity of the adsorption process.48 Additionally, the nature of the Hg(II) adsorption process, either physisorption or chemisorption, can be distinguished from the D–R model's free energy (E). For E < 8 kJ mol−1, the sorption proceeds by physisorption, while for E ∼ 8–16 kJ mol−1, the sorption process is driven by chemical forces.49 The free energy from the D–R model was 9.2 kJ mol−1, which indicated that the Hg(II) adsorption process over Zr-MOF@Fe3O4 was multilayer adsorption driven by physicochemical forces.
Adsorption mechanism
The FTIR spectra of fresh and spent Zr-MOF@Fe3O4 are shown in Fig. 7a. The intensity of the band at 1707 cm−1 for free –COOH groups diminished after Hg(II) adsorption. The decreased intensity was due to Hg(II) ion binding with the free carboxylic group. This Hg(II)-bound carboxylate group was observed as a new band at 1653 cm−1. The band at 3397 cm−1 for OH stretching in adsorbed water molecules and Zr–OH groups lost intensity in the Hg(II)-adsorbed MOF. The decreased intensity was due to the interaction of Hg(II) ions with the Zr–OH groups upon deprotonation at pH 6. Thus, the FTIR predicted the involvement of free –COOH and Zr–OH groups in the adsorption of Hg(II) ions. The XPS analysis of the spent adsorbent confirmed the presence of 0.7% of Hg ions (Table S3†). The HRXPS Hg 4f spectrum has a peak at 100.8 eV, which was assigned to the Hg 4f7/2 for Hg(II) ions (Fig. 7b).50 The HRXPS Zr 3d spectrum of the spent adsorbent has two peaks at 183.2 and 185.6 eV, assigned to the 3d5/2 and 3d3/2 peaks, respectively. The Zr 3d5/2 peak in the fresh adsorbent was observed at 182.9 eV. The blue shift of 0.3 eV in the binding energy of the Zr 3d5/2 peak was due to the change in the electron density around Zr after interaction of Hg(II) ions with the deprotonated Zr–OH sites (Fig. 7c). Thus, free carboxylic acid groups and Zr–OH were responsible for Hg(II) adsorption over the magnetic Zr-MOF. This peak was absent in the pristine sample, which confirmed the Hg(II) adsorption process over the magnetic MOF composite (Fig. 7d).
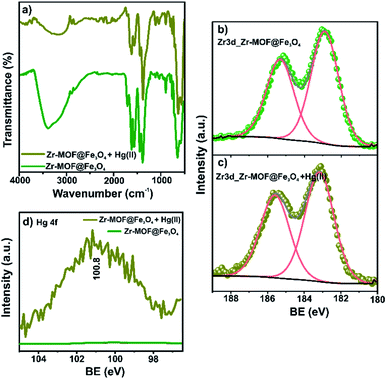 |
| Fig. 7 (a) FTIR spectra; XPS Zr 3d high-resolution signals before (b) and after (c) Hg(II) adsorption, (d) Hg 4f high-resolution signals before and after Hg(II) adsorption. | |
Reusability
For practical applications, reusability is a crucial factor, which profoundly impacts the sustainability and affordability of the developed adsorbent. In the present study, we have adopted the use of 0.1 mol L−1 NaOH (Fig. 8a) or HCl (Fig. 8b) solution for stripping Hg(II) ions from the loaded composite. The sorbed Hg(II) ions could be stripped using strong acidic solutions due to the competitive nature of protons for the adsorption sites.51,52 In the first cycle, the regeneration of the spent composite using the HCl solution desorbed 72% of the adsorbed Hg(II) ions. The desorption efficiency remained in the 63–71% range for the next four cycles. The partial desorption using HCl was due to the significantly high adsorption capacity of the composite at pH 2, which severely limited the desorption process at pH 1. Though the desorption efficiency was less satisfactory, the adsorption capacity of 49.2 mg g−1 in the first cycle reached 44.7 mg g−1 in the fifth cycle (∼10% drop), suggesting appreciable reusability of the composite. In the reported work on Hg(II) adsorption on Zr-based MOFs, it has been observed that the adsorption capacity tends to drop in a basic solution.40,44 Thus, the use of a highly basic eluent solution could be effective in the regeneration of the spent adsorbent. The pH 13 solution was highly effective in stripping sorbed Hg(II) ions, where the desorption efficiency remained in the range of 94–97% for five cycles. Moreover, the adsorption capacity of 49.2 mg g−1 in the first cycle dropped by ∼1.8% in the fifth cycle. Thus, it was evident that the NaOH solution was highly efficient for regeneration purposes.
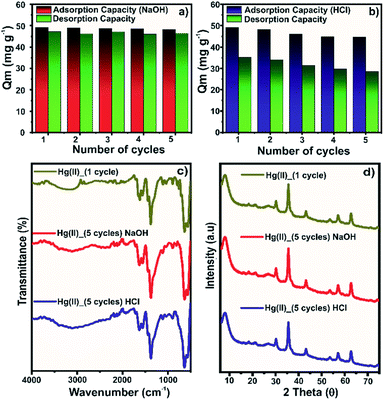 |
| Fig. 8 Regeneration and reusability tests of Zr-MOF@Fe3O4 for the removal of Hg(II) with (a) NaOH eluent and (b) HCl eluent; (c) FTIR spectra; (d) PXRD patterns of Zr-MOF@Fe3O4 after reusability tests. Conditions: mass of Zr-MOF@Fe3O4 = 30 mg, [Hg(II)] = 50 mg L−1, [NaOH]/[HCl] = 0.1 mol L−1, volume = 30 mL, time = 12 h. | |
The material stability after five adsorption–desorption cycles was assessed by FTIR (Fig. 8c) and PXRD (Fig. 8d) analysis. In the FTIR spectra of regenerated samples, an insignificant change in the band intensity was observed. Only the band at ∼1620 cm−1 for asymmetric stretching of the Zr–COO linkage showed a slight decrease in the intensity, probably due to the breakage of some coordinative bonds. The PXRD diffraction patterns of regenerated samples for five cycles were identical to those of the sample of the first adsorption cycle. Thus, the spectroscopic evidence further confirmed the stability of the composite for multiple Hg(II) adsorption–desorption cycles.
Conclusions
In this work, we have synthesized a magnetic Zr-MOF to remove toxic metal ions from water. The synthesis of Zr-MOF@Fe3O4 was confirmed through microscopic and spectroscopic techniques such as SEM, FT-IR, Raman, DRX, TGA, and VSM. The Zr-MOF@Fe3O4 showed the maximum Hg(II) uptake capacity of 303 mg g−1, which was higher than that of the reported Zr-MOF in the literature, and with an easy phase separation process after adsorption. The Hg(II) adsorption process was driven by physicochemical forces, which were inferred from the fitting of kinetic and adsorption data in the Elovich and Langmuir model, respectively. The mechanism of adsorption was identified by FTIR and XPS analyses, which confirmed the involvement of –COOH and Zr–OH groups in the Hg(II) adsorption process. Zr-MOF@Fe3O4 showed a simultaneous high uptake capacity of 45.1, 42.9, and 44.2 mg g−1 for Hg(II), Cd(II), and Pb(II), respectively in a multi-element study. The spent MOF was successfully regenerated by 0.1 mol L−1 NaOH solution with the retention of 90% of its initial adsorption capacity after 5 cycles. Thus, the study demonstrated a novel magnetic Zr-MOF as a potential candidate for decontaminating heavy metal-rich wastewater.
Author contributions
R. P. oversaw data curation, formal analysis, and methodology. H. V. and N. K. G. oversaw conceptualization, visualization, software, validation, writing – original draft, and writing – review & editing. A. R.-G. and C. L. were responsible for funding acquisition, investigation, project administration, resources, and supervision.
Conflicts of interest
There are no conflicts to declare.
Acknowledgements
The authors would like to thank Laboratorio Nacional de Ciencia, Tecnología y Gestión Integrada del Agua (LNAGUA) and LNCAE (Laboratorio Nacional de Conversión y Almacenamiento de Energía) for their experimental and instrumental facilities.
References
- Y. Y. Xiong, J. Q. Li, L. Le Gong, X. F. Feng, L. N. Meng, L. Zhang, P. P. Meng, M. B. Luo and F. Luo, Using MOF-74 for Hg2+ removal from ultra-low concentration aqueous solution, J. Solid State Chem., 2017, 246, 16–22 CrossRef CAS.
- P. B. Tchounwou, W. K. Ayensu, N. Ninashvili and D. Sutton, Review: Environmental exposure to mercury and its toxicopathologic implications for public health, Environ. Toxicol., 2003, 18, 149–175 CrossRef CAS.
- G. K. Kinuthia, V. Ngure, D. Beti, R. Lugalia, A. Wangila and L. Kamau, Levels of heavy metals in wastewater and soil samples from open drainage channels in Nairobi, Kenya: community health implication, Sci. Rep., 2020, 10, 8434 CrossRef CAS PubMed.
- W. Yang, K. Cheng, R. Tang, S. Wu, H. Wang, Z. Han, E. Liu, S. Zhu and L. Che, Ion exchange resin derived magnetic activated carbon as recyclable and regenerable adsorbent for removal of mercury from flue gases, J. Energy Inst., 2021, 97, 225–232 CrossRef CAS.
- S. Azat, E. Arkhangelsky, T. Papathanasiou, A. A. Zorpas, A. Abirov and V. J. Inglezakis, Synthesis of biosourced silica–Ag nanocomposites and amalgamation reaction with mercury in aqueous solutions, C. R. Chim., 2020, 23, 77–92 CrossRef CAS.
- Q. Zhang, N. Liu, Y. Cao, W. Zhang, Y. Wei, L. Feng and L. Jiang, A facile method to prepare dual-functional membrane for efficient oil removal and in situ reversible mercury ions adsorption from wastewater, Appl. Surf. Sci., 2018, 434, 57–62 CrossRef CAS.
- A. Pohl, Removal of heavy metal ions from water and wastewaters by sulfur-containing precipitation agents, Water, Air, Soil Pollut., 2020, 231, 1–17 CrossRef.
- L. Hao, N. Wang, C. Wang and G. Li, Arsenic removal from water and river water by the combined adsorption-UF membrane process, Chemosphere, 2018, 202, 768–776 CrossRef CAS.
- Z. Liu, D. Liu, B. Zhao, L. Feng, M. Ni and J. Jin, Mercury removal based on adsorption and oxidation by fly ash: A review, Energy Fuels, 2020, 34, 11840–11866 CrossRef CAS.
- N. K. Gupta and A. Gupta, 2D and 3D carbon-based adsorbents for an efficient removal of HgII ions: A review, FlatChem, 2018, 11, 1–14 CrossRef CAS.
- H. Viltres, Y. C. López, N. K. Gupta, C. Leyva, R. Paz, A. Gupta and A. Sengupta, Functional metal-organic frameworks for metal removal from aqueous solutions, Sep. Purif. Rev., 2022, 51, 78–99 CrossRef CAS.
- S. Ploychompoo, J. Chen, H. Luo and Q. Liang, Fast and efficient aqueous arsenic removal by functionalized MIL-100(Fe)/rGO/δ-MnO2 ternary composites: Adsorption performance and mechanism, J. Environ. Sci., 2020, 91, 22–34 CrossRef.
- Q. Liu, Q. Zhang, B. Liu and J. Ma, A new synthesis and adsorption mechanism of ZrO2 based metal-organic frames for efficient removal of mercury ions from aqueous solution, Ceram. Int., 2019, 45, 15720–15724 CrossRef CAS.
- Y. Peng, H. Huang, Y. Zhang, C. Kang, S. Chen, L. Song, D. Liu and C. Zhong, A versatile MOF-based trap for heavy metal ion capture and dispersion, Nat. Commun., 2018, 91, 187 CrossRef PubMed.
- J. E. Efome, D. Rana, T. Matsuura and C. Q. Lan, Insight studies on metal-organic framework nanofibrous membrane adsorption and activation for heavy metal ions removal from aqueous solution, ACS Appl. Mater. Interfaces, 2018, 10, 18619–18629 CrossRef CAS PubMed.
- R. Paz, H. Viltres, N. K. Gupta and C. Leyva, Fabrication of magnetic cerium-organic framework-activated carbon composite for charged dye removal from aqueous solutions, J. Mol. Liq., 2021, 337, 116578 CrossRef CAS.
- H. Furukawa, F. Gándara, Y.-B. Zhang, J. Jiang, W. L. Queen, M. R. Hudson and O. M. Yaghi, Water adsorption in porous metal–organic frameworks and related materials, J. Am. Chem. Soc., 2014, 136, 4369–4381 CrossRef CAS.
- C. Ardila-Suárez, J. Rodríguez-Pereira, V. G. Baldovino-Medrano and G. E. Ramírez-Caballero, An analysis of the effect of zirconium precursors of MOF-808 on its thermal stability, and structural and surface properties, CrystEngComm, 2019, 21, 1407–1415 RSC.
- A. Rajan, M. Sharma and N. K. Sahu, Assessing magnetic and inductive thermal properties of various surfactants functionalised Fe3O4 nanoparticles for hyperthermia, Sci. Rep., 2020, 10, 15045 CrossRef PubMed.
- S. Biswas, T. Ahnfeldt and N. Stock, New functionalized flexible Al-MIL-53-X (X = -Cl, -Br, -CH3, -NO2, -(OH)2) solids: Syntheses, characterization, sorption, and breathing behavior, Inorg. Chem., 2011, 50, 9518–9526 CrossRef CAS.
- J. Gong, M. J. Katz and F. M. Kerton, Catalytic conversion of glucose to 5-hydroxymethylfurfural using zirconium-containing metal–organic frameworks using microwave heating, RSC Adv., 2018, 8, 31618–31627 RSC.
- N. K. Gupta, S. Kim, J. Bae and K. S. Kim, Chemisorption of hydrogen sulfide over copper-based metal–organic frameworks: methanol and UV-assisted regeneration, RSC Adv., 2021, 11, 4890–4900 RSC.
- C. Ardila-Suárez, A. M. Díaz-Lasprilla, L. A. Díaz-Vaca, P. B. Balbuena, V. G. Baldovino-Medrano and G. E. Ramírez-Caballero, Synthesis, characterization, and post-synthetic modification of a micro/mesoporous zirconium–tricarboxylate metal–organic framework: towards the addition of acid active sites, CrystEngComm, 2019, 21, 3014–3030 RSC.
- V. A. J. Silva, P. L. Andrade, M. P. C. Silva, A. D. Bustamante, L. De Los Santos Valladares and J. Albino Aguiar, Synthesis and characterization of Fe3O4 nanoparticles coated with fucan polysaccharides, J. Magn. Magn. Mater., 2013, 343, 138–143 CrossRef CAS.
- M. Todaro, A. Alessi, L. Sciortino, S. Agnello, M. Cannas, F. M. Gelardi and G. Buscarino, Investigation by Raman spectroscopy of the decomposition process of HKUST-1 upon exposure to air, J. Spectrosc., 2016, 2016, 8074297 Search PubMed.
- K. Doloi, A. Kumar and M. N. Rao, Study of polymerization dynamics in micropores of metal-organic framework, AIP Conf. Proc., 2020, 2265, 030013 CrossRef CAS.
- K. Knozowska, R. Thür, J. Kujawa, I. Kolesnyk, I. F. J. Vankelecom and W. Kujawski, Fluorinated MOF-808 with various modulators to fabricate high-performance hybrid membranes with enhanced hydrophobicity for organic-organic pervaporation, Sep. Purif. Technol., 2021, 264, 118315 CrossRef CAS.
- E. Plessers, G. Fu, C. Y. X. Tan, D. E. De Vos and M. B. J. Roeffaers, Zr-based MOF-808 as Meerwein–Ponndorf–Verley reduction catalyst for challenging carbonyl compounds, Catalysts, 2016, 6, 104 CrossRef.
- E. T. Tenório-Neto, T. Jamshaid, M. Eissa, M. H. Kunita, N. Zine, G. Agusti, H. Fessi, A. E. El-Salhi and A. Elaissari, TGA and magnetization measurements for determination of composition and polymer conversion of magnetic hybrid particles, Polym. Adv. Technol., 2015, 26, 1199–1208 CrossRef.
- A. E. Baumann, J. R. Downing, D. A. Burns, M. C. Hersam and V. S. Thoi, Graphene–metal–organic framework composite sulfur electrodes for Li–S batteries with high volumetric capacity, ACS Appl. Mater. Interfaces, 2020, 12, 37173–37181 CrossRef CAS.
- R. Xu, Q. Ji, P. Zhao, M. Jian, C. Xiang, C. Hu, G. Zhang, C. Tang, R. Liu, X. Zhang and J. Qu, Hierarchically porous UiO-66 with tunable mesopores and oxygen vacancies for enhanced arsenic removal, J. Mater. Chem. A, 2020, 8, 7870–7879 RSC.
- N. K. Gupta, Y. Ghaffari, J. Bae and K. S. Kim, Synthesis of coral-like α-Fe2O3 nanoparticles for dye degradation at neutral pH, J. Mol. Liq., 2020, 301, 112473 CrossRef CAS.
- A. P. Grosvenor, B. A. Kobe, M. C. Biesinger and N. S. McIntyre, Investigation of multiplet splitting of Fe 2p XPS spectra and bonding in iron compounds, Surf. Interface Anal., 2004, 36, 1564–1574 CrossRef CAS.
- S. Pourbeyram, Effective removal of heavy metals from aqueous solutions by graphene oxide–zirconium phosphate (GO–Zr-P) nanocomposite, Ind. Eng. Chem. Res., 2016, 55, 5608–5617 CrossRef CAS.
- Y. Liu, X. Liu, W. Dong, L. Zhang, Q. Kong and W. Wang, Efficient adsorption of sulfamethazine onto modified activated carbon: A plausible adsorption mechanism, Sci. Rep., 2017, 7, 12437 CrossRef.
- N. K. Gupta, M. Saifuddin, S. Kim and K. S. Kim, Microscopic, spectroscopic, and experimental approach towards understanding the phosphate adsorption onto Zn–Fe layered double hydroxide, J. Mol. Liq., 2020, 297, 111935 CrossRef CAS.
- R. S. El-Tawil, S. T. El-Wakeel, A. E. Abdel-Ghany, H. A. M. Abuzeid, K. A. Selim and A. M. Hashem, Silver/quartz nanocomposite as an adsorbent for removal of mercury (II) ions from aqueous solutions, Heliyon, 2019, 5, E02415 CrossRef.
- A. Gupta, H. Viltres and N. K. Gupta, Sono-adsorption of organic dyes onto CoFe2O4/Graphene oxide nanocomposite, Surf. Interfaces, 2020, 20, 100563 CrossRef CAS.
- N. Assaad, G. Sabeh and M. Hmadeh, Defect control in Zr-based metal-organic framework nanoparticles for arsenic removal from water, ACS Appl. Nano Mater., 2020, 3, 8997–9008 CrossRef CAS.
- Z. Seyfi Hasankola, R. Rahimi, H. Shayegan, E. Moradi and V. Safarifard, Removal of Hg2+ heavy metal ion using a highly stable mesoporous porphyrinic zirconium metal-organic framework, Inorg. Chim. Acta, 2020, 501, 119264 CrossRef CAS.
- Y. Zhao, D. Wang, W. Wei, L. Cui, C.-W. Cho and G. Wu, Effective adsorption of mercury by Zr(IV)-based metal-organic frameworks of UiO-66-NH2 from aqueous solution, Environ. Sci. Pollut. Res., 2020, 28, 7068–7075 CrossRef PubMed.
- S. Chen, F. Feng, S. Li, X.-X. Li and L. Shu, Metal-organic framework DUT-67 (Zr) for adsorptive removal of trace Hg2+ and CH3Hg+ in water, Chem. Speciation Bioavailability, 2018, 30, 99–106 CrossRef CAS.
- P. Yang, Y. Shu, Q. Zhuang, Y. Li and J. Gu, A robust MOF-based trap with high-density active alkyl thiol for the super-efficient capture of mercury, Chem. Commun., 2019, 55, 12972–12975 RSC.
- L. Fu, S. Wang, G. Lin, L. Zhang, Q. Liu, J. Fang, C. Wei and G. Liu, Post-functionalization of UiO-66-NH2 by 2,5-Dimercapto-1,3,4-thiadiazole for the high efficient removal of Hg(II) in water, J. Hazard. Mater., 2019, 368, 42–51 CrossRef CAS.
- J. Li, Y. Liu, Y. Ai, A. Alsaedi, T. Hayat and X. Wang, Combined experimental and theoretical investigation on selective removal of mercury ions by metal organic frameworks modified with thiol groups, Chem. Eng. J., 2018, 354, 790–801 CrossRef CAS.
- L. Ding, X. Luo, P. Shao, J. Yang and D. Sun, Thiol-functionalized Zr-based metal–organic framework for capture of Hg(II) through a proton exchange reaction, ACS Sustainable Chem. Eng., 2018, 6, 8494–8502 CrossRef CAS.
- K. Riahi, S. Chaabane and B. Ben Thayer, A kinetic modeling study of phosphate adsorption onto Phoenix dactylifera L. date palm fibers in batch mode, J. Saudi Chem. Soc., 2017, 21, S143–S152 CrossRef CAS.
- M. Chiban, G. Carja, G. Lehutu and F. Sinan, Equilibrium and thermodynamic studies for the removal of As(V) ions from aqueous solution using dried plants as adsorbents, Arabian J. Chem., 2016, 9, S988–S999 CrossRef CAS.
- N. K. Gupta, A. Gupta, P. Ramteke, H. Sahoo and A. Sengupta, Biosorption-a green method for the preconcentration of rare earth elements (REEs) from waste solutions: A review, J. Mol. Liq., 2019, 274, 148–164 CrossRef CAS.
- V. Chandra and K. S. Kim, Highly selective adsorption of Hg2+ by a polypyrrole–reduced graphene oxide composite, Chem. Commun., 2011, 47, 3942–3944 RSC.
- S. Venkateswarlu and M. Yoon, Rapid removal of cadmium ions using green-synthesized Fe3O4 nanoparticles capped with diethyl-4-(4 amino-5-mercapto-4H-1,2,4-triazol-3-yl)phenyl phosphonate, RSC Adv., 2015, 5, 65444–65453 RSC.
- Z. Ramezani, N. Pourmand, A. Behfar and A. Momeni, Removal and recovery of mercury from chlor-alkali petrochemical wastes using γ-Fe2O3 nanoparticles, Appl. Petrochem. Res., 2016, 6, 403–411 CrossRef CAS.
|
This journal is © The Royal Society of Chemistry 2022 |