Greenhouse gas and air pollutant emissions from power barges (powerships)†
Received
21st December 2021
, Accepted 8th March 2022
First published on 21st March 2022
Abstract
Power barges or powerships that operate on natural gas (NG) are an increasingly appealing easy-to-use solution to electricity deficits in Africa, Asia, the Middle East, and the Caribbean. Global generating capacity has increased from 0.1 to 2.6 GW, and 4.4 GW is under construction. South Africa has licensed three powerships to provide 1.2 GW generating capacity with foreign liquefied NG (LNG) over 20 years. To understand the importance of this source, we estimate lifecycle emissions of GHGs and air pollutants for South Africa and extend this to the global fleet. Annual lifecycle GHG emissions for 1.2 GW generating capacity total 2.6–3.8 Tg carbon dioxide equivalents (CO2e) using 100 year global warming potentials (GWPs). This increases to 4.0–7.1 Tg CO2e using 20 year GWPs, due to the potency of fugitive methane (CH4). Adoption of air pollutant emission control technology will need to be enforced to achieve compliance with national standards for fine particles (PM) and nitrogen oxides (NOx). A global fleet of 7.0 GW generating capacity reliant on domestic NG could emit 12 Tg CO2, 2.2–8.6 Tg CH4, 4.3 Gg NOx, and 2.6 Gg PM. Additional NOx and SO2 emissions would result from imported LNG, as LNG tankers burn dirty fuel oil, though SO2 emissions may be curtailed with recent stricter limits on the fuel sulfur content. These powerships could have important regional impacts, but emission estimates are uncertain. Characteristic emission factors, detailed operating conditions, and NG composition data are urgently needed to address uncertainties in emissions for air quality and climate modelling of this emergent source.
Environmental significance
Powerships that run on natural gas are an appealing solution to electricity deficits, as these can easily connect to the national power grid. Global generating capacity has increased 17-fold since 2010. We obtain the first theoretical estimate of air pollution and greenhouse gas emissions from the global fleet. Greenhouse gas emissions are only less than those from coal-based energy if methane leakage is curtailed. Air pollutant emissions are substantial if liquefied natural gas is obtained from distant locations, necessitating transport by tankers that burn heavy fuel oil. Our estimates are obtained with limited activity and emission factor data specific to powerships. Such information is crucial for determining the influence of this emerging source on the climate, air quality, and atmospheric chemistry.
|
1. Introduction
Floating power barges or powerships are increasingly used as an off-the-shelf solution to energy supply deficits in countries with limited land-based energy generating infrastructure and to circumvent lengthy lead times for constructing and commissioning new power plants. These moor at ports and connect directly to the power grid. Powerships have been deployed to provide energy to coastal and inland countries in Africa (Ghana, Senegal, Zambia via Mozambique, Sudan, Sierra Leone, Guinea-Bissau, and Gambia), the Middle East (Iraq and Lebanon), Asia (Pakistan and Indonesia) and the Caribbean (Cuba). In some of these countries, powerships account for all (Guinea-Bissau), most (60% for Gambia), or a large portion (∼25% for Ghana and Lebanon) of the electricity generated. Globally, generating capacity from powerships has increased 17-fold from a modest 144 MW in 2010 to more than 2.6 GW in 2020. An additional 4.4 GW is under construction (http://www.karpowership.com/en/; accessed 24 August 2021). To ease routine power outages, South Africa recently licensed three powerships with a combined generating capacity of 1.2 GW (two at 450 MW and one at 320 MW), despite not being granted environmental approval.1
Little is known about the potential emissions of health-hazardous air pollutants and climate-altering greenhouse gases (GHGs) from powerships.2 Powerships are reciprocating engines that run on either natural gas (NG) or heavy fuel oil (HFO) commonly used by ships over the ocean. Most of the current fleet uses NG from domestic reserves, after originally using HFO. NG for the powerships planned for deployment in South Africa will be shipped as liquefied natural gas (LNG) supplied exclusively by Shell.3 Reliance on distant LNG would lead to additional air pollutants and GHG emissions from fugitive emissions of methane (CH4) during upstream extraction and processing of NG, from combustion of NG to supply energy for liquefaction and regasification, and from combustion of a combination of NG and HFO for tanker transport of LNG.2,4–8
Here we conduct the first theoretical estimate of air pollutants and GHG emissions from NG fuelled powerships, focusing our method development on the 20 year adoption of powerships by South Africa. We then extend this to the global fleet of currently deployed powerships, powerships under construction, and a speculative scenario of widespread use by all other Sub-Saharan African countries to ease electricity shortages in the region.
2. Methods
Emissions of GHGs and air pollutants are calculated for each major lifecycle step. These include upstream extraction and processing, transmission and storage, liquefaction (gas cooled to −162 °C), LNG tanker transport, regasification, and eventual NG combustion for the conversion of gas to electricity by the powerships. We use a standard approach9 to estimate emissions: | 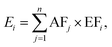 | (1) |
where Ei is the mass of the emitted pollutant or GHG i summed over each process j, AFj is the activity factor for process j, and EFi is the emission factor of GHG or air pollutant i. Values of EFi for combustion are in mass units emitted per unit energy produced or required or per unit fuel consumed. Values of EFi for CH4 leakage losses are in percent, by volume, of NG used. AFj values are determined as energy required (in MWh) for liquefaction, LNG tanker transport, and regasification, electric energy produced by the powerships (in TWh), and total NG used (in m3) for CH4 losses. NG provides energy for liquefaction, regasification and the LNG tanker outward journey. The LNG tanker uses HFO on its return.
GHG emissions from LNG processing and powerships are dominated by carbon dioxide (CO2) and CH4, but also include smaller contributions from the much more potent GHGs nitrous oxide (N2O) and sulfur hexafluoride (SF6). Total GHG emissions are calculated in CO2-equivalents (CO2e) using 20 and 100 year time horizons for global warming potential. These are 72 for CH4, 289 for N2O, and 16
300 for SF6 on 20 year timescales and 25 for CH4, 298 for N2O, and 22
800 for SF6 on 100 year timescales.10 Emissions of air pollutants hazardous to health and the environment that are regulated by South Africa through the National Environmental Management Air Quality Act of 2004 (ref. 11) include nitrogen oxides (NOx ≡ NO + NO2), sulfur dioxide (SO2), and total particulate matter (PM). Other air pollutants that will also result from NG and HFO combustion include carbon monoxide, non-methane volatile organic compounds, ammonia, and the heavy metals mercury, lead, and cadmium.12,13
We compile EFs for input to eqn (1) for NG and HFO combustion from international reports and peer-reviewed publications. These are representative of uncontrolled conditions and are summarized in Tables S1 and S2.† Air pollution EFs for gas-powered reciprocating engines are limited, so we supplement these with values for gas turbines. There are no specific EFs for the few gas turbines that operate in South Africa. The NG end use market in South Africa is small (1–3% of total fossil fuel energy) compared to end use of coal (>60%) and is dominated by industrial use for synthesizing liquid fuels.14 This may change with the discovery of shale gas in the Karoo Basin.15 Reported CH4 loss rates across the whole supply chain vary widely, due to dependence on operating conditions.16 To account for this variability, we estimate the range of these at each relevant stage of the lifecycle using reported loss rate ranges, where available. These include 0.7–4% for upstream activities,17 and 0.3–1% for transmission, distribution, and storage across the whole supply chain.17 Information for liquefaction is limited to a single value of 1.1%.18 There are no reported values for regasification, so we assume no losses for this stage.19 Losses during tanker transport are assumed negligible, as most of the boil-off-gas is used to power the ship on the outward journey.17,20 CH4 losses for the full lifecycle total 2.1–6.1%, mostly (33–66%) from upstream activities, and similar to 0.5–6.2% in other lifecycle studies.4,6,19,21
The EFs we use are consistent with those compiled for the National Renewable Energy Laboratory (NREL) harmonization project16 and used as input to lifecycle analysis models such as the Greenhouse gases, Regulated Emissions, and Energy use in Transportation (GREET) model.17
We estimate lifecycle AFs from combustion of NG and HFO assuming energy is generated by the powerships 68.75% of each day (as per the licensing requirement),22 that the LNG tanker used holds ∼170
000 m3 LNG,23 that LNG is from Shell operations in Nigeria24 ∼2600 nautical miles from South African ports, that the LNG tanker travels at 20 knots25 with an energy capacity of 40 MW,26 that the energy required for liquefaction is 0.38 kWh (kg LNG)−1,27,28 and that regasification uses 2.5% LNG by volume.28 We also consider the effect of longer tanker travel distances if instead LNG is from Shell facilities further afield that already supply to Atlantic and Indian Ocean markets. These include Oman (∼5000 nautical miles) and Trinidad and Tobago (∼6700 nautical miles).29
3. Results and discussion
3.1 Emissions from adoption of powerships by South Africa
Fig. 1 summarizes the median and range (25th to 75th percentile) of collated EFs (eqn (1)) of dominant GHGs and air pollutants from combustion of NG for liquefaction, regasification, the tanker outward journey, and to generate electricity, as well as combustion of HFO for the tanker return journey. The wide range for NOx (74.4–124 g kg−1) and PM (31.7–62.7 g kg−1) from NG combustion reflects uncertainty in the combustion efficiency of powerships, in reciprocating engine EFs, and in the source and composition of NG.30 Use of gas turbine EFs likely leads to an underestimate in powership air pollutant emissions, as the reported EFs for reciprocating engines exceed those for gas turbines.13 The powership company plans to adopt selective catalytic reduction capable of converting up to 90% of NOx to N2 and three-stage filtration capable of removing most PM.31 HFO SO2 EFs in Table S2† are for fuel with a mass percent sulfur content of 4–7%, assuming complete conversion of sulfur to SO2 during combustion. As of January 2020, the International Convention for the Prevention of Pollution from Ships (MARPOL) has imposed a limit on HFO sulfur content of 0.5 mass% or 0.8–0.9 kg MWh−1;32 at least an order of magnitude less than the values shown in Fig. 1 and Table S2.†
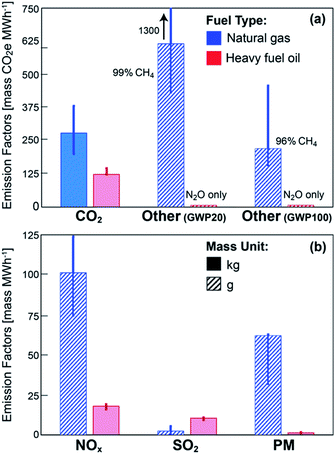 |
| Fig. 1 Emission factors of dominant GHGs (a) and regulated air pollutants (b) from the combustion of natural gas (NG, blue) and heavy fuel oil (HFO, red). The bars are medians and lines are the 25th to 75th percentile range. The values are mass per MWh energy required or generated. GHGs are in mass of CO2-equivalents (CO2e) for CO2 and others (CH4, SF6, and N2O) for 20 year (GWP20) and 100 year (GWP100) time horizons. Inset values for other NG GHG EFs give the CO2e contribution of CH4 and the 75th percentile value at GWP20. | |
The amount of electrical energy that would be generated for consistent operation over 20 years totals 147 TWh. The amount of LNG required to generate 147 TWh, for NG with an energy capacity of 4.59 kWh m−3,33 and for compression of 632.35 m3 NG to 1 m3 LNG, is 50.6 million m3. This would require a refuel rate of 66 days for the two 450 MW powerships and 94 days for the 320 MW powership, totaling 298 tanker trips.
Fig. 2 shows total emissions of dominant GHGs and air pollutants from each downstream step and gas-to-electricity conversion by the powerships over 20 years. Gas-to-electricity dominates CO2 (92% of emissions) and PM (78% of emissions). Transport is the largest source of NOx (64%) and SO2 (98%) emissions due to the combustion of HFO on the tanker return journey. If instead LNG is from facilities in Oman or Trinidad and Tobago, total air pollutant emissions increase by 60–100% for NOx to 70–88 Gg, 90–150% for SO2 to 32–42 Gg and 20–30% for PM to 14–15 Gg. If the recent international limits on the fuel sulfur content are enforced, SO2 emissions shown in Fig. 2 decline to 2–5 Gg, depending on the origin of LNG. Over the open ocean, emissions from the LNG tanker would contribute to ship traffic lane indirect and direct radiative forcing by forming the short-lived climate forcers ozone and aerosols, and by altering the radiative properties of clouds.34–38 Emissions from ships also alter the chemistry of naturally emitted compounds by perturbing the oxidative potential of the overlying atmosphere.39 There is also potential to degrade air quality at ports, though this can be prevented by enforcing international regulations on shipping emissions.40–43 CH4 emissions in Fig. 2 are almost exclusively from fugitive emissions and range from 0.5 Tg at a loss rate of 2.1% to 1.3 Tg at 6.1%. Regasification emissions are small in comparison to the other processes, though CH4 losses from this step are not known.
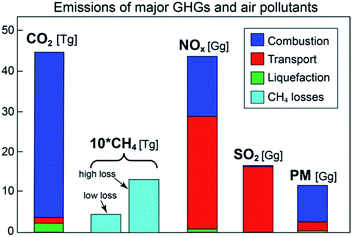 |
| Fig. 2 LNG lifecycle emissions of major GHGs and air pollutants for a 20 year adoption of powerships by South Africa. CH4 emissions are at low (2.1%) and high (6.1%) loss rates. | |
Annual GHG emissions for each of the lifecycle stages using 20 year global warming potentials are summarized in Fig. 3. These total 4.0–7.1 Tg CO2e. Totals on the 100 year time horizon are 2.6–3.8 Tg CO2e, due to the reduced potency of CH4. Gas-to-electricity is 92% of total CO2e emissions in the absence of CH4 losses and 30% of the total at a CH4 loss rate of 6.1%. The upstream, liquefaction, pipeline transmission, and storage emissions we estimate are 0.03–0.1% of Shell's total carbon footprint.44 Annual GHG emissions normalized to the amount of electrical energy generated are 540–970 kg CO2e MWh−1 on a 20 year time horizon and 350–520 kg CO2e MWh−1 on a 100 year time horizon. This represents 40–100% of the 950–1000 kg CO2 MWh−1 for South Africa's coal-fired power plants.45 The 40–100% contribution is unaffected by also accounting for national CH4 emissions from coal mining, as this source totals 72 Gg per year,46 or 41 kg CO2e MWh−1 on a 20 year time horizon.
 |
| Fig. 3 Total annual GHG emissions from each NG lifecycle stage for South Africa. Values are ranges in GHG emissions in CO2-equivalents (CO2e) on a 20 year time horizon totaling 4.0–7.1 Tg CO2e (blue) and in reported CH4 loss rates in percent per volume NG (red). | |
South Africa already relies on mostly aged and poorly regulated coal-fired power plants with a total generating capacity of 40 GW.2,45,47–50 Annual GHG emissions reported for South Africa in 2019 by the widely used global inventory Emission Database for Global Atmospheric Research (EDGAR) total 532 Tg CO2e, 46% (245 Tg CO2e) from energy generation.51 South Africa is ranked the 14th largest emitter of GHGs (https://www.carbonbrief.org/the-carbon-brief-profile-south-africa, accessed 10 August 2021), so even though the contribution of GHG emissions from gas-to-power conversion by the powerships is only 1–2% of total annual GHG emissions, South Africa's insufficient Paris Climate Accord commitments (https://climateactiontracker.org/countries/south-africa/, accessed 9 August 2021) support the need to reduce reliance on fossil fuels.
Table 1 compares the gas-to-electricity powership air pollutant emissions we obtain in mg per m3 NG to the national emission limits for NG power plants and gas-fuelled reciprocating engines. Both standards are included, as it is not evident which one will be used to regulate emissions. Powerships are technically reciprocating engines, but the powerships to be moored at ports in South Africa will exclusively use NG (synonymous with NG combustion installations). The median NOx and PM emissions in Table 1 exceed both standards, whereas SO2 (8.0–14 mg m−3) is well below both (400 and 1170 mg m−3). Successful adoption of emission control technologies should lead to near-negligible emissions of PM and NOx emissions would decline to 47 mg m−3; below the emission standard for NG combustion installations. However, our NOx emission estimate is derived from uncertain reciprocating engine EFs.
Table 1 Comparison of powership emissions to Minimum Emission Standards for South Africa
Pollutant |
Powership emissions [mg m−3] |
National emission standardsa [mg m−3] |
Median |
25th to 75th percentiles |
NG combustion installation |
Reciprocating engineb |
Standards are from the 2004 Air Quality Act11 for stationary systems designed to run on gas fuel only (NG combustion installations) and on liquid and gas fuels (reciprocating engines).
Separate reciprocating engine standards exist for liquid and gas fuels. Gas fuel values are provided.
|
PM |
285 |
146–288 |
10 |
50 |
NOx as NO2 |
465 |
342–569 |
50 |
400 |
SO2 |
11 |
8.0–14 |
400 |
1170 |
3.2 Global powership fleet emissions
Estimated annual emissions from the current global powership fleet (2.6 GW generating capacity) that relies mostly on domestic NG total 4.4 Tg CO2, 0.8–3.2 Tg CH4, 1.6 Gg NOx and 1.0 Gg PM, assuming that electricity is also generated by these 67.85% of the day and that no emission controls are adopted. Emissions increase almost 3-fold with the additional 4.4 GW powerships under construction. If all 4.4 GW powerships were to use LNG, liquefaction and regasification would add a further 0.4 Tg CO2. The LNG tanker’s contribution to air pollutant emissions may also be substantial (Fig. 2), though this depends on tanker travel distances and the HFO sulfur content.
Almost 600 million people lack access to electricity in Sub-Saharan Africa alone.52 This is a deficit of 480 GW, using South Africa's per capita installed capacity in 2010 of ∼0.8 kW.14 Based on the current powership fleet, adoption is not limited to coastal (Zambia) or affluent (Guinea-Bissau) countries. If every other country in Sub-Saharan Africa similarly adopts 1.2 GW generating capacity from powerships, this would total 54 GW and address 11% of the current deficit. GHG emissions from upstream, transmission, storage and combustion could total 110–160 Tg CO2e on a 100 year time horizon. This is 5–7% of the total reported gross GHG emissions for Sub-Saharan Africa in 2018 of 2.36 Pg CO2e.53
4. Conclusions
Powerships represent a growing source of GHGs and air pollutants in regions keen to quickly address energy shortages. The ability to reliably assess the impacts of adoption of powerships on air quality, climate, and Paris Climate Accord commitments is challenging due to a lack of measurements of air pollutant and GHG emission factors and a detailed understanding of operating conditions characteristic of powerships.
Emission control technologies and international regulation have the potential to mitigate air pollutant emissions from powerships and LNG tanker transport, but many countries adopting powerships lack environmental regulation or the means to enforce regulation. Measurements of CH4 losses during regasification and liquefaction and air pollutant and GHG emissions from gas-fuelled reciprocating engines under a range of representative operating conditions should be prioritised to reduce uncertainties in emission estimates.
Author contributions
OA conducted formal analysis and data curation and contributed to methodology development. Supervision, writing (original draft, review and editing), conceptualization, funding acquisition, investigation, and methodology were by EAM. CW aided in methodology and writing (review and editing).
Conflicts of interest
There are no conflicts to declare.
Acknowledgements
EAM is grateful for funding from NERC/EPSRC (grant number EP/R513465/3). OA was supported by the NERC summer Research Experience Programme (REP).
References
-
T. Carnie, Karpowership wins last-minute Nersa approval – but environment minister Barbara Creecy is yet to weigh in, Daily Maverick, 21 September 2021, https://www.dailymaverick.co.za/article/2021-09-21-karpowership-wins-last-minute-nersa-approval-but-environment-minister-barbara-creecy-is-yet-to-weigh-in/ Search PubMed.
- E. A. Marais, R. F. Silvern, A. Vodonos, E. Dupin, A. S. Bockarie, L. J. Mickley and J. Schwartz, Air quality and health impact of future fossil fuel use for electricity generation and transport in Africa, Environ. Sci. Technol., 2019, 53, 13524–13534 CrossRef PubMed.
-
Karpowership, Karpowership SA appointed as preferred bidder on the RMIPPPP, http://www.karpowership.com/es/karpowership-sa-appointed-as-preferred-bidder-on-the-rmipppp Search PubMed.
- A. Q. Gilbert and B. K. Sovacool, US liquefied natural gas (LNG) exports: Boom or bust for the global climate?, Energy, 2017, 141, 1671–1680 CrossRef.
- A. R. Brandt, G. A. Heath, E. A. Kort, F. O'Sullivan, G. Petron, S. M. Jordaan, P. Tans, J. Wilcox, A. M. Gopstein, D. Arent, S. Wofsy, N. J. Brown, R. Bradley, G. D. Stucky, D. Eardley and R. Harriss, Methane leaks from North American natural gas systems, Science, 2014, 343, 733–735 CrossRef CAS PubMed.
- R. A. Alvarez, S. W. Pacala, J. J. Winebrake, W. L. Chameides and S. P. Hamburg, Greater focus needed on methane leakage from natural gas infrastructure, Proc. Natl. Acad. Sci. U. S. A., 2012, 109, 6435–6440 CrossRef CAS PubMed.
- K. Vohra, A. Vodonos, J. Schwartz, E. A. Marais, M. P. Sulprizio and L. J. Mickley, Global mortality from outdoor fine particle pollution generated by fossil fuel combustion: Results from GEOS-Chem, Environ. Res., 2021, 195, 110754 CrossRef CAS PubMed.
-
API (American Petroleum Institute), Liquefied Natural Gas (LNG) Operations: Consistent Methodology for Estimating Greenhouse Gas Emissions, 2015 Search PubMed.
-
IPCC, 2006 IPCC Guidelines for National Greenhouse Gas Inventories, UN, 2006 Search PubMed.
-
P. Forster, V. Ramaswamy, P. Artaxo, T. Berntsen, R. Betts, D. W. Fahey, J. Haywood, J. Lean, D. C. Lowe, G. Myhre, J. Nganga, R. Prinn, G. Raga, M. Schulz and R. V. Dorland, in Climate Change 2007: the Physical Science Basis. Contribution of Working Group I to the Fourth Assessment Report of the Intergovernmental Panel on Climate Change, eds. S. Solomon, D. Qin, M. Manning, Z. Chen, M. Marquis, K. B. Averyt, M. Tignor and H. L. Miller, Cambridge University Press, Cambridge, UK, 2007 Search PubMed.
-
DFFE, Department of Environment, Forestry and Fisheries National Environmental Management: Air Quality Act, 2004, 2010 Search PubMed.
-
NETL, National Energy Technology Laboratory: Life Cycle Analysis: Natural Gas Combined Cycle (NGCC) Power Plants, US DOE, 2018 Search PubMed.
-
EEA, European Environment Agency: Guidebook 2019: 1.A.1 Energy Industries, 2019 Search PubMed.
-
DEA, GHG National Inventory Report South Africa 2000-2010, 2014 Search PubMed.
- C. Geel, H.-M. Schulz, P. Booth, M. deWit and B. Horsfield, Shale Gas Characteristics of Permian Black Shales in South Africa: Results from Recent Drilling in the Ecca Group (Eastern Cape), Energy Procedia, 2013, 40, 256–265 CrossRef CAS.
- P. R. O'Donoughue, G. A. Heath, S. L. Dolan and M. Vorum, Life Cycle Greenhouse Gas Emissions of Electricity Generated from Conventionally Produced Natural Gas, J. Ind. Ecol., 2014, 18, 125–144 CrossRef.
- A. Burnham, J. Han, C. E. Clark, M. Wang, J. B. Dunn and I. Palou-Rivera, Life-Cycle Greenhouse Gas Emissions of Shale Gas, Natural Gas, Coal, and Petroleum, Environ. Sci. Technol., 2011, 46, 619–627 CrossRef PubMed.
- I. Tamura, T. Tanaka, T. Kagajo, S. Kuwabara, T. Yoshioka, T. Nagata, K. Kurahashi and H. Ishitani, Life cycle CO2 analysis of LNG and city gas, Appl. Energy, 2001, 68, 301–319 CrossRef CAS.
- L. S. Abrahams, C. Samaras, W. M. Griffin and H. S. Matthews, Life Cycle Greenhouse Gas Emissions From U.S. Liquefied Natural Gas Exports: Implications for End Uses, Environ. Sci. Technol., 2015, 49, 3237–3245 CrossRef CAS PubMed.
-
ICCT, International Council on Clean Transportation Assessment of the Fuel Cycle Impact of Liquefied Natural Gas as Used in International Shipping, 2013 Search PubMed.
- D. Farquharson, P. Jaramillo, G. Schivley, K. Klima, D. Carlson and C. Samaras, Beyond global warming potential: A comparative application of climate impact metrics for the life cycle assessment of coal and natural gas based electricity, J. Ind. Ecol., 2017, 21, 857–873 CrossRef CAS.
-
DMR, Department of Mineral Resources and Energy: The Risk Mitigation Independent Power Producer Procurement Programme (RMIPPPP) in Context, 2021 Search PubMed.
-
Shell, The Shell Shipping Fleet, https://www.shell.com/business-customers/trading-and-supply/shell-shipping-and-maritime/our-shipping-fleet.html, accessed 10 August 2021 Search PubMed.
-
Shell, LNG Supply Projects and Regasification Plants, https://www.shell.com/energy-and-innovation/natural-gas/liquefied-natural-gas-lng/lng-supply-projects-and-regasification-plants.html, accessed 17 August 2021 Search PubMed.
- R. P. Sinha and W. M. N. Wan Nik, Investigation of propulsion system for large LNG ships, IOP Conf. Ser.: Mater. Sci. Eng., 2012, 36, 012004 Search PubMed.
- P. Jaramillo, W. M. Griffin and H. S. Matthews, Comparative Life-Cycle Air Emissions of Coal, Domestic Natural Gas, LNG, and SNG for Electricity Generation, Environ. Sci. Technol., 2007, 41, 6290–6296 CrossRef CAS PubMed.
- J. Pospíšil, P. Charvát, O. Arsenyeva, L. Klimeš, M. Špiláček and J. J. Klemeš, Energy demand of liquefaction and regasification of natural gas and the potential of LNG for operative thermal energy storage, Renewable Sustainable Energy Rev., 2019, 99, 1–15 CrossRef.
- M. Ulvestad and I. Overland, Natural gas and CO2 price variation: impact on the relative cost-efficiency of LNG and pipelines, Int. J. Environ. Sci., 2012, 69, 407–426 CAS.
-
Shell, LNG Supply Projects and Regasification Plants, https://www.shell.com/energy-and-innovation/natural-gas/liquefied-natural-gas-lng/lng-supply-projects-and-regasification-plants.html, accessed 14 November 2021 Search PubMed.
-
EPA (US Environmental Protection Agency), Natural gas combustion, https://www.epa.gov/sites/default/files/2020-09/documents/1.4_natural_gas_combustion.pdf, accessed 10 August 2021 Search PubMed.
-
EPA (US Environmental Protection Agency), Natural gas-fired reciprocating engines, https://www3.epa.gov/ttnchie1/ap42/ch03/final/c03s02.pdf, accessed 1 March 2022 Search PubMed.
-
IMO, IMO 2020 – cutting sulphur oxide emissions, https://www.imo.org/en/MediaCentre/HotTopics/Pages/Sulphur-2020.aspx, accessed 1 March 2022 Search PubMed.
-
EIA, US Energy Information Administration: How much coal, natural gas, or petroleum is used to generate a kilowatthour of electricity?, https://www.eia.gov/tools/faqs/faq.php?id=667%26t=8, accessed 10 August 2021 Search PubMed.
- M. S. Diamond, H. M. Director, R. Eastman, A. Possner and R. Wood, Substantial Cloud Brightening From Shipping in Subtropical Low Clouds, AGU Adv., 2020, 1, e2019AV000111 Search PubMed.
- S. B. Dalsøren, M. S. Eide, G. Myhre, Ø. Endresen, I. S. A. Isaksen and J. S. Fuglestvedt, Impacts of the large increase in international ship traffic 2000−2007 on tropospheric ozone and methane, Environ. Sci. Technol., 2010, 44, 2482–2489 CrossRef PubMed.
- A. Lauer, V. Eyring, J. J. Corbett, C. Wang and J. J. Winebrake, Assessment of near-future policy instruments for oceangoing shipping: Impact on atmospheric aerosol burdens and the Earth's radiation budget, Environ. Sci. Technol., 2009, 43, 5592–5598 CrossRef CAS PubMed.
- A. Lauer, V. Eyring, J. Hendricks, P. Jöckel and U. Lohmann, Global model simulations of the impact of ocean-going ships on aerosols, clouds, and the radiation budget, Atmos. Chem. Phys., 2007, 7, 5061–5079 CrossRef CAS.
- M. Righi, J. Hendricks and R. Sausen, The global impact of the transport sectors on atmospheric aerosol: Simulations for year 2000 emissions, Atmos. Chem. Phys., 2013, 13, 9939–9970 CrossRef.
- Q. Li, A. Badia, R. P. Fernandez, A. S. Mahajan, A. I. López-Noreña, Y. Zhang, S. Wang, E. Puliafito, C. A. Cuevas and A. Saiz-Lopez, Chemical Interactions Between Ship-Originated Air Pollutants and Ocean-Emitted Halogens, J. Geophys. Res.: Atmos., 2021, 126, e2020JD034175 CAS.
- M. Sofiev, J. J. Winebrake, L. Johansson, E. W. Carr, M. Prank, J. Soares, J. Vira, R. Kouznetsov, J.-P. Jalkanen and J. J. Corbett, Cleaner fuels for ships provide public health benefits with climate tradeoffs, Nat. Commun., 2018, 9, 406 CrossRef PubMed.
- M. Viana, P. Hammingh, A. Colette, X. Querol, B. Degraeuwe, I. d. Vlieger and J. van Aardenne, Impact of maritime transport emissions on coastal air quality in Europe, Atmos. Environ., 2014, 90, 96–105 CrossRef CAS.
- J. E. Jonson, M. Gauss, M. Schulz, J.-P. Jalkanen and H. Fagerli, Effects of global ship emissions on European air pollution levels, Atmos. Chem. Phys., 2020, 20, 11399–11422 CrossRef CAS.
-
IMO, International Convention for the Prevention of Pollution from Ships (MARPOL), https://www.imo.org/en/About/Conventions/Pages/International-Convention-for-the-Prevention-of-Pollution-from-Ships-(MARPOL).aspx, accessed 28 September 2021 Search PubMed.
-
Shell, Shell Sustainability Report, 2021 Search PubMed.
- I. Pretorius, S. Piketh, R. Burger and H. Neomagus, A perspective on South African coal fired power station emissions, J. Energy South Afr., 2015, 26, 27–40 CrossRef.
- P. J. D. Lloyd and A. Cook, Methane release from South African coalmines, J. S. Afr. Inst. Min. Metall, 2005, 105, 483–490 CAS.
- J. M. Dabrowski, P. J. Ashton, K. Murray, J. J. Leaner and R. P. Mason, Anthropogenic mercury emissions in South Africa: Coal combustion in power plants, Atmos. Environ., 2008, 42, 6620–6626 CrossRef CAS.
- A. Dalton, G. T. Feig and K. Barber, Trace metal enrichment observed in soils around a coal fired power plant in South Africa, Clean Air J., 2018, 28 DOI:10.17159/2410-972x/2018/v28n2a1.
- F. Tshidavhu and N. Khatleli, An assessment of the causes of schedule and cost overruns in South African megaprojects: A case of the critical energy sector projects of Medupi and Kusile, Acta Structilia, 2020, 27, 119–143 CrossRef.
-
EIA, Country Brief: South Africa, https://www.eia.gov/international/analysis/country/ZAF, accessed 27 September 2021 Search PubMed.
-
EC, European Commission: EDGAR - Emissions Database for Global Atmospheric Research: Country Fact Sheet, https://edgar.jrc.ec.europa.eu/country_profile/ZAF, accessed 10 August 2021 Search PubMed.
-
IEA, International Energy Agency: Africa Energy Outlook 2019, 2019 Search PubMed.
-
ClimateWatch, Historical GHG Emissions, 2021 Search PubMed.
Footnote |
† Electronic supplementary information (ESI) available. See DOI: 10.1039/d1va00049g |
|
This journal is © The Royal Society of Chemistry 2022 |