Techno-socio-economic analysis of geological carbon sequestration opportunities†
Received
23rd November 2021
, Accepted 10th March 2022
First published on 11th March 2022
Abstract
Although geological carbon sequestration is considered one of the pillars required to achieve the goals of the Paris Agreement, only a few demonstration sites are currently being developed around the globe. Lab-scale tests, pilot-scale tests, and a few pioneering demonstration projects suggest that substantial amounts of CO2 could be stored in depleted hydrocarbon reserves, saline aquifers, basalts and un-minable coal reserves, albeit a number of risks need to be managed. In this paper, we identify key features of potential geological sequestration sites and study their feasibility via a social-economic assessment, including technical parameters such as volumetric capacity, and reservoir characteristics such as porosity, depth, formation thickness, and initial water saturation. Several geographical sites were further studied in terms of the lifetime duration of a possible geological repository for a preliminary economic assessment. Among the five sites considered, i.e., Cantarell in Mexico, Oloibiri in Nigeria, Frigg in Norway, Rio Vista in the United States of America and Romashkino in Russia, our analysis identifies the Frigg Field as the most favourable site for geological carbon sequestration because of its significant volumetric capacity, no obvious cautionary technical issues, optimistic economic outlook, and extensive social support. Although preliminary, our results suggest that a viable industrial operation could be maintained for several decades in this location, paving the way for the global implementation of geological carbon sequestration required to achieve the goals of the Paris Agreement.
Environmental significance
Large scale adoption of carbon sequestration is required to mitigate excessive carbon emissions to the atmosphere, which are primarily generated by the industrial and power sectors. Here, we consider potential sites around the globe which possess the capacity to store substantial quantities of carbon dioxide. Out of the sites considered, analysis of technical, economic and societal requirements has identified the most feasible sites globally. The approach implemented could help identify future sequestration sites to ensure the necessary measures are taken to avoid excessive accumulation of CO2 in the atmosphere.
|
1. Introduction
A growing global population with increasing standards of living has led to an expanding industrial sector and an alarming increase in fossil fuel dependence. However, the use of fossil fuels has led to the emission of large quantities of carbon dioxide (CO2), a greenhouse gas (GHG) whose increased concentration in the atmosphere is associated with climate change, correlated with increased warming of the Earth's surface. The Paris Agreement aims to limit this increase in global temperature to “well below 2 °C”,1 which requires, within many different scenarios, significant reductions in CO2 emissions, and perhaps also in capturing part of the CO2 already present in the atmosphere. In fact, since the industrial revolution, atmospheric CO2 concentration has increased by 47%.2
Carbon Capture and Sequestration (CCS) is a process in which CO2 is captured, preferably from high concentration CO2 emitters such as power or industrial sources, but also from the atmosphere when feasible, and is then transported to an appropriate site for long-term storage.3 There are different types of sequestration methods such as terrestrial sequestration, ocean sequestration and geological sequestration.4 The focus of this paper is geological carbon storage, whereby CO2 is compressed and injected into underground formations. Suitable geological formations include depleted oil and gas reservoirs, coal beds, aquifers and basalt formations.5 Although it is widely accepted that geological carbon sequestration is required to achieve the goals of the Paris Agreement, few industrial-scale operations are currently underway, presumably because of several risks and concerns, ranging from induced seismicity, to future liability in case of escape, in the long term, of the sequestered CO2. It is noted that prior pilot-scale CCS projects are vital in providing key learnings, including from technical, economic, and social aspects. We survey below the key learnings achieved from selected pilot-scale CCS operations conducted around the globe, which provide the foundations for techno-socio-economic evaluations for future geological carbon sequestration projects. Out of 14 possible geological sites considered, we short-listed three nearly depleted hydrocarbon sites. We discuss below their commercial deployment and identify economic, technical and social factors that could discriminate a feasible site for industrial-scale CO2 sequestration.
1.1. Point sources of CO2
According to current literature, the largest CO2 emissions arise from transport, industrial and electricity generation sectors. The primary emission sectors are similar, globally. Prime point sources, therefore, include power facilities and CO2-emitting industries such as cement, iron and steel manufacture.6 Methodologies for storing CO2 from these locations will be considered in what follows.
1.2. Methods for geological carbon sequestration
Geological carbon storage involves the separation of CO2 from industrial emissions, compression, transportation to injection sites, and injection into deep subsurface formations to achieve long-term storage. Promising formation candidates include coal seams, deep saline aquifers, basaltic rocks and depleted or mature oil and gas fields.7,8 Of concern is the possibility that the stored CO2 would eventually seep back to the atmosphere over the years. Potential sites with presumably suitable caprocks are identified in an effort to minimise this possibility.
1.2.1. Depleted hydrocarbon reservoirs.
Carbon storage in depleted hydrocarbon reservoirs requires CO2 to fill, at least partially, the pore space in sedimentary rock formations and to remain within these cavities indefinitely; impermeable rocks, known as caprocks, are expected to impede the CO2 leakage to the surface or to other locations. This approach is often considered as the most viable geological sequestration option,9 mainly for three reasons: (1) depleted hydrocarbon sites have been studied for long periods, leading to large datasets and information that can assist reducing operational risks;8 (2) costs can be reduced since injection wells and other facilities are already in place;9 (3) enhanced oil recovery (EOR), a technique often adopted to recover residual oil-in-place, can be promoted by CO2 injection.8 It should be recognised that drilling and injecting in depleted hydrocarbon reservoirs involve a pressure build up, which could result in formation damage and potential fluid leakages through the cracks formed. This risk can likely be managed, as demonstrated by the SACROC project, which has been running in a depleted hydrocarbon reservoir for over 50 years.10
1.2.2. Saline aquifers.
Saline aquifers are sedimentary rocks containing saline fluids. Formations with sufficient porosity and permeability can be chosen for sequestration projects when they are located at depths of at least 800 m below sea level. When CO2 is injected into saline aquifers, often at supercritical conditions, it can be more buoyant than the reservoir fluids; therefore, CO2 would tend to rise to the top, while some of the injected CO2 could dissolve within the brine. In some cases, the dissolution of CO2 in saline water alters porosity, permeability and transport pathways for CO2 within the reservoir,11 therefore, a layer of impermeable caprock is often required to trap CO2.
An advantage of saline aquifers compared to other formations is their high porosity, which yields high capacity for storage, which has been estimated in around 1000–10
000 giga tonnes of CO2.11 It has been estimated that saline aquifer formations can retain CO2 for 1000–10
000 years.11 A successful example of such carbon storage implementations is the Sleipner project, in which more than 17 Mtonne of CO2 has been stored.12 However, since no pre-existing infrastructure is generally available at these sites, it is expected that large capital investments are required to build the infrastructure and to minimise leakage risks.12
1.2.3. Un-mineable coal beds.
Un-mineable coal beds represent potential targets for geological carbon sequestration because coal has a high adsorption capacity for CO2. Injected CO2 is adsorbed into the rock matrix of the coal seam and trapped physically by cleats within the coal due to the intrinsic low permeability of the formation. It has been estimated that CO2 could remain sequestered within the coal bed for up to 105–106 years, as long as the formation remains unmined. Injected CO2 may also displace methane, which could lead to enhance natural gas recovery.13 In general, the competitive adsorption of CO2 and methane in coal needs to be better understood to further this attractive technology.13 An example project of un-mineable coal beds is FLEXIS, in Poland, which focuses on carbon sequestration without combined enhanced methane recovery operations.
1.2.4. Carbon mineralisation in basaltic formations.
Basalts are dark rocks formed by the rapid cooling of lava from volcanic eruptions. They contain high quantities of magnesium, iron and calcium, are weakly basic, and can react with CO2 in a mineralization reaction, forming a nonbuoyant solid carbonate.14 Extensive amounts of water are usually needed for the mineralization, which at the moment renders such sequestrations nearly twice as expensive compared to other geological sequestration methods.15 However, global estimates suggest that mineralisation in basaltic formations, worldwide, could permanently sequester up to 100
000 Gtonnes of CO2. Further, because of mineralisation, caprocks are not required to prevent CO2 leakage. Although recent studies suggest that CO2 can form minerals within one year of injection,16 the method is still being optimised, for example to reduce water usage and control the kinetics of the reaction. Recent success has been reported by the related CarbFix and CarbFix2 projects in Iceland.15
1.3. Examples of carbon sequestration projects
To understand the feasibility of future implementation of carbon sequestration, it is necessary to learn best practices from current demonstration projects being pioneered around the world. Here we summarise different CCS project, referring to the technologies briefly summarised in Section 1.2.
1.3.1. Depleted hydrocarbon reservoirs – SACROC, Texas.
CO2 injection in the Scurry Area Canyon Reef Operators Committee (SACROC) oil field in Texas, USA, began in 1972. This project is recorded as one of those which injected the largest amounts of CO2 so far, globally.17 In this project, CO2 injection facilitates enhanced oil recovery (EOR). In this CO2-EOR project, about 100 million tonnes of CO2 have been stored. The long-term duration of the site acts as a good reference in examining and assessing future projects in depleted hydrocarbon formations.
The SACROC site has 1700 wells and 240 active injectors.18 Monitoring of surrounding groundwater supplies suggests that the shale caprock formation has successfully acted as a seal. Examination has been carried out on a sample of Portland cement wells (well 49-6) at the SACROC site. The results show that the structural integrity of the wells tested has been retained after 30 years of CO2 exposure, which has successfully prevented significant transport of fluids through cement.19 Shallow groundwater has been slightly contaminated with a small quantity of oil-field brine, which could potentially be due to leakage of CO2-saturated brine through shale matrices or pits at the surface.20 The observations from this project indicate that the sequestration of CO2 over a relatively long time can be achieved via storage in mature oil reservoirs, as long as an adequate seal is in place.
1.3.2. Saline aquifers – Sleipner, Norway.
Sleipner, operated by Equinor, is an offshore carbon storage project that began operations in 1996 in Norway.21 The Sleipner project has provided a useful understanding of the technical and economic requirements of monitoring CO2 storage in saline aquifers.22 Nearly one million tonnes of CO2 per year has been stored in the aquifer, ∼1 km below sea level.21 The overlying shale layers act as a caprock seal with a pressure threshold high enough to contain the CO2 stored underneath.23 In this project, operators found uncertainty concerning the integrity of the seals, which demanded high monitoring costs.21Via 3-D seismic surveys, the operators monitored rigorously the operation and found that 5% of the pore volume has been occupied since 2008;23 the reports suggest the enduring integrity of the caprock in place. In part because Sleipner was a pioneering project initiated when few operational guidelines were in place, in part because of the extensive seismic monitoring approaches implemented in this project, and in part for other reasons, the cumulative operational costs of the Sleipner project have exceeded $100 million.
1.3.3. Un-mineable coal beds – Flexis, Poland.
The project FLEXIS commenced in September 2020, with a strategy to inject CO2 into coal seams in a test site in Mikolow, Poland.24 Current plans are outlined for pilot testing followed by field testing over an approximately 5 year period. At the pilot site, CO2 injection and comprehensive monitoring of safety risks will occur in conjunction with lab testing and modelling work meant to monitor changes in formation permeability under sequestration conditions;17 assuming the pilot will succeed, a larger-scale commercial site will be chosen for quantifying the field-scale efficiency of CO2 injectivity as well as for conducting a techno-economic analysis. Best practice procedures could be learned from Australia, where carbon sequestration in deep un-mineable coal seams has been attempted.25,26 At this stage, it is understood that CO2 sequestration in un-mineable coal beds requires extensive studies, at lab- to field-scales, before it is accepted as a feasible method for commercial carbon storage.
1.3.4. Basalts – CarbFix, Iceland.
The ongoing CarbFix project in Iceland is storing CO2 in the basalt formation used for geothermal energy production in the region. Once hot water generates power in the turbines, the ‘spent’ CO2–water mixture is injected into the target basalt formation. The mixed fluid reacts with basalts and forms a solid carbonate, which immobilises the CO2 within the formation. The process is considered successful, with an annual storage capacity of 12
000 tonnes; it has been estimated that 80% of the injected CO2 has been successfully mineralized within one year of operations.15 During part of the CarbFix project, 10–20 ktonnes per year of CO2 has been injected, exhibiting the rapid carbon mineralization rates.27 Current estimated costs for the CarbFix method range between $20–$30 per tonne of CO2; it is expected that CO2 mineralisation in basalt will most likely be successful in regions with volcanic activity.27 Although more experience through pilot studies is required to mitigate the risks associated with this carbon sequestration strategy,28 recent developments suggest a possibility of using seawater for achieving CO2 mineralisation.29
In summary (see Table 1), the above analysis suggests that, at present, the most attractive geological storage method for CO2 sequestration is using depleted hydrocarbon fields. Advantages include low capital costs, significant availability of essential reservoir data, extensive knowledge of the geochemical processes involved in the operation, and overall manageable safety risks. This said, the other technologies also present advantages, and it is expected they could soon be viable at scale.
Table 1 Summary of learnings from pilot carbon sequestration studies
Technology |
Case study |
Benefits |
Drawbacks |
Depleted hydrocarbon reservoir |
SACROC, Texas |
• Vast amount of data available for the formation |
• Existing wells could lead to preferential pathways for CO2 leaks |
• Decreased costs with facilities already in place |
• Potential large distances from point sources of CO2 to remote storage sites |
• Further economic viability due to potential for enhanced oil recovery |
|
Saline aquifers |
Sleipner, Norway |
• Large capacities for storage |
• No pre-existing infrastructure available incurring greater costs |
• Significant depths |
• The integrity of saline formations acting as an effective seal is uncertain |
• Physical properties could help prevent CO2 leaks |
|
Basalts |
Carbfix, Iceland |
• Successful mineralization of CO2 within one year |
• Method is still in research stages |
• Potential prevention of future CO2 leaks |
• Potential large amount of fresh water usage (Basalts) |
Un-minable coal beds |
FLEXIS, Poland |
• Potential for methane production connected with CO2 sequestration |
|
1.4. Criteria for selecting a potential CO2 geological sequestration site
As outlined above, the requirements for the success of a sequestration site are extensive. A comprehensive feasibility study is essential when choosing a carbon sequestration site, given the potential leakage risks and the high operational and monitoring costs. Previous results have indicated significant factors that need to be considered to estimate risks are: technical parameters, economic viability, and social concerns.30,34 We discuss these aspects in details below.
1.4.1. Technical parameters.
Formation geology.
Appropriate geology of the rock formation is essential for the successful storage of CO2. The best-suited rocks for storage are sedimentary rocks. Such rocks are favoured because they possess a porosity sufficiently high for storing a significant amount of CO2 and have a high surface horizontal permeability that aids well injectivity.31
Fluid state of CO2 in the formation.
A factor affecting the storage capacity is the fluid state of CO2, which depends on the depth, temperature and pressure conditions of the reservoir.31 It is optimal for CO2 to be in a supercritical state so that the CO2 occupies the smallest pore volume possible, therefore achieving the maximum storage capacity, while its viscosity allows for good processability. The supercritical state implies that the density of CO2 is similar to that of the liquid state, and that its viscosity is similar to that of the gas phase, a condition which can be achieved at temperatures above 32 °C and pressures above 73.7 bar; the minimum depth required for these conditions is ∼800 m.10 In general, these conditions are likely found in deep geological reservoirs.32
Trapping mechanism.
Assessment of seals and well integrity is essential to CO2 trapping.33 The trapping mechanism depends on the reservoir characteristics, such as confining CO2 under a low-permeability cap rock, mineral trapping which dissolves the CO2 to form carbonate precipitates, and/or leveraging surface tension to confine CO2 in pores as an immobile phase.20
To choose a suitable CO2 storage site, a screening process taking into consideration all the aforementioned parameters is required. Such preliminary screening can be conducted using as reference the parameters summarised in Table 2.
Table 2 Parameters required for consideration in choosing a carbon sequestration site34
Parameters |
Positive indicators |
Cautionary indicators |
Depth |
1000–2500 m |
<800 m or >2500 m |
Permeability |
>300 mD |
10–100 mD |
Interval thickness |
≫50 m |
<20 m |
Porosity |
>20% |
<10% |
Density |
300–1000 kg m−3 |
<300 kg m−3 |
Residual water saturation |
Less |
High |
Distance between CO2 source and target formation |
<300 km |
>300 km |
1.4.2. Economic viability.
Economic viability is an essential aspect when evaluating a potential geological site. Since CO2 pipeline transportation costs are highly variable and dependant on the nature of the terrain the infrastructure passes through, the lowest-cost point source close to the storage site is essential to secure longevity of the operation.35 Costs encountered prior to the storage stage include feasibility studies, capture and transportation. Cost during storage includes capital costs which comprise of infrastructure for injection, drilling of wells, initial surveys and other field requirements. Operational costs include running monitoring networks, maintenance and labour costs.11
For economic feasibility to be achieved, our assumption is that the cost of sequestration must be lower than the imposed carbon tax. Indeed, governments introduce carbon taxes primarily to reduce carbon emissions. If the storage project costs less than the enforced taxes, point source companies will be encouraged to implement the sequestration methods. For example, the Sleipner field, mentioned above, commenced carbon sequestration methods in 1996 probably for a number of reasons, including the Norwegian government's carbon taxes. The storage site receives credits, yielding an attractive return on investment.36 It should be pointed out that carbon tax regimes are different in different parts of the world, which could affect geopolitical decisions in this field. For example, the 45-Q tax credit has applied to the sites in the US.136 However, we did not find information about whether the Rio Vista site, the only US site in this study, has benefitted from 45-Q tax credits. The other sites considered in this study are outside of the US.
1.4.3. Social acceptability assessment.
The success of a carbon sequestration project is heavily reliant on the support of a range of stakeholder groups, including government, local population, non-governmental organizations (NGOs), policymakers, investors, contractors and social media. For example, (1) securing governmental support is essential, and political leaders frequently, but not always, endorse CO2 storage projects. (2) Local's views have had significant impacts on past CCS projects; public concern over CO2 leakage disrupted the Schwartz Pump project close to populous towns in Germany, possibly in part because of the involvement of NGO groups.37,107 (3) Environmental groups could promote unfavourable views, stating the CCS process is insufficient to tackle climate change within the required time, which could impede or delay the implementation of carbon storage projects.38 (4) Local policymakers can also play a key role in determining the outcome of a project. For example, the Dutch Barendrecht project, intended to store CO2 from a Shell refinery, failed due to resistance from local authorities causing delays in securing the permissions required for execution of the plan.39 In sum, it is becoming increasingly apparent that stakeholders' perceptions translate to support or resistance to the project, and this often determines the project's fate. We provide an exemplar Stakeholder groups analysis in Table 3.
Table 3 Stakeholder analysis for a potential geological carbon sequestration project
Stakeholder Category |
Reasons to be interested in the project |
Influence on the project |
Local population |
• Job opportunities |
• Willing local workforce |
• Safety of the project |
• Vocal influence on policymakers |
• Unaffected living conditions |
|
Policy Makers (nationwide) |
• Remediating negative impact on environment |
• Potential for government financial support/investment |
• Improving the countries global position in GHG emissions |
• Issuing regulations impacting the feasibility of the project |
• Sharing responsibilities to remediate negative environmental impacts |
• Prioritisation of different projects |
• Socio-economic development |
|
Policy Makers (municipality wide) |
• Relating safety to surrounding communities and environment |
• Opportunities to lobby |
• Increasing attractiveness of investment in the region |
• Influencing perception of local public and higher-lever policy makers |
• Socio-economic development |
|
Financial Investors |
• Economic success of the project, a financial net positive result |
• Providing financial resources for the project |
• Expanding portfolios and increase an environmentally conscious reputation |
|
Non-Governmental Organizations |
• Safety of the project to the environment and surrounding communities |
• Potential to lobby against the project |
|
• Potential to create a negative perception of the project to other stakeholders |
Suppliers and Contractors |
• Creating long-term contracts for sustainable upkeep of the project |
• Technical feasibility of the project to meet requirements |
• Financial benefits |
|
Media |
• Providing information of the project |
• Influencing general perception of the project |
• Gaining public attention |
• Influencing the reputation of the operating company |
We generate a stakeholder matrix in Fig. 1 to identify the best communication strategy for each of the stakeholders groups discussed in Table 3. Our analysis suggests that: (1) significant time and efforts must be devoted to liaising with governments, local authorities, and investors to support geological carbon storage projects. (2) Regulations and financial assets will ultimately deem the feasibility of the project. (3) It is likely essential to keep close communication with suppliers and contractors, to ensure excellent technical support, as they will influence the long-term success of the project. (4) Given the examples we examined above, it is essential that local communities and NGOs are supportive and are kept satisfied with the planning and development of the project. (5) Transparency and complete involvement, from the planning stages of a project onwards, are required to maintain a social license to operate. (6) Last but not least, media perception is important, although this might have lesser significance as it is highly dependent on other stakeholders, who should be actively involved.
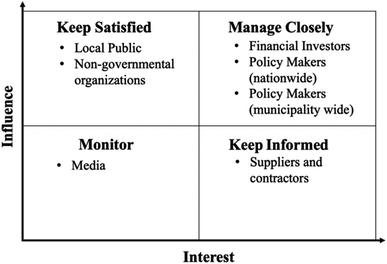 |
| Fig. 1 Stakeholder matrix, indicating a possible engagement strategy for some of the stakeholder groups with different levels of interest on a carbon sequestration project. | |
2. Methodology
Based on the above overview, long-term CO2 storage in depleted hydrocarbon fields has been considered as the most viable and economic option for the time being. It has been estimated that 80% of the world's oil reserves are suitable for CO2 recovery.40 We initially considered 14 sites throughout the world, as listed in Table 4. It should be noted that, because the peak production in these sites occurred as far back as in the middle of the twentieth century, we did not include the potential benefit due to hydrocarbon production via EOR in our analysis – we just focused on costs and benefits due to CO2 sequestration. Out of these 14 geological sites, a few were short-listed (see Table 5) via this workflow: (1) the list of possible sites is narrowed down to those sites which have peaked in production at least 15 years ago and are considered at least 85% depleted of their Estimated Ultimate Recovery (EUR). (2) Because a concentrated point source is considered necessary for the long-term success and viability of the project, sites found in countries holding only CO2 point sources of <30 million tonnes of CO2 per year were discarded. (3) Out of the remaining sites, only those found within countries which were assumed to have sufficient economic capacity to accomplish a geological carbon sequestration project were selected, and those found within countries with ongoing political unrest were also omitted. This procedure led us to short-list potential fields in Mexico, Nigeria, Norway, the USA and Russia. When several possible geological sites are available within a country, a single depleted field was selected in that country by considering the shortest distance (100–300 km) from large carbon emitters, supporting reduced costs and risks related to CO2 transportation. We note that the initial list of potential sites (Table 4) contains two from USA; the Rio Vista site outperforms the Atlantis one based on our selection criteria because its peak production year was in 1940 as opposed to 2007, and because its depletion ration is estimated in 87%. Therefore, the Rio Vista site was chosen for further investigation. This selection does not imply that the Rio Vista site is proposed here as representative of sequestration in the entirety of the US. The geographical location of the shortlisted sites is shown in Fig. 2, in relation to the global CO2 storage potential.
Table 4 List of nearly depleted sites considered for the exercise discussed herein
Country |
Site |
Year of peak |
Depletion ration |
Saudi Arabia |
Ghanwar Field |
2005 |
8% (ref. 41) |
Kuwait |
Burgan Field |
2005 |
14% (ref. 42) |
Mexico |
Cantarell Field |
2004 |
92% (ref. 43) |
Russia |
Samorlor Field |
1980 |
73% (ref. 44) |
Russia |
Romashkino |
1949 |
85% (ref. 45) |
Nigeria |
Oloibiri |
1964 |
85% (ref. 46) |
USA |
Rio Vista |
1940 |
87% (ref. 47) |
Norway |
Frigg |
1990 |
89% (ref. 48) |
Pakistan |
Qadirpur |
2017 |
43% (ref. 49) |
Libya |
East Central Mabruk |
1999 |
8% (ref. 50) |
Netherlands |
Groningen |
1980 |
6% (ref. 51) |
Libya |
Zella |
1998 |
60% (ref. 52) |
China |
Daqing |
2008 |
4.50% (ref. 53) |
USA |
Atlantis |
2007 |
57% (ref. 54) |
Table 5 List of chosen potential carbon sequestration sites
Site |
Field index |
Company |
Year discovered |
Year of peak hydrocarbon production |
Country CO2 emissions (Mtonne per year) |
Cantarell, Mexico |
I |
Peroleos Mexicanos |
1976 (ref. 55) |
2004 (ref. 55) |
665.3 (ref. 56) |
Oloibiri, Nigeria |
II |
Shell |
1956 (ref. 57) |
1964 (ref. 57) |
82.1 (ref. 57) |
Frigg, Norway |
III |
Total SE |
1977 (ref. 58) |
2004 (ref. 58) |
33.6 (ref. 59) |
Rio Vista, USA |
IV |
Rosetta Resources |
1936 (ref. 60) |
1951 (ref. 60) |
670 (ref. 61) |
Romashkino, Russia |
V |
Tatneft |
1948 (ref. 62) |
1993 (ref. 62) |
150 (ref. 62) |
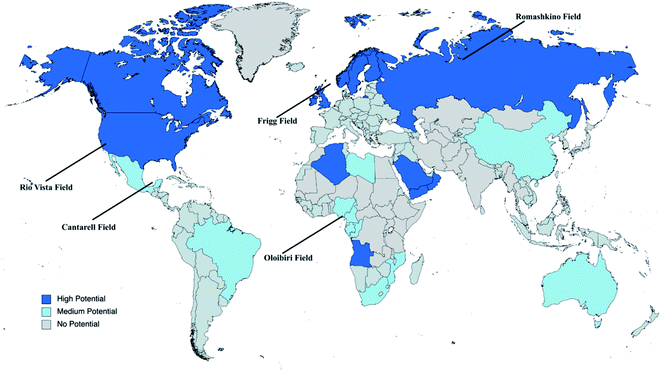 |
| Fig. 2 Geographical location of the five sites shortlisted in Table 5 in relation to the global CO2 storage potential.63–66 | |
From the five sites identified in Table 5, we conducted a techno-socio-economic feasibility study to narrow down our choice to the single potentially most feasible site for carbon sequestration. The following workflow was implemented, as schematically represented in Fig. 3.
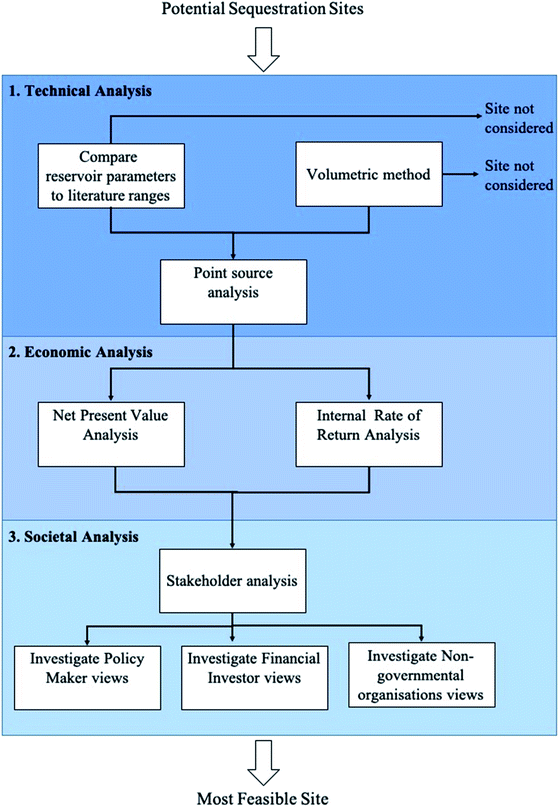 |
| Fig. 3 Workflow for the proposed technical, economic and societal analysis. | |
(1) Conduct a volumetric estimation to quantify the capacity of the site compared to the amount of CO2 produced towards estimating the duration of a possible project.
(2) Quantify the economic feasibility based on existing carbon tax implications.
(3) Perform qualitative country-specific social analysis, to understand existing resistance and possible support for the implementation of carbon sequestration demonstration projects.
2.1. Technical analysis
2.1.1. Reservoir parameters and ranking criteria.
A ranking exercise was performed to assess the technical feasibility of each site. The ranking was produced based on optimal ranges extracted from literature for each of the parameters listed in Table 2. Parameters that fall within the optimal value range are given a rank of 5, while non-optimal values, estimated to be within the cautionary conditions, are given a rank of 1. Other values between the positive and cautionary ranges are ranked in-between. For example, because offshore sites entail more costly facilities and requirements for intensive monitoring,67 they are regarded as less than optimal and are therefore ranked at 3; on the contrary less cost-intensive onshore sites are ranked at 4.
2.1.2. Volumetric method.
Assuming that the injected CO2 will fully replace the reservoir fluid in place (i.e., hydrocarbons), we adopted the volumetric method to estimate the theoretical capacity for carbon storage in each field.68 For completeness, it should be noted that due to the presence of reservoir fluids and geological heterogeneities, the value calculated by this method likely corresponds to the maximum capacity achievable within a given field. Following the best practices identified by the US Department of Energy,68 the method implemented calculates the original gas in place (OGIP), via: | G = A × Th × φ × (1 − Sw) × B. | (1) |
In eqn (1), G is the storage capacity in m3, A is the field area in m2, Th is the field thickness in m, φ is the average reservoir porosity in %, Sw is the reservoir water saturation. B is the gas formation volume factor in Rm3 per Sm3 (reservoir cubic metres per standard cubic meters); based on literature information, B was assumed to be 1.46 Rm3 per Sm3 for carbon sequestration in hydrocarbon formations.69
To estimate the mass of CO2 that could be stored, the following equation is used:
| Q = A × Th × φ × (1 − Sw) × B × ρCO2 | (2) |
In
eqn (2),
Q is the mass capacity in tonnes, where
ρCO2, the CO
2 density in kg m
−3, is calculated by the Span–Wagner equations of state.
137
2.1.3. Point source analysis and estimate of the sequestration project duration.
We identify potential CO2 point sources for the possible geological sites. It is noted that in our analysis (1) direct CO2 capture from air is not considered as a carbon source here due to its expected high costs; (2) in the event the CO2 source was to close down unexpectedly, the sequestration project is considered affected, yielding financial risks. From this perspective, we choose relatively new industrial operations as optimal point sources of CO2, because they are considered to offer geographical advantages to the geological sequestration. Finally, the injection rate in the potential sites was assumed to be similar to the CO2 emission rate of the nearby facilities; shallow locations were chosen for ease of operations.
An estimate of the duration of the geological sequestration project (limited by the capacity of the geological formation as estimated from eqn (1) and (2)) will be useful for assessing financial risks and implications, as discussed later in the analysis of the net present value. To estimate the lifetime of the sequestration project, i.e., time to reach CO2 capacity (tmax), we divided the estimated mass capacity by the rate of injectivity per well, multiplied by the number of wells available for the process:
|  | (3) |
where
Q is the capacity of the field in tonnes,
E is the rate of injectivity per well in tonnes/year, and
N is the number of wells.
2.2. Economic analysis
We implement a preliminary AACE (Association for the Advancement of Cost Engineering) Class 5 Cost Estimate.70 This approach factors in capacity and parametric models,91 thereby providing valuable information for project screening and selection. The data has been collated and reported in ESI.† The results will be compared to the carbon tax to assess the net present value for the different projects, as well as for ranking the correspondent internal rates of return.
2.2.1. Main assumptions.
(1) Lifetime of the projects are estimated initially as 20 years, taken as the average injection period of past projects.91 Note that this assumption holds as long as eqn (4) yields a value larger than 20 years, otherwise a different value should be used. (2) The capital investments are staggered over a period of ten years, following suggestion by the UK Department for Business, Energy and Industrial Strategy on business models for Carbon Storage.71 (3) The annual carbon tax credit enforced by governmental policy in each country is based on disposing of 50% of the total yearly point source emissions.67 (4) Operational costs are approximated as fixed per site per year for the 20 year period. (5) The single source of revenue for the project is due to carbon tax credits. (6) Costs related to post-injection monitoring and well plugging are included in the analysis.
To calculate the costs for the implementation of carbon sequestration at the potential sites, the following interventions are considered:72
(1) Geological site surveys
(2) Well construction
(3) Old well remediation
(4) Post injection requirements
(5) Operating, monitoring and maintenance
Annual revenue is calculated as:
where In is the annual income, dependent entirely on the carbon tax credit, and OpEX is the annual operational cost for each site where monitoring costs are evaluated in Table S6 of ESI.
†
The Net Present Value (NPV) is calculated by:135
| 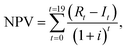 | (5) |
where
t is the number of years into the future and
i is discount factor.
t = 0 is the immediate time at the start of the project and
t = 19 is the time at the end of the project lifetime,
i.e. 20 years.
The Norwegian Environmental Agency (NEA) advises implementing a discount rate (i) of 4% for NPV analysis over the first 40 years duration of a project.73 For simplicity, this value is implemented for all sites considered here. This choice is consistent with literature studies on globally inclusive discount rates, which implement values between 2% and 5%.74 Because projected inflation rates for the three countries within which the sites of Table 5 are located are between 2 and 3%, an average 2.5% inflation rate is considered for the analysis, which provides an adjusted discount rate of 6.6%.75–77
The internal rate of return (IRR) is estimated by implementing a goal-seek algorithm to satisfy the condition that NPV = 0, by changing the discount rate. IRR is useful to potential investors since it is independent of project scale, while NPV is not. Investors with options to invest elsewhere will have a minimum threshold for an acceptable IRR.
3. Results and discussion
3.1. Technical analysis
The key technical parameters which must be maintained within suitable ranges are discussed in Section 1.4. Data for each of the five sites have been collated for analysis in Table 6. In Table 7, we provide our ranking of the properties of interest. It should be stressed that this ranking is somewhat subjective. Nevertheless, it helps us compare the possible sites.
Table 6 Reservoir parameters for the five potential sites identified in Table 5 (parameters representative of cautionary conditions are marked with (*))
Site |
Cantarell, Mexico |
Oloibiri, Nigeria |
Frigg, Norway |
Rio Vista, USA |
Romashkino, Russia |
Field index |
I |
II |
III |
IV |
V |
Location |
Offshore |
Onshore |
Offshore |
Onshore |
Onshore |
Depth (m) |
1500 (ref. 30) |
3660* (ref. 57) |
1850 (ref. 59) |
1367 (ref. 60) |
1800 (ref. 83) |
Permeability (Darcy) |
3–4 |
1.94 |
1–5 (ref. 81) |
1 (ref. 60) |
1–3.5 (ref. 83) |
Interval thickness (m) |
100 (ref. 30) |
6–18* (ref. 79) |
10–80 (ref. 82) |
15–100 (ref. 79) |
30–40 (ref. 83) |
Porosity (%) |
12–20* (ref. 78) |
19–24 (ref. 79) |
27–32 (ref. 81) |
19–35 (ref. 79) |
14–24 (ref. 83) |
Temperature (°C) |
67.7 (ref. 78) |
122 (ref. 80) |
60 (ref. 82) |
81 (ref. 60) |
65 (ref. 83) |
Pressure (bar) |
190.83 (ref. 78) |
350 (ref. 80) |
223.4 (ref. 81) |
210 (ref. 60) |
120 (ref. 83) |
CO2 density (kg m−3) |
648.52 |
613.30 |
756.70 |
612.32 |
382.88* |
Fluid state |
Supercritical |
Supercritical |
Supercritical |
Supercritical |
Supercritical |
Initial water saturation (%) |
21 (ref. 30) |
33 (ref. 79) |
26 (ref. 82) |
20 (ref. 60) |
19 (ref. 83) |
Table 7 Ranking of the reservoir parameters for each site considered
Site |
Cantarell |
Oloibiri |
Frigg |
Rio Vista |
Romashkino |
Field index |
I |
II |
III |
IV |
V |
Depth |
4 |
1 |
4 |
4 |
5 |
Permeability |
5 |
4 |
4 |
3 |
4 |
Thickness |
5 |
1 |
3 |
4 |
2 |
Porosity |
3 |
4 |
5 |
5 |
4 |
Density |
4 |
4 |
5 |
4 |
1 |
Off/onshore |
3 |
4 |
3 |
4 |
4 |
Based on data shown in Tables 6 and 7, it appears that the Oloibiri and Romashkino sites are sub-optimal, because of their inferior formation thickness and depth, and CO2 densities, respectively, compared to the other sites considered. (1) Low thickness can lead to risks of loss of containment, while deep formations imply much high drilling costs, which could lead to an economically infeasible project. (2) Because high CO2 densities are advantageous for optimum storage, low density could limit the storage capacity of the site and also lead to high CO2 mobility. To complement the characterisation of the potential sites, their storage capacities were estimated, and the results are summarised in Table 8. The results confirm that the storage capacity in the Oloibri and in the Romashino sites is estimated to be lower than that in the remaining three sites (Cantarell, Frigg and Rio Vista), which received satisfactory rankings for most parameters considered in Table 7.
Table 8 Estimated maximum CO2 storage capacity of the field sites shortlisted in Table 5
Site |
Cantarell78 |
Oloibiri84 |
Frigg85 |
Rio Vista60 |
Romashkino45 |
Field index |
I |
II |
III |
IV |
V |
Country |
Mexico |
Nigeria |
Norway |
USA |
Russia |
Reservoir area (m2) |
1.62 × 108 |
1.375 × 107 |
1.15 × 108 |
1.2 × 108 |
4.2 × 107 |
(1 − Sw) (%) |
79 |
67 |
73.8 |
80 |
81 |
G (m3) |
1.28 × 109 |
2.38 × 107 |
1.13 × 109 |
1.44 × 109 |
2.59 × 108 |
Q (kg) |
8.30 × 1011 |
1.46 × 1010 |
8.53 × 1011 |
8.79 × 1011 |
2.12 × 1011 |
Q (tonne) |
8.30 × 108 |
1.46 × 107 |
8.53 × 108 |
8.79 × 108 |
2.12 × 108 |
It is highly desirable for a potential field site to hold significant volumes of sequestered CO2; this will improve the technical feasibility of the project. Furthermore, larger amounts stored are likely to offset capital costs faster, reducing the average cost of storing CO2, thereby ensuring an acceptable return on investment. The results in Table 8 show that the largest storage capacities are offered by the Cantarell, Frigg and Rio Vista sites. It should however be recognised that the real storage field capacity is somewhat lower than the upper limits estimated in Table 8 due to uncertainty in storage volume estimates,67 and due to the presence of other fluids within the formation.
Out of the three promising sites identified so far, Frigg and Rio Vista fields exhibit all characterisation parameters (Tables 6 and 7) within desirable ranges; on the contrary, the porosity of the Cantarell field is on the lower limit of the desirable range. The latter shortcoming was considered minimal in our analysis because of the large storage capacity offered by the Cantarell site (Table 8).
For the three most attractive sites as identified so far, an analysis was carried out to identify potential point-source large CO2 emitters. The results, shown in Table 9, suggest that all three sites are within a reasonable distance from concentrated sources of CO2, which entails moderate transportation costs. It should be noted that all the point-sources identified in Table 9 are located in proximity of additional refineries and power plants, suggesting that additional CO2 emissions can be used, if necessary. To calculate the duration to fill the field, the rate of injectivity for each well is employed from literature as 260 tonnes/year assuming 255 operational days in the year.86 It is worth repeating that this is an estimate. To change the duration of a project one could for example use CO2 from multiple emitters and use several injection sites within a formation. However, to decide injection rates one should also consider possible risks of induced seismicity, as it has been reported that injecting water in the subsurface with high flow rates could trigger seismic events.134 Excluding safety and technical feasibility considerations, increasing the injection rate could shorten the duration of the project, anticipating possible revenues expected form the carbon tax. Analysis on the sensitivity of NPV and IRR on this parameter has not been conducted herein, but it could be done with straightforward modifications of the model developed here.
Table 9 Point source large CO2 emitters in geographical proximity of the three shortlisted geological sites based on the analysis of Tables 6 and 7
Site |
Field index |
Point source |
Sector |
Distance from point source to field (km) |
Point source emissions, E (tonnes year) |
Injectivity rate86 (tonnes per day) |
Years to fill the field |
Cantarell Mexico |
I |
CFE Central Termoeléctrica Adolfo Lopez Mateos |
Electricity generation |
Field is 80 km offshore |
4.3 × 106 (ref. 87) |
9360 |
348 |
Power station is 91.9 km from the harbour |
Total is 171.9 km |
Frigg Norway |
III |
Equinor ASA Mongstad |
Oil & gas |
Field is 209 km offshore |
4.0 × 106 (ref. 88) |
8320 |
403 |
Mongstad refinery is 4 km from the harbour |
Total is 303 km |
Rio Vista USA |
IV |
Chevron Refinery California |
Oil & gas |
By land 93 km |
4.8 × 106 (ref. 89) |
10 400 |
332 |
3.2. Economic analysis
The analysis of capital costs, inclusive of preparatory work conducted to enable the sites to be functional, geological modelling, survey of existing wells as well as upgrading of the existing wells to enable CO2 injection, drilling of new wells and other costs related to maintaining the wells after injection is completed is presented in ESI,† together with assumptions made to estimate the various cost components. In Table 10, we summarise the resultant capital costs estimated for the shortlisted three sites.
Table 10 Summary of capital and operational costs estimated for the three field sites. The capital costs are expressed on a yearly basis to reflect that capital operations will be spread over the first decade of operations, following suggestion by the UK Department for Business, Energy and Industrial Strategy on business models for Carbon Storage71
Site |
Cantarell |
Frigg |
Rio Vista |
Field index (Table 4) |
I |
III |
IV |
Capital cost (M$ per year) |
4.5 |
5.2 |
2.7 |
Operational costs (M$ per year) |
10.285 |
13.120 |
15.653 |
The operational costs are estimated for the three sites on an annual basis. In our analysis, we accounted for labour costs,116–122,133 monitoring and maintenance, mechanical testing and detection and finally surveying.123–128,132 The details of the calculations, together with assumptions made, are reported as ESI.† The resultant operational costs per annum are reported in Table 10 for the three sites of interest. In our analysis, these costs are considered constant throughout the 20 years of operation.
As mentioned above, income for the operations considered here is assumed to only be due to carbon tax offset. The carbon tax is a fee imposed by governments that emitters must pay based on each tonne of CO2 released by various private enterprises.90 Because the successful execution of carbon sequestration will prevent CO2 release to the atmosphere, the industrial emitters whose CO2 is being captured and sequestered are expected to save the relevant carbon taxes. In our analysis, profit for the operator engaged in the sequestration project is based on the difference between the carbon tax imposed by the country where the operation occurs, and the operational costs, inclusive of depreciation, for storing carbons. The carbon tax is country and sometimes location specific. For example, the Rio Vista Site, located in the USA, is eligible for the 45Q carbon sequestration tax credit policy as the CO2 is being permanently buried in our model assumption.91 In Table 11 we report our estimates for the carbon tax as relevant for the three sites considered here, and in Table 12 we report our estimates for the NPV for the three sites.
Table 11 Carbon tax analysis relevant for the three sites considered
Site |
Cantarell95 |
Frigg98 |
Rio Vista96 |
Field index |
I |
III |
IV |
Norway has higher than average tax rates on electricity generation and the industrial sector.
California enforces a cap-and-trade system, it is mainly enforced on electric power plants and the industrial sector. The system places carbon allocation allowances on systems producing at least 25 000 tonnes of CO2.
|
Carbon tax |
$3.50 per tonne of CO2 released |
$57 per tonne of CO2 releaseda |
$10-$50 per ton of CO2b |
Amount of emissions from point source per year |
2.15 × 106 tonnes |
2 × 106 tonnes |
2.4 × 106 tonnes |
CO2 sequestered per year per well calculated using total number of wells Table S2 |
59 722 tonnes |
62 500 tonnes |
60 000 tonnes |
Table 12 Net present value over the 20 year period for the three sites shortlisted in Tables 6 and 7
Site |
Cantarell |
Frigg |
Rio Vista |
Field index |
I |
III |
IV |
Net present value ($M) |
−420 |
486 |
362 |
Our analysis does not include the costs related to carbon capture. This approach complements recent reports by Wilcox and co-workers which outline the costs of carbon capture from major industries and the sequestration processes whilst taking into account federal US tax credits,92 which yield costs in the order of $22–26 per tonne of CO2 sequestered.93 Past studies94 suggest that sequestration cost only occupies 20% of the total CCS costs. Based on this information, we evaluate the remaining portion of CCS transportation and capture costs, as presented in the ESI.†
3.2.1. Rates of return.
Using the total capital investments estimated for each project, and the total return achievable based on the profit and annual operational cost of three sites during their 20 years of operation, it is possible to estimate the internal rates of return (IRR). The IRRs calculated for Frigg and Rio Vista are ∼27% and ∼23%, respectively. These are very attractive IRRs as compared to 16.7%, which is the average IRR for almost 90% of global sequestration projects that reported emission reductions.97 For the Frigg site, this high IRR is attributed to high carbon tax credits; for the Rio Vista site, the high IRR is due to the lowest depth requirement to reach the formation, which implies the lowest well construction costs. Using our estimates, the Frigg site yields the highest IRR, which seems to be consistent with the vast expansion of CCS projects in Norway. Within this landscape, it seems appropriate to point out that the Norwegian government is considering tripling the CO2 tax credits by 2030, further increasing attractiveness for sequestration projects.98 In our analysis, the Cantarell site is unable to yield an attractive IRR within the 20 year lifetime, this can be attributed to the considerably low carbon tax credit available for this site. The different IRR for the three sites considered here highlights the importance of the stability, certainty, and magnitude of carbon tax credits for the economic sustainability of carbon sequestration projects.
To complement our study, a sensitivity analysis was carried out for a scenario in which carbon tax credits available for the Cantarell site corresponds to an average of those available for Frigg and Rio Vista sites (e.g., $43.5 per tonne of CO2). The results yield a significant hypothetical IRR of 26%, more attractive than those estimated in the other sites. This high IRR is due to the low labour costs expected at the Cantarell, compared to the other sites. Within this landscape, it is worth pointing out that the Mexican government increased carbon tax credits to $30 in 2008, much higher than the value considered in Table 11. Unfortunately, however, due to a wide economic crisis, industrial activity recessed and the carbon tax rate was subsequently lowered.
3.2.2. Net present value.
To further assess the relative attractiveness of the three field sites, the discounted cumulative cash flow is calculated. As seen in Fig. 4, the payback period is ten years for the Rio Vista site and nine years for Frigg. Within the 20 year lifetime, the Cantarell site does not reach the break-even point. Based on a sensitivity analysis, we conclude that for the Cantarell site to reach a break-even within the 20 year lifetime, the carbon tax credit would need to be increased to $13.6 per tonne of CO2 or above.
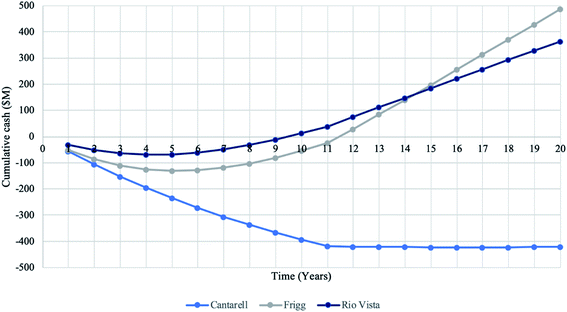 |
| Fig. 4 Cumulative discounted cash flow analysis for the three sites considered based on analysis shown in Tables 6 and 7. | |
At this stage of analysis, both the NPV and the IRR suggest that the Frigg and the Rio Vista operations are attractive. Certainly, however, these results are based on many assumptions; further research and more detailed costing must be carried out to ascertain these conclusions and better define OpEx and CapEx.
3.3. Social acceptability analysis
Based on the above, two out of the three sites considered are technically and economically feasible. However, as outlined above, this is insufficient to complete a project, unless a social license to operate is acquired and maintained. Akin to the ideas presented by Dowd outlining the requirement to consider stakeholder perspective for the long-term success of CCS,99 a study into the expected Stakeholder groups' views, specific for the three countries of interest, has been carried out. The results are summarised in Table 13.
Table 13 Country-specific stakeholder group analysis for the three sites considered in Fig. 4
Stakeholder group |
Mexico |
Norway |
USA |
Policy makers (nationwide) |
• In the Paris agreement promise to reduce greenhouse gas emissions by 50%, 19% of its mitigation strategy will be focussed on carbon capture and storage |
• Plans to be carbon neutral by 2030 and carbon capture projects play a major role to achieve this goal101 |
• The US Environmental Protection Agency (US EPA) regulates greenhouse gas emissions under the Clean Air Act as an endangerment to public health, however they lack authority to address problems including pipeline access, long term site access and pore ownership101 |
• Work is being made to produce a carbon capture and storage roadmap which is currently in the research stages and is projected to launch in 2024 |
• In 1991 taxes were set on CO2 emissions from fuels and the petroleum industry, however the effectiveness of the law was debatable due to extensive tax exemptions, with a resultant decrease in onshore emissions by only 1.5%101 |
• Currently no legal basis exists purely for carbon capture and storage (CCS) activities it is assumed these activities are covered by relating existing regulations set for outside the sector, work is still to be made to produce a sector specific regulatory framework100 |
• Well-developed legal framework is in place for petroleum industries it has been tailored specifically for CCS101 |
Policy makers (municipality) |
• Regulations for CCS in Mexico are based on a nation-wide scale |
• Municipalities in Norway are highly supportive of CCS. the Oslo municipality is currently assessing the capture of 315 000 tonnes of CO2 from a local power plant. Extensive studies are carried out into other opportunities for CCS102 |
• US states implement independent climate change measures for example California implements The California Global Warming Solutions Act of 2006, which hopes to decrease emissions by 80% by 2050. This will be accomplished through voluntary actions, incentives and market tools101 |
Financial Investors |
• The world bank implemented a project to provide aid of $50m to develop CCS in Mexico in 2018. This is subsidised by the Norwegian and UK government103 |
• Norwegian government has proposed to invest £54m to carbon sequestration projects from 2020 (ref. 104) |
• The US government has invested $72m in carbon capture technologies in 2020106 |
• There is no data available on substantial governmental investment in CCS |
• Equinor runs the petroleum refinery CO2 point source. They have engaged in many CCS partnerships and are currently evaluating storage off the Norwegian shelf. The company could be a prime investor in the project105 |
• Point source is managed by chevron, the company supports the Paris agreement, with goals to decrease GHG emissions. However, their current CCS project in Australia has been unsuccessful and the company are accountable to pay at least – in carbon offsets, it is unlikely the company would be interested in currently investing in another ccs project |
• The point source CFE Central Termoeléctrica Adolfo Lopez Mateos is owned by the government, as there is not much governmental investment into CCS it is unlikely there will be substantial support |
Non-Governmental Organizations |
• Currently no substantial data is available on Mexican NGO's opinion on carbon sequestration |
• NGOs in Norway; Young Friends of the Earth, believe that CCS is required to reduce greenhouse gases131 |
• Organizations in the US are not as assured about the effectiveness of CCS. As above-mentioned groups such as Green Peace view CCS as unrealistic to delivery carbon emissions reduction goals |
Local Communities |
• It is believed that a CCS project will improve the atmospheric pollution and environmental quality for inhabitants in the immediate region108 |
• In contrast to other European countries locals in Norway display very positive attitudes towards CCS109 |
• Local communities are ambivalent towards CCS projects, this may lead to opposition due to due to costs on the US economy110 |
3.4. Summary of case studies
3.4.1. Cantarell, Mexico.
In our analysis, regulations available in Mexico somewhat lack in specificity for CCS projects; considering the long duration of a successful geological sequestration project, significant government support, clarity and transparency are essential for geological carbon sequestration projects. There are approximately ten past and current sequestration projects in depleted hydrocarbon reservoirs.18 Of these, only one has been successfully implemented in developing countries, i.e., In-Salah in Algeria. The limited number of sequestration projects in developing countries is attributed to a variety of factors, including the lack of skilled human capacity, understanding of constraints, and the lack of favourable incentives. Another critical impediment is limited access to adequate capital funding. These general observations, combined with the socio-economic assessment conducted above for the Cantarell Mexico site, suggest that these barriers might explain why the Cantarell site has not yet been developed. The barriers to the implementation of CCS in developing countries have been recognized by The World Bank.111 The World Bank is currently working on test injection projects within developing countries, in particular in South Africa. In the future, a deeper understanding of CCS operations will ascertain success for sequestration projects in developing countries and will encourage international funding.
3.4.2. Frigg, Norway.
As outlined in Table 13, the social perceptions in Norway are highly supportive of the development of CCS where there is ample governmental support and funding seems to be available for sequestration projects. Local policymakers also highly encourage CCS projects, and positive opinion regarding CCS is shared among external groups including local communities and NGO's. According to the Global CCS Institute's Carbon Capture and Storage Readiness Index 2018, Norway is one of the five highest-scoring nations in CCS pioneering models. This is due to the establishment of national and state energy regulatory frameworks, substantial investment initiatives, and consistent incentives in CCS in the past two decades.112 As outlined above, learnings from the Sleipner project have been continuous and successful in Norway.129 Following suit, a joint sequestration venture between Equinor, Shell and Total is currently underway. The Longship project aims to store 0.8 million tonnes of CO2 per year in reservoirs beneath the sea bottom. Of the required $2.7 billion overall costs to fund the project, the Norwegian government has offered $1.8 billion.113 The Longship project provides evidence according to which ample governmental local operational support is likely to be available.
3.4.3. Rio Vista, USA.
The USA is also one of the five highest-ranking countries in the CCS readiness index.114 However, operations in the USA currently could face variable levels of higher-level governmental support; in addition, the state of California seems to provide substantial encouragement for carbon sequestration projects, although this might not translate into the necessary capital investment. Governmental support is of utmost importance and can sway that of the public. Moving forward, it is crucial for the government and other municipal lawmakers to provide the public with sufficient information about the benefits of CCS projects.115 In the past, several CCS projects have been conducted in depleted hydrocarbon reservoirs in the US; Weyburn, Cranfield and the SACROC projects, which led to a significant body of knowledge and technical understanding.130 Although recent investment in CCS has been rather small, with multiple investments up to $1 million for CCS enhancement,116 in 2020, the US Department of Energy promised $110 million for research and development of CCS projects, suggesting that further research and development will be supported in the near future.
4. Conclusions
Several scenarios have been proposed for our society to achieve the goals of the Paris Agreement. Carbon capture and sequestration is an essential component of all scenarios that could lead to achieving the goals of such agreement. However, few demonstration sites are being developed around the globe.
In this paper, information from existing pilot field sites is reviewed, leading to the conclusion that geological carbon sequestration is generally considered to be technically feasible.
Building on the analysis provided for example by Wilcox and Hannis18 regarding the costs associated with carbon capture, we provide a discussion of our threefold, in-depth feasibility study on three geological carbon sequestration sites. The successful and safe operability of a CCS project is of utmost importance, therefore the primary assessment for site feasibility must be based on a technical analysis. In our analysis, over the 20 year period considered for our class 5 estimates, assuming the current single point source emissions, we estimate an average cost of $7.25 per tonne of CO2 sequestered, which is within the range of carbon sequestration costs reported in the literature, from $2.48 to $28.12 per metric tonne of CO2 processed.111 The case studies in the literature prove that the societal views have been the ultimate deciding factor in the success of multiple sequestration projects, as evidenced by the failure of the Schwartz, Pump project and Dutch Barendrecth project due to lack of stakeholder support. We conclude that a convergence of technical feasibility, economic attractiveness, and social acceptability needs to be achieved for pilot carbon sequestration projects to be initiated. In this landscape, our results point to the importance of carbon tax in enhancing the attractiveness of projects for the long-term sequestration of CO2 in geological repositories.
Conflicts of interest
There are no conflicts of interest to declare.
Acknowledgements
This project was supported, in part, by the UCL Chemical Engineering Department. Funding from the European Commission via Consortium Agreement number 764810, which supported the consortium Science4CleanEnergy, is gratefully acknowledged.
References
-
H. Ritchie and M. Roser, CO2 and other Greenhouse Gas Emissions, Our World in Data, 2017, https://ourworldindata.org/co2-and-other-greenhouse-gas-emissions Search PubMed
.
-
NASA, The Causes of Climate Change, Climate Change: Vital Signs of the Planet, NASA, 2018, https://climate.nasa.gov/causes/ Search PubMed
.
-
E. Rubin, Carbon dioxide capture and storage intergovernmental panel on climate change carbon dioxide capture and storage, https://www.ipcc.ch/site/assets/uploads/2018/03/srccs_wholereport-1.pdf Search PubMed
.
-
What is Carbon Sequestration, http://www.kgs.ku.edu/Midcarb/sequestration.shtml Search PubMed
.
-
Geological sequestration, Climate Change Connection, 2020, https://climatechangeconnection.org/solutions/carbon-sequestration/geological-sequestration/ Search PubMed.
-
CO2 Emissions - Worldometers, Worldometers.info., 2016, https://www.worldometers.info/co2-emissions/ Search PubMed.
-
S. Goulding and J. Esterie, Comparative review of carbon geosequestration options, Asia Pacific Coalbed Methane Symposium, 2008 Search PubMed
.
-
M. Phyoe Wai Aung, Analysis on EOR/CO2 Sequestration in Sacroc Unit, Texas Using A Compositional Simulator, Faculty of Petroleum Engineering Department, New Mexico Institute of Mining and Technology, 2009 Search PubMed
.
-
https://www.imperial.ac.uk/media/imperial-college/grantham-institute/public/publications/briefing-papers/Carbon-dioxide-storage----Grantham-BP-4.pdf
.
-
Barriers to overcome in implementation of CO2 capture and storage, 2000, https://ieaghg.org/docs/General_Docs/Reports/PH3_22%20Storage%20in%20oil%20and%ields.pdf Search PubMed.
- M. Jafari, S. C. Cao and J. Jung, Geological CO2 sequestration in saline aquifers: Implication on potential solutions of China's power sector, Resour., Conserv. Recycl., 2017, 121, 137–155 CrossRef
, https://www.sciencedirect.com/science/article/pii/S0921344916301240.
-
L. Tomić, V. K. Maričić, D. Danilović and M. Crnogorac, Criteria for CO2 storage in geological formations, Podzemni Radovi, 2018 (32):61–74, http://ume.rgf.bg.ac.rs/index.php/ume/article/view/116 Search PubMed
.
- J. Q. Shi and S. Durucan, CO2 Storage in Deep Unminable Coal Seams, Oil Gas Sci. Technol., 2005, 60(3), 547–558 CrossRef CAS
, https://hal.archives-ouvertes.fr/hal-02017220/document.
-
Basalt - an Overview, ScienceDirect Topics, Sciencedirect.com, 2017, https://www.sciencedirect.com/topics/earth-and-planetary-sciences/basalt Search PubMed
.
- S. R. Gislason and E. H. Oelkers, Carbon Storage in Basalt, Science, 2014, 344(6182), 373–374 CrossRef CAS PubMed
.
- J. M. Matter, W. S. Broecker, S. R. Gislason, E. Gunnlaugsson, E. H. Oelkers and M. Stute,
et al., The CarbFix Pilot Project–Storing carbon dioxide in basalt, Energy Procedia, 2011, 4, 5579–5585 CrossRef CAS
.
-
R. B. Ghshfarokhi, S. Matson and M. Linroth, Overview of CO2 Injection and WAG Sensitivity in SACROC, Society of Petroleum Engineers, 2016 Search PubMed
.
- S. Hannis, J. Lu, A. Chadwick, S. Hovorka, K. Kirk and K. Romanak,
et al., CO2 Storage in Depleted or Depleting Oil and Gas Fields: What can We Learn from Existing Projects?, Energy Procedia, 2017, 114, 5680–5690 CrossRef CAS
, https://www.sciencedirect.com/science/article/pii/S1876610217319082.
- J. W. Carey, M. Wigand, S. J. Chipera, G. WoldeGabriel, R. Pawar and P. C. Lichtner,
et al., Analysis and performance of oil well cement with 30 years of CO2 exposure from the SACROC Unit, West Texas, USA, Int. J. Greenhouse Gas Control, 2007, 1(1), 75–85 CrossRef CAS
.
- W. S. Han, B. J. McPherson, P. C. Lichtner and F. P. Wang, Evaluation of trapping mechanisms in geologic CO2 sequestration: Case study of SACROC northern platform, a 35-year CO2 injection site, Am. J. Sci., 2010, 310(4), 282–324 CrossRef CAS
, http://www.ajsonline.org/content/310/4/282.abstract.
- A. J. Cavanagh and R. S. Haszeldine, The Sleipner storage site: Capillary flow modeling of a layered CO2 plume requires fractured shale barriers within the Utsira Formation, Int. J. Greenhouse Gas Control., 2014, 21, 101–112 CrossRef CAS
, https://www.sciencedirect.com/science/article/pii/S1750583613004192.
- A.-K. Furre, O. Eiken, H. Alnes, J. N. Vevatne and A. F. Kiær, 20 Years of Monitoring CO2-injection at Sleipner, Energy Procedia, 2017, 114, 3916–3926 CrossRef CAS
.
- O. Eiken, P. Ringrose, C. Hermanrud, B. Nazarian, T. A. Torp and L. Høier, Lessons learned from 14 years of CCS operations: Sleipner, In Salah and Snøhvit, Energy Procedia, 2011, 4, 5541–5548 CrossRef
.
-
FLEXIS secure €2M funding to expand its research into reducing the CO2 in Our atmosphere, Flexis, 2020, https://www.flexis.wales/blog/flexis-secure-e2m-funding-to-expand-its-research-into-reducing-the-co2-in-our-atmosphere-2/ Search PubMed.
-
CCS best practices, Globalccsinstitute.com, 2021, https://www.globalccsinstitute.com/archive/hub/publications/159708/best-practices-monitoring-verification-accounting-co2-stored-deep-geologic-formations.pdf Search PubMed.
-
CO2 Storage in Deep Unminable Coal Seams, Hal.archives-ouvertes.fr, 2021, https://hal.archives-ouvertes.fr/hal-02017220/document Search PubMed.
- H. Kristjánsdóttir and S. Kristjánsdóttir, Carbfix and Sulfix in Geothermal Production, and the Blue Lagoon in Iceland: Grindavík Urban Settlement, and Volcanic Activity, Baltic J. Econ. Stud., 2021, 7(1), 1–9, DOI:10.30525/2256-0742/2021-7-1-1-9
.
- S. Ó. Snæbjörnsdóttir and S. R. Gislason, CO2 Storage Potential of Basaltic Rocks Offshore Iceland, Energy Procedia, 2016, 86, 371–380 CrossRef
, https://www.sciencedirect.com/science/article/abs/pii/S1750583606000041.
-
U. N. Environment, Carbon Dioxide Capture and Storage, UNEP - UN Environment Programme, 2017, https://www.unenvironment.org/resources/report/carbon-dioxide-capture-and-storage Search PubMed
.
-
D. Romo, The Cantarell Oil Field and the Mexican Economy, Revista Problemas del Desarrollo, 2015, vol. 46, http://www.scielo.org.mx/pdf/prode/v46n183/0301-7036-prode-46-183-00141-en.pdf Search PubMed
.
- S. M. Benson and D. R. Cole, CO2 Sequestration in Deep Sedimentary Formations, Elements, 2008, 4(5), 325–331 CrossRef CAS
, https://www.geo.arizona.edu/%7Ereiners/geos195K/CO2Sequestration_Benson_ELEMENTS.pdf.
- S. Bachu, Screening and ranking of sedimentary basins for sequestration of CO2 in geological media in response to climate change, Environ. Geol., 2003, 44(3), 277–289 CrossRef CAS
.
-
S. Bachu, Screening and ranking of hydrocarbon reservoirs for CO2 storage in the Alberta Basin, Canada, In US Department of Energy–National Energy Technology Laboratory, National Conference on Carbon Sequestration, 2001, vol. 67 Search PubMed
.
- A. Raza, R. Rezaee, R. Gholami, C. H. Bing, R. Nagarajan and M. A. Hamid, A screening criterion for selection of suitable CO2 storage sites, J. Nat. Gas Sci. Eng., 2016, 28, 317–327 CrossRef CAS
, https://espace.curtin.edu.au/bitstream/handle/20.500.11937/19127/238307_238307.pdf?sequence=2.
- E. S. Rubin, J. E. Davison and H. J. Herzog, The cost of CO2 capture and storage, Int. J. Greenhouse Gas Control, 2015, 40, 378–400 CrossRef CAS
.
-
Can We Make CO2 Capture Profitable?, Global CCS Institute, https://www.globalccsinstitute.com/news-media/insights/can-we-make-co2-capture-profitable/ Search PubMed
.
- M. Fischedick, K. Pietzner, N. Supersberger, A. Esken, W. Kuckshinrichs, P. Zapp and J. Linßen, Stakeholder acceptance of carbon capture and storage in Germany, Energy Procedia, 2009, 1, 4783–4787 CrossRef
.
- A. Peters, False Hope Why Carbon Capture and Storage Won't save the Climate, Energy Environ., 2012 May, 23(2–3), 417–418 Search PubMed
.
-
Global Energy Monitor, Shell's Barendrecht Carbon Capture and Storage Project, Global Energy Monitor, 2019, https://www.gem.wiki/Shell%27s_Barendrecht_Carbon_Capture_and_Storage_Project Search PubMed
.
- A. Kovseck, Screening Criteria for CO2 storage oil reservoirs, Pet. Sci. Technol., 2007, 841–866 Search PubMed
.
-
Foreign Policy, Foreign Policy: The List: Taking Oil Fields Offline, Web.archive.org, 2022, https://web.archive.org/web/20060820080847/https://foreignpolicy.com/story/cms.php?story_id=3567 Search PubMed
.
-
Mindfully, Archive.ph, 2022, https://archive.ph/20120525185811/http://www.mindfully.org/Energy/2005/Burgan-Field-Kuwait12nov05.htm Search PubMed
.
-
Pemex, SEC Filings, Pemex.com, 2022, https://www.pemex.com/en/investors/regulatory-filings/Paginas/sec-filings.aspx Search PubMed
.
-
Engineering Information Administration, Russia Oil reserves, 2007, https://web.archive.org/web/20071230085111/http://www.eia.doe.gov/emeu/cabs/Russia/Oil.html Search PubMed
.
- L. Lu, Z. Liu, H. Liu and Y. Yan, Study on Technical Measures of Romashkino Oil Field after Entering Ultra-High Water Cut Stage, Engineering, 2013, 05(07), 622–628 CrossRef
.
-
Energy Global News, Oloibiri Field January 1956 - Nigeria’s First Commercial Oil, Energy Global News, 2019, http://www.energyglobalnews.com/oloibiri-field-january-1956-nigerias-first-commercial-oil/ Search PubMed
.
- S. Deverel, D. Leighton, C. Lucero and T. Ingrum, Simulation of Subsidence Mitigation Effects on Island Drain Flow, Seepage, and Organic Carbon Loads on Subsided Islands Sacramento–San Joaquin Delta, San Franc. Estuary Watershed Sci., 2017, 15(4), 2 Search PubMed
.
-
A. Kjemperud, Production Curve Modeling, Ccop.or.th, 2004, http://www.ccop.or.th/ppm/document/PHWS3/PHWS3DOC19_kjemperud.pdf Search PubMed
.
-
A. Khan
Country's natural gas production in decline, 2018, https://www.dawn.com/news/1625018 Search PubMed
.
- A. Jellah and M. Alhashi, Decline Curve Analysis in East Almabrouk Field- Case Study, Int. J. Sci. Technol. Res., 2015, 4, 73–79 Search PubMed
.
- M. Höök and K. Aleklett, A decline rate study of Norwegian oil production, Energy Policy, 2008, 36(11), 4262–4271 CrossRef
.
-
MEES, Libya's Zueitina Restarts Zella Field, MEES, 2022, https://www.mees.com/2021/9/24/news-in-brief/libyas-zueitina-restarts-zella-field/31eba2b0-1d37-11ec-9397-33ae2994f290 Search PubMed
.
-
CNPC, Tapping the Potentials of Mature Oilfields—CNPC's Practices in China's Eastern Oilfields, 2022, http://www.cnpc.com.cn/en/speeches/201407/4c0a1bc2867e4b2abcfde8971004405c.shtml Search PubMed
.
-
EIA, EIA: Gulf of Mexico Oil and Gas Production Remains Strong, 2022, https://oilprice.com/Energy/Energy-General/EIA-Gulf-Of-Mexico-Oil-And-Gas-Production-Remains-Strong.html Search PubMed
.
-
C. Oilfield, Gulf of Mexico - Offshore Technology | Oil and Gas News and Market Analysis, https://www.offshore-technology.com/projects/cantarell/ Search PubMed.
-
Greenhouse Gas Emissions Factsheet: Mexico, Climatelinks, 2017, https://www.climatelinks.org/resources/greenhouse-gas-emissions-factsheet-mexico Search PubMed.
-
Nigeria CO2 Emissions - Worldometer, https://www.worldometers.info/co2-emissions/nigeria-co2-emissions/ Search PubMed
.
-
Field: FRIGG, https://www.norskpetroleum.no/en/facts/field/frigg/ Search PubMed.
-
Norway: carbon dioxide emissions 2000-2018, Statista, https://www.statista.com/statistics/449787/co2-emissions-norway/ Search PubMed.
-
C. M. Oldenburg and S. M. Benson, Carbon sequestration with enhanced gas recovery: Identifying candidate sites for pilot study, 2001, https://www.osti.gov/servlets/purl/821005 Search PubMed
.
-
U.S. Emissions, Center for Climate and Energy Solutions, 2017, https://www.c2es.org/content/u-s-emissions/ Search PubMed.
-
Russia: carbon dioxide emissions 1985-2019, Statista, https://www.statista.com/statistics/449817/co2-emissions-russia/ Search PubMed.
- C. P. Consoli and N. Wildgust, Current Status of Global Storage Resources, Energy Procedia, 2017, 114, 4623–4628 CrossRef
.
-
H. Rütters and the CGS Europe partners, State of play on CO2 geological storage in 28 European countries, CGS Europe report No. D2.10, June 2013, p. 89 Search PubMed
.
-
T. Johansson, A. Patwardhan, N. Nakicenovic and L. Gomez-Echeverri, Global Energy Assessment: Toward a Sustainable Future, 2012, vol. 10 Search PubMed
.
-
United Nations, Geologic CO2 storage in Eastern Europe, Caucasus and Central Asia An initial analysis of potential and policy, United Nations Economic Commission For Europe, 2021, https://unece.org/sites/default/files/2021-04/Geologic%20CO2%20storage%20report_final_EN.pdf04/Geologic%20CO2%20storage%20report_final_EN.pdf Search PubMed
.
-
H. Herzog and K. Smekens, Cost and economic potential, IPCC Special Report on Carbon dioxide Capture and Storage, 2006, https://www.ipcc.ch/site/assets/uploads/2018/03/srccs_chapter8-1.pdf Search PubMed
.
-
W. Peck, N. Azzolina and J. Ge, Best practices for quantifying the CO2 storage resource estimates in CO2 enhanced oil recovery, 3rd International Conference on Greenhouse Gas Control Technologies, 2016, vol. 114, pp. 4141–4749 Search PubMed
.
- A. M. AlRassas, S. Ren, R. Sun, H. V. Thanh and Z. Guan, CO2 storage capacity estimation under geological uncertainty using 3-D geological modeling of unconventional reservoir rocks in Shahejie Formation, block Nv32, China, J. Pet. Explor. Prod. Technol., 2021 Jun, 11(6), 2327–2345 Search PubMed
.
-
P. Christensen, L. Dysert, J. Bates, D. Burton, R. Creese, J. Hollmann. Cost Estimate Classification System -As Applied In Engineering, Procurement, and Construction for the Process Industries TCM Framework: 7.3 -Cost Estimating and Budgeting Acknowledgments, https://www.costengineering.eu/Downloads/articles/AACE_CLASSIFICATION_SYSTEM.pdf Search PubMed.
-
Department of Business Energy and Industry Strategy, Carbon Capture, Usage and Storage An update on business models for Carbon Capture, Usage and Storage, 2020, https://assets.publishing.service.gov.uk/government/uploads/system/uploads/attachment_data/file/946561/ccus-business-models-commercial-update.pdf Search PubMed
.
-
Geologic CO 2 Sequestration Technology and Cost Analysis TECHNICAL SUPPORT DOCUMENT, 2008, https://www.epa.gov/sites/production/files/2015-07/documents/support_uic_co2_technologyandcostanalysis.pdf Search PubMed.
-
The Norwegian Full-Scale CCS Demonstration Project Potential for reduced costs for carbon capture, transport and storage value chains (CCS) Gassnova SF, 2020, https://ccsnorway.com/wp-content/uploads/sites/6/2020/07/Report-Cost-reduction-curves-for-CCS-Gassnova-version-2b-1.pdf Search PubMed
.
- J. Emmerling, L. Drouet, K.-I. van der Wijst, D. van Vuuren, V. Bosetti and M. Tavoni, The role of the discount rate for emission pathways and negative emissions, Environ. Res. Lett., 2019, 14(10), 104008 CrossRef CAS
.
-
Statistica, Norway - Inflation rate 2024, Statista, 2018, https://www.statista.com/statistics/327359/inflation-rate-in-norway/ Search PubMed.
-
Statistica, Mexico - Inflation rate 2022, Statista, 2018, https://www.statista.com/statistics/275414/inflation-rate-in-mexico/ Search PubMed
.
-
Statista, U.S. - projected inflation rate 2008-2024, Statista, 2021, https://www.statista.com/statistics/244983/projected-inflation-rate-in-the-united-states/ Search PubMed
.
-
T. Limón-Hernández, G. G. Ponce and C. L. Aguiñaga, Status of the Cantarell Field Development Program: An Overview, Offshore Technology Conference, 2001, https://www.onepetro.org/conference-paper/OTC-13175-MS Search PubMed
.
- M. Ehigiator and N. Chigbata, Geophysical and Well Correlation Analysis of Ogo Field: A Case Study in Niger Delta Basin of Nigeria, Niger. J. Technol., 2017, 36, 729–733 CrossRef
.
-
I. Akpabio, J. Ejedawe and J. Ebeniro, Thermal State of the Niger Delta Basin, PROCEEDINGS, Thirty-Eighth Workshop on Geothermal Reservoir Engineering, 2013, https://pangea.stanford.edu/ERE/pdf/IGAstandard/SGW/2013/Akpabio.pdf Search PubMed
.
-
Abandoned hydrocarbon fields, 2013, https://www.npd.no/en/facts/publications/co2-atlases/co2-atlas-for-the-norwegian-continental-shelf/4-the-norwegian-north-sea/4.2-storage-options-in-the-north-sea/4.2.2-abandoned-hydrocarbon-fields/ Search PubMed.
-
A. De Leebeeck, The Frigg Field reservoir: characteristics and performance. North Sea Oil and Gas Reservoirs, 1987, pp. 89–100 Search PubMed
.
-
L. Lu and Z. Liu, Study on Technical Measures of Romashkino Oil Field after Entering Ultra-High Water Cut Stage, 2013 Jan, vol. 5, pp. 622–628 Search PubMed
.
-
About, Oloibiri Oilfield, dbpedia.org., https://dbpedia.org/page/Oloibiri_Oilfield Search PubMed.
-
F. E. Heritier, A. Conort and E. Mure. Frigg Field--U.K. and Norway Viking Graben, North Sea. archivesdatapagescom, 1990, vol. 23, pp. 69–90, https://archives.datapages.com/data/specpubs/fieldst4/data/a023/a023/0001/0050/0069.html Search PubMed.
- C. M. Oldenburg, S. H. Stevens and S. M. Benson, Economic Feasibility of Carbon Sequestration with Enhanced Gas Recovery (CSEGR), Energy, 2004, 29(9–10), 1413–1422 CrossRef CAS
, https://www.osti.gov/servlets/purl/828128.
- R. Lacy, C. Serralde, M. Climent and M. Vaca, Initial assessment of the potential for future CCUS with EOR projects in Mexico using CO2 captured from fossil fuel industrial plants, Int. J. Greenhouse Gas Control, 2013, 19, 212–219 CrossRef CAS
.
-
B. Kristoffersen and T. Palm, CO2 -infrastructure on the Norwegian-British Shelf ZERO-MEMO, December 2006, https://zero.no/wp-content/uploads/2016/06/co2-infrastructure-on-the-norwegian-british-shelf.pdf Search PubMed
.
-
What are the largest sources of global warming emissions in California? The list is out, The Mercury News, 2009, https://www.mercurynews.com/2009/11/22/what-are-the-largest-sources-of-global-warming-emissions-in-california-the-list-is-out/ Search PubMed.
-
Center for Climate and Energy Solutions, Carbon Tax Basics, Center for Climate and Energy Solutions, 2018, https://www.c2es.org/content/carbon-tax-basics/ Search PubMed.
-
Carbon Sequestration Tax Credit FAQ #1: Who is Eligible for the Credit?, Kirkland & Ellis LLP, https://www.kirkland.com/publications/blog-post/2020/07/carbon-sequestration-tax-credit-faq Search PubMed.
- N. McQueen, P. Psarras, H. Pilorgé, S. Liguori, J. He, M. Yuan, C. M. Woodall, K. Kian, L. Pierpoint, J. Jurewicz, J. Matthew Lucas, R. Jacobson, N. Deich and J. Wilcox, Cost Analysis of Direct Air Capture and Sequestration Coupled to Low-Carbon Thermal Energy in the United States, Environ. Sci. Technol., 2020, 54(12), 7542–7551 CrossRef CAS PubMed
.
- P. Psarras, J. He, H. Pilorgé, N. McQueen, A. Jensen-Fellows, K. Kian and J. Wilcox, Cost Analysis of Carbon Capture and Sequestration from U.S. Natural Gas-Fired Power Plants, Environ. Sci. Technol., 2020, 54(10), 6272–6280 CrossRef CAS PubMed
.
-
United States Environmental Protection Agency, Geologic CO2 Sequestration Technology and Cost Analysis Technical Support Document, 2008 Jun, p. 816 Search PubMed
.
-
Mexico's 3 Big Steps Towards Comprehensive Carbon Pricing, World Resources Institute, 2017, https://www.wri.org/blog/2017/04/mexicos-3-big-steps-towards-comprehensive-carbon-pricing Search PubMed.
- P. Tcvetkov, A. Cherepovitsyn and S. Fedoseev, Public perception of carbon capture and storage: A state-of-the-art overview, Heliyon, 2019, 5(12), e02845 CrossRef PubMed
.
-
The ClimaTe has Changed laTin ameriCa & The Caribbean, https://www.wemeanbusinesscoalition.org/wp-content/uploads/2017/08/The-Climate-Has-Changed_LAC.pdf Search PubMed.
-
Norway proposes €200 per ton CO2 tax by 2030, Bellona.org, 2021, https://bellona.org/news/ccs/2021-02-norway-proposes-e200-per-ton-co2-tax-by-2030 Search PubMed.
- A.-M. Dowd and M. James, A Social Licence for Carbon Dioxide Capture and Storage: How Engineers and Managers Describe Community Relations, Soc. Epistemol., 2014, 28(3–4), 364–384 CrossRef
.
- F. Mourits, N. Kulichenko-Lotz, G. H. González and J. M. Nieta, Overview of World Bank CCUS Program Activities in Mexico, Energy Procedia, 2017, 114, 5916–5932 Search PubMed
.
-
Carbon capture and storage – legal and regulatory framework, United States Energy Association, https://usea.org/sites/default/files/ccc17012011_Carbon%20capture%20and%20storage%20-%20legal%20and%20regulatory%20framework_ccc179.pdf Search PubMed.
-
The Future of CCS in Norway, World Coal Association, 2017, https://www.worldcoal.org/future-ccs-norway Search PubMed.
-
Business Case for Project Extensions, https://aidstream.org/files/documents/CCS-Business-Case-Extension-Sept-17-20180711120738.pdf Search PubMed.
-
Norway proposes to invest £54m in CCS projects in 2020, Energy Live News, 2020, https://www.energylivenews.com/2020/01/08/norway-proposes-to-invest-54m-in-ccs-projects-in-2020/ Search PubMed.
-
Statoil evaluating new CO2 storage project on the Norwegian continental shelf, Equinor.com., 2019, https://www.Equinor.com/en/news/co2-ncs.html Search PubMed.
-
US Government invests $72m in carbon capture technologies, Energy Live News, 2020, https://www.energylivenews.com/2020/09/04/us-government-invests-72m-in-carbon-capture-technologies/ Search PubMed.
-
Global NGO views on CCS and storage of CO2, zeroco2, http://www.zeroco2.no/nyheter/global-ngo-views-on-ccs-and-storage-of-co2 Search PubMed.
- C. Vega-Ortiz, F. Avendaño-Petronilo, B. Richards, R. Sorkhabi, L. Torres-Barragán and N. Martínez-Romero,
et al., Assessment of carbon geological storage at Tula de Allende as a potential solution for reducing greenhouse gas emissions in central Mexico, Int. J. Greenhouse Gas Control, 2021, 109, 103362 CrossRef CAS
.
- J. K. Haug and P. Stigson, Local Acceptance and Communication as Crucial Elements for Realizing CCS in the Nordic Region, Energy Procedia, 2016, 86, 315–323 CrossRef
.
-
P. Parfomark, Community Acceptance of Carbon Capture and Sequestration Infrastructure: Siting Challenges, Congressional Research Service, 2008, https://fas.org/sgp/crs/misc/RL34601.pdf Search PubMed.
-
Global CCS Institute, Making the case for funding carbon capture and storage in developing countries, GlobalCCSInstitute.com, 2013, p. 11 Search PubMed
.
-
F. Almendra, L. West, L. Zheng and S. Forbes, CCS Demonstration in Developing Countries: Priorities for a Financing Mechanism for Carbon Dioxide Capture and Stor, World Resource Institute, 2011, vol. 12 Search PubMed
.
-
F. Almendra, L. West, L. Zheng and S. Forbes, CCS Demonstration in Developing Countries: Priorities for a Financing Mechanism for Carbon Dioxide Capture and Storage, 2011, https://www.globalccsinstitute.com/archive/hub/publications/159663/ccs-demonstration-developing-countries-priorities-financing-mechanism-carbon-dioxide-capture-storage.pdf Search PubMed.
-
N. Rokke, Norway To Build $3 Billion ‘Longship’ Carbon Dioxide Capture Project, Forbes, https://www.forbes.com/sites/nilsrokke/2020/09/21/norways-18-billion-ccs-proposal-is-great-news-for-the-climate/ Search PubMed.
-
“The Carbon Dioxide Dilemma: Promising Technologies and Policies” at NAP.edu, https://www.nap.edu/read/10798/chapter/3#26 Search PubMed.
-
ARPA, FLECCS-FLExible Carbon Capture and Storage, 2019, https://arpa-e.energy.gov/sites/default/files/documents/files/FLECCS_Project_Descriptions.pdf Search PubMed.
-
Institute EER, Geologist Salary Mexico, SalaryExpert, https://www.salaryexpert.com/salary/job/geologist/mexico Search PubMed.
-
Institute EER, Geologist Salary Norway, SalaryExpert, https://www.salaryexpert.com/salary/job/geologist/norway Search PubMed.
-
Institute EER, Exploration Geologist Salary the United States, SalaryExpert, https://www.salaryexpert.com/salary/job/exploration-geologist/united-states Search PubMed.
-
Petroleum Engineer Average Salary in Mexico 2021, The Complete Guide, http://www.salaryexplorer.com/salary-survey.php?loc=139%26loctype=1%26job=573%26jobtype=3 Search PubMed.
-
Engineering Average Salaries in Norway 2021 - The Complete Guide, http://www.salaryexplorer.com/salary-survey.php?loc=162%26loctype=1%26job=22%26jobtype=1 Search PubMed.
-
Petroleum Engineer Salary, PayScale, https://www.payscale.com/research/US/Job=Petroleum_Engineer/Salary Search PubMed.
-
Aeromagnetic Survey, Open Energy Information, https://openei.org/wiki/Aeromagnetic_Survey Search PubMed.
-
Cement Bond Log, Open Energy Information, https://openei.org/wiki/Cement_Bond_Log Search PubMed.
-
United States Environmental Protection Agency, Geologic CO2 Sequestration Technology and Cost Analysis Technical Support Document, 2008 Jun, p. 816 Search PubMed
.
-
Petrowiki.org, 2021, https://petrowiki.org/images/f/f7/Devol2_1102final_Page_505_Image_0001.png.
-
AFE, Drilling Rig and Tools, PetroWiki, https://petrowiki.org/AFE:_drilling_rig_and_tools Search PubMed.
- D. S. Amorim, O. L. A. Santos, R. C. Azevedo and A. C. Chieregati, A solution for funding the development of technology in oilwell drilling, J. Pet. Explor. Prod. Technol., 2021, 11, 243–252 CrossRef
.
-
Field: ØST FRIGG, Norwegianpetroleum.no, https://www.norskpetroleum.no/en/facts/field/ost-frigg/ Search PubMed.
-
S. T. Hector, Rio Vista Gas Field: History Of Development, http://www.searchanddiscovery.com/abstracts/html/2014/90191pacsec/abstracts/9.1.html Search PubMed.
-
S. Joshi, SPE 83621 Cost/Benefits of Horizontal Wells, 2003, http://www.joshitech.com/images/spe83621.pdf Search PubMed.
-
Costs and Indices for Domestic Oil and Gas Field Equipment and Production Operations, 1992, https://www.arlis.org/docs/vol1/8715527/8715527-1992-1995.pdf Search PubMed.
-
Labor Rates In Mexico - Lower Operating Expenses | Mexican Labor Rates, IVEMSA, https://www.ivemsa.com/manufacturing-in-mexico/mexican-labor-rates/ Search PubMed.
- R. T. Porter, A. Striolo, H. Mahgerefteh and J. Faure Walker, Addressing the risks of induced seismicity in subsurface energy operations, Wiley Interdiscip. Rev.: Energy Environ., 2019, 8(2), e324 Search PubMed
.
-
M. Hopkinson, Net present value and risk modelling for projects, Routledge, 2017 Search PubMed
.
- H. Pilorgé, N. McQueen, D. Maynard, P. Psarras, J. He, T. Rufael and J. Wilcox, Cost analysis of carbon capture and sequestration of process emissions from the US industrial sector, Environ. Sci. Technol., 2020, 54(12), 7524–7532 CrossRef PubMed
.
- R. Span and W. Wagner, A New Equation of State for Carbon Dioxide Covering the Fluid Region from the Triple-Point Temperature to 1100 K at Pressures up to 800 MPa, J. Phys. Chem. Ref. Data, 1996, 25(6), 1509–1596 CrossRef CAS
.
Footnote |
† Electronic supplementary information (ESI) available. See DOI: 10.1039/d1va00036e |
|
This journal is © The Royal Society of Chemistry 2022 |
Click here to see how this site uses Cookies. View our privacy policy here.