DOI:
10.1039/D1VA00024A
(Critical Review)
Environ. Sci.: Adv., 2022,
1, 238-258
Methodologies to characterize, identify and quantify nano- and sub-micron sized plastics in relevant media for human exposure: a critical review
Received
24th October 2021
, Accepted 3rd May 2022
First published on 12th May 2022
Abstract
Micro- and nanoplastics (MNPs) in the environment are an emerging issue of global concern. They accumulate in natural ecosystems, and are ingested by organisms and transferred to humans potentially causing adverse toxicological effects. Knowledge on the magnitude of these effects is limited due to the lack of knowledge on realistic exposures especially for nano- and sub-micron size plastics. Their size and shape have a significant influence on the encountered health effects as well as the presence of additives. Currently, there are no standardized protocols for their reliable characterization (size, shape), identification and quantitation. There is a growing number of reported studies on occurrence of microplastics above 10 μm in size and of limited polymer types (mainly polystyrene, polyethylene terephthalate, polycarbonate and polyethylene). New analytical approaches are needed for a complete and reliable risk assessment of MNPs, especially of sizes below 1 μm, on human health. This review evaluates the progress made concerning the sub-micron (100 nm to 1 μm) and nanometer (<100 nm) size range of MNPs on: (i) human exposure to evaluate the intrinsic hazards, (ii) sampling and sample preparation methods and (iii) methods for characterization (size, shape), identification and quantitation, with a focus on relevant media for human exposure. Methods that could be used for the extraction of submicron and nanoplastics from relevant matrices are recommended. Novel methods (e.g. Raman imaging and single-particle inductively coupled plasma-mass spectrometry) and new combinations of analytical methods (e.g. atomic force microscopy coupled to infrared/raman spectroscopy, field-flow fractionation-multiangle light scattering offline coupled to pyrolysis-gas chromatography-mass spectrometry) are proposed and discussed.
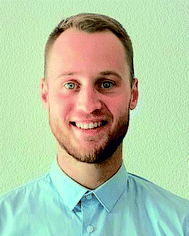 Carlo Roberto de Bruin | MSc Carlo Roberto de Bruin (corresponding author) obtained his master degree (2021) in Analytical Chemistry at the University of Amsterdam. His research was divided over two projects. He worked on a literature review on the characterization of sub-micron and nanoplastics in complex media (e.g. food, air etc.) relevant for human exposure. Afterwards, he joined Genmab BV working on method development for the characterization of released N-glycans from mAbs by high-resolution mass spectrometry. Currently he is pursuing a PhD studying lipids and phytochemicals with cyclic ion mobility mass spectrometry in complex food matrices at the University of Wageningen. |
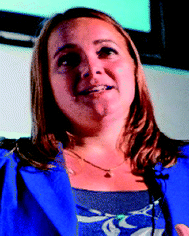 Eva de Rijke | Dr Eva de Rijke works as a labmanager in the soil and environmental chemistry laboratories of the Institute of Biodiversity and Ecosystem Dynamics (IBED) of the University of Amsterdam. She is an analytical chemist who did her PhD at the Vrije Universiteit Amsterdam. After that she worked over 10 years as a scientist/project leader in both government and industrial R&D in the area of food quality and safety involved in research and management of (european) consortia, before returning to environmental analytical chemistry research at the University of Amsterdam. She has broad (hands-on) knowledge of a wide range of analytical techniques to determine e.g. taste/odour compounds, environmental contaminants, additives and (veterinary) drugs. She was board member of the NVMS between 2012 and 2019 and is now board member of the Benelux Association of Stable Isotope Scientists (BASIS). |
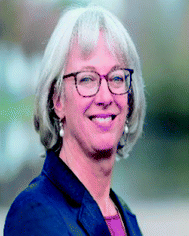 Annemarie P. van Wezel | Prof. Dr Annemarie van Wezel (1968, MSc Biology UU, PhD environmental chemistry and toxicology UU) has long experience as scientist in water quality, risk assessment and mitigation, environmental toxicology and chemistry, and environmental policy evaluation. She was granted as applicant and co-applicant many projects in the field of chemicals of emerging concern and water quality, examples are the European projects FP7 Solutions, ITN ECORISK2050, ITN PERFORCE3, and Dutch NWO funded projects such as Shale gas & water, TRAMP (Technologies for Risk Assessment for Microplastics), EMERCHE (Effect-directed Monitoring tools to assess Ecological and human health Risks of CHemicals of Emerging concern in the water cycle), RUST (Re-USe of Treated effluent for agriculture), PsychoPharmac'eau (Psychopharmaceuticals Prevention & Pilots to Reduce Effects in the water cycle), NWO Large Scientific Infrastructure; Authoritative and Rapid Identification System for Essential biodiversity information (ARISE) and NWO Perspective AQUACONNECT. She is interested in the science-to-policy interface, in scientific outreach and has ample experience in media appearances. She is a member of the Dutch Health Council and the Dutch Board on authorization of plant protection products and biocides CTGB. She holds the chair Environmental Ecology and is Scientific Director of IBED (Institute for Biodiversity and Ecosystem Dynamics) at the University of Amsterdam. |
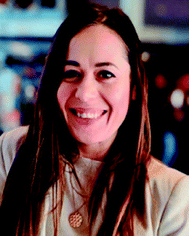 A. Astefanei | Dr Alina Astefanei obtained her PhD from the University of Barcelona on the characterization of carbon nanoparticles in environmental samples. She then joined the HIMS institute at the University of Amsterdam, where she was appointed assistant professor in 2019. Her work is now directed at methodological innovation to solve problems of high impact on society, such as environmental science and art conservation. Alina is developing tools for detailed characterization and quantitation of both large and small molecules, to understand how they interact with each other, and change over time in different conditions. Field-flow fractionation and mass spectrometry (soft and ambient ionization) form the main technology platform. She also coordinates the joint analytical sciences master programme of the Amsterdam Universities. |
Environmental significance
Submicron and nano-plastics in the environment are an emerging issue of global concern. There is a knowledge gap on the magnitude of the posed toxicological effects due to the lack of knowledge on realistic exposures. New analytical approaches and standardized protocols to reliably characterize (size, shape), identify and quantify them are required for a complete and reliable risk assessment on human health. This manuscript reviews the most recent trends and discusses future perspectives of new sample treatment procedures and combinations of newly developed analytical methods and instrumentation for a comprehensive characterization, identification and quantitation of nanoplastics and sub-micron sized plastics in relevant matrices for human health exposure studies.
|
1 Introduction
Plastics are produced in extreme quantities over the entire globe, and their production steeply increased over the past few decades to 368 million tons in 2019. This is expected to have tripled by the year 2050 (Statista 2019).1 Our obsession with plastic can be attributed to its extremely low cost, versatility, inertness, and durability. Currently, plastics are used in all kinds of products such as packaging, clothing, electronics, industrial materials or office supplies. They degrade and transform via mechanical, chemical and biological processes accumulating and persisting in our environment and creating an emerging threat.2,3 Not only are many different polymers used in consumer products, but most also contain co-polymers and additives to tailor the functionality to its intended use. Some additives, such as flame retardants, are toxic and plastics which contain them are certainly not suited to be recycled into things such as children's toys or food packaging. Therefore virgin plastic is often preferred to recycled plastic by manufacturers.4 Of all the plastic ever produced, roughly 12% gets incinerated for energy recovery while 60% is simply disposed of and allowed to accumulate in landfills and the natural environment.5 Plastic debris fractionates into increasingly smaller particles, micro- and nanoplastics (MNPs).6 MNPs have the tendency to accumulate in different matrices like soil,7 freshwater,8 sediment,9 fish tissue10 and air.11 Nanoplastics (NPs) are more reactive and potentially more harmful to humans and ecosystem.12 There are different definitions related to the size range of NPs (Allan et al. 2021), here we use the size definition by Hartmann et al. (2019) with for nanoplastics a size of 1 to <100 nm and for submicron-plastics a size of 100 to <1000 nm. Nanoplastics are polydisperse in physical properties and heterogeneous in composition as their occurrence and production are highly dependent on the degradation of microplastics. They present colloidal behavior which can induce aggregation, depending on the physical and chemical conditions of the medium such as the ionic strength, pH, temperature and UV light. It is believed that microplastics (MPs) can be formed in the environment by four main routes: photodegradation, thermooxidative degradation, hydrolytic degradation and biodegradation by microorganisms.2 For example, photodegradation of larger plastic debris into MPs occurs by exposure to sunlight.13 This triggers a free radical mechanism which is auto accelerated and decreases the average molecular weight of the polymer structure over time. After extensive degradation of the polymer, it becomes brittle enough to disintegrate into MPs and further on to NPs.13,14
Knowledge on exposure levels, i.e. amounts of NPs present in air and media that are ingested via food and water, is still limited due to the lack of dedicated and standardized analytical methodologies. This complicates human risk assessment.
For humans, the major exposure routes are expected to be inhalation (e.g. indoor/outdoor dust, atmospheric fallout) and ingestion (food and beverages). The aim of this review is to give an overview of potential human health effects for sub-micron and nanoplastics, their occurrence in exposure media and a critical discussion on the advantages and limitations of reported sampling, sample treatment, characterization, identification and quantification methods of MNPs in relevant media. Recommendations on future developments of analytical methodologies and new combinations of analytical techniques that are required to make a step forward and cover the current knowledge gaps are being made.
2 Human exposure and health effects
2.1 Exposure routes
Humans can be exposed to MNPs via multiple routes; inhalation, ingestion and dermal contact.15,16 Based on food consumption, a daily intake of 107–142 microplastic particles per person was estimated.17 Fiber exposure during a meal through dust fallout was estimated to range from 38 to 187 particles per day.18
Microplastics have been found in multiple food samples, e.g. in table salt (PE, PP within 171–515 μm),19,20 beer, tap water (>100 μm fibers, no identification).21,22 PS particles in the nanometer range (122–295 nm) were recently characterized in spiked fish samples from a local supermarket in Beijing and were quantified at concentrations ranging 0.068–0.146 mg g−1,23 mainly accumulated in the gills, liver and guts of fish, which are not usually consumed by humans. On the other hand, mussels are consumed as a whole and MNPs accumulate in mussels as well.24 These findings are raising concerns on the bioaccumulation of MNPs in the food chain. Contaminated food and drinking water is one of the greatest concerns in the public media.25,26 It has been reported that exposure routes from packaging could lead to contamination of food as well.27,28
The air contamination with MNPs has multiple sources, such as fibers from clothes and abrasion of materials (e.g. plastic sheets and tires) by wind. The particles are easily transported by the wind and are very persistent. Chen et al. found that airborne microplastics are mainly from synthetic textiles and the dominant shape in the atmosphere are fibers. Fibers larger than 250 μm have been observed in human lungs and may cause chronic and acute inflammation.29 Multiple studies estimated the inhalation of microplastic particles per day. For instance, Prata reviewed the consequences of the inhalation of airborne microplastics and estimated that 26–130 particles could be inhaled per day for each individual.30 This estimation was based on measured particles in the studies from Dris et al.11,31 and the human tidal volume 6 L per min estimated by Guyton and Hall.32 However, Vianello et al. estimated a much higher value of 272 inhaled particles per day for each individual.33 Within all the estimations made in the mentioned studies, variating types of microplastics were identified such as PS, PP, PE, PET, polyester, nylon etc.
Unfortunately, such estimations were not yet made for sub-micron or nanosized plastics and they are required for the risk assessment of NP exposure through air. The results are highly dependent of sampling and sample treatment procedures and this information should be taken into account when discussing this type of information.
Microplastic exposure through dermal contact is less plausible due to the particle size, as it is not likely that they are able to cross the dermal barrier. In contrast, nanoplastics could potentially transverse the dermal barrier, although this was not proven yet.34 However, due to the lack of knowledge on the properties and toxicity of these particles, this possibility should not be underestimated. Cosmetics containing nanoplastics,35,36 dust particles in the air or polluted water may be potential exposure routes for nanoplastics across the dermal barrier. In addition, it has been shown that nanoplastics are capable of penetrating cell membranes,37 which can cause changes of behavior from fish shown by a study of Mattsson et al.38
Forte et al. studied polystyrene (PS) nanoparticles in adenocarcinoma gastric cells (AGS), and reported that smaller sized nanoplastics (44 nm) accumulated faster and more efficiently in the cytoplasm of AGS compared to larger ones (100 nm).39 This indicates that the size determines the concentration in certain body tissues, and therefore, the smallest nanoplastics might cause the most damage to human health.
In summary, for all exposure routes there is limited knowledge on the exposure levels of sub-micron and nanosized plastics.
2.2 Health effects
The exposure to sub-micron and nanosized plastics can lead to potential adverse health effects within humans.36 Effects such as oxidative stress, chronic inflammation, cytotoxicity, endocrine disruption, immune disruption and neurotoxicity40–44 have been reported. These effects may be more severe for nanoplastics, due to faster accumulation in the cytoplasm, more efficient translocation and agglomeration of particles.45
Besides the potential health effects of MNP particles, the release of additives and associated chemicals/contaminants like POPs adsorbed to MNPs may also enhance their toxicity and can cause more significant threats to organisms than MNPs themselves.46 Additives may leach from the plastic into the surrounding environment and enter the human body, contributing to potential health effects.40,47 Leaching will primarily occur at the surface of the plastic particles into the body fluids or tissue. For example, plastic additives like phthalates, brominated flame retardants (BFRs) and bisphenol A (BPA) are most abundant and can be a concern to human health.48 These additives can cause severe health effects such as endocrine disruption,49 neurobehavioral effects50 and carcinogenesis and mutagenesis.51 The studies mentioned above confirm the need for further research on the physical and chemical weathering that can cause breakdown of MNPs and on the interactions of digestive fluid and lipids with plastic matrices within organisms. This is needed for an accurate determination of leaching chemicals and their risk assessment.
Besides the potential hazards of additives from plastics, other compounds that may adsorb to or desorb from plastics may also cause potential health effects. The hydrophobicity of plastic in combination with the high surface area of a micro- and especially nanoparticle, makes them great adsorbents for compounds such as POPs including polychlorinated biphenyls (PCBs), polycyclic aromatic hydrocarbons (PAHs) or heavy metals.52 Similarly as for MNPs, these kind of compounds, can also induce carcinogenesis and mutagenesis,53 and therefore, the combination of these compounds may enhance the toxicity of the whole complex. In this context, Liu et al. studied the adsorption of PAHs on 70 nm PS spheres and concluded a higher adsorption of PAHs to nanoplastics when compared to microplastics (>1000 nm).54 This can be explained by the higher surface to volume ratio of nanoplastics. This finding is relevant because the abundance/amount of nanoplastics will increase over time due to the continuous degradation of (micro)plastics, leading to nanoplastics which could potentially be more harmful to humans.
Regarding the adsorption of heavy metals to MNPs, Liao and Yang conducted a study on spherical PE, PP, PVC and PS microplastics (150 μm) that serve as vector for chromium (Cr) in an in vitro human digestive model.55 Cr can be released in the gastric and intestines phase, but in accordance with the calculated daily intake of Cox et al., the released amounts would not pose any hazards for human health.17 However, for nanoplastics this could be different due to a probable higher adsorption of metals per volume unit, due to the larger surface area. First studies are conducted already on the potential interactions between NPs and metals (e.g. PS and Ag), which showed an increase of the harmful cellular effects.56 This can be of concern when/if metals are added to the plastic during the production process. Furthermore, the adsorption ability of different pollutants is also dependent on several factors such as salinity, temperature, pH, dissolved organic matter and the physical–chemical properties and aging of MNPs.57
2.3 Toxicity studies
The toxicity and potential hazardous properties of NPs are assessed by toxicity studies. Nowadays, animal or in vitro studies are used to provide knowledge on the health effects and toxicity of MNPs.58 For example, Sökmen et al. investigated the exposure of PS nanospheres (20 nm) to zebrafish embryos, and it was shown that these particles can reach the brain and bioaccumulate there, leading to oxidative DNA damage in the brain.59 However, using animals for this purpose is complex due to qualitative and quantitative differences. To explore qualitative and quantitative differences and interactions of toxic compounds within organisms, toxicity-based-toxicokinetic/toxicodynamic (TBTK/TD) modelling can be used.60 TBTK/TD modelling is a powerful mechanistic approach clarifying fate and behaviors of specific toxicants, facilitating to translate exposure to time course of toxic effects on related biomarkers, for example the inhibition of cytochrome P450. A TBTK/TD model was used to quantify organ-bioaccumulation and biomarker responses from PS microplastic particles in mice, that generally serve as mammalian terrestrial model organism.60 This model offers a framework for microplastic exposure in mammals and offers an algorithm for the extrapolation from animals to humans for health risk assessment perspective, which also have the potential to be used for nanoplastics. Unfortunately, such a study has not been reported yet.
Currently, the studies published on in vivo nanoplastics exposure are increasing, for example the studies from Auguste et al.,61 Elizalde-Velázquez et al.62 and Wang et al.63 In addition, ex vivo studies are gaining interest as well.64 However, more studies are needed to clarify the mechanisms for bioaccumulation of nanoplastics in mammals.
Animal testing is not promoted due to ethical issues, and therefore in vitro studies that can provide complementary valuable information are used. Instead of TBTK/TD modelling, physiologically-based toxicokinetic (PBTK) modelling can be used, which enables animal-free risk assessment.65 Mammalian cell lines have proven to be excellent models for the determination of cytotoxicity of potential harmful compounds to human health. Gopinath et al. exposed human blood cells to different forms (virgin, isolated and coronated) of PS nanoplastics (100 nm) in concentrations ranging from 10 to 100 μg mL−1.36 Conformational changes in blood protein, cytotoxicity, genotoxicity and hemolysis were observed after different exposure durations (4 h or 24 h). In addition, there was a significant decrease in cell viability and also damage to the DNA structure. The disadvantage of in vitro studies is the lack of insight in the bioaccumulation process of MNPs, because this process may influence the cytotoxicity. To tackle this limitation, a combination of in vivo and in vitro studies would be more appropriate to investigate toxicity and uptake and bioaccumulation processes of MNPs.45 For example, the question still remains if it is possible for microplastics to degrade in the body of a human or animal into nanoplastics during its excretion process. Future toxicity studies should include different types of nano-sized plastics of various shapes and sizes rather than exclusively using commercially available PS nanospheres. In this context, Gray and Weinstein investigated the influence of different sizes and shapes of microplastics (PS, PE, PP) and it turned out that the mortality of shrimps was highest when exposed to fiber shaped PP microplastics instead of spheres and fragments.66 Until now, doses employed for exposure studies62 (50 mg mL−1 to 0.025 μg mL−1, both in vitro and in vivo) seem unrealistic for environmental exposure of NPs with sizes between 20 and 100 nm. In addition, variation in results may be explained by differences in chemical nature of MNPs such as; size, shape, surface chemistry, other physicochemical properties and different exposure routes. The production of commercially available nanoplastics with variating shapes and sizes needs to be expanded to support the development of toxicological and analytical studies. In Fig. 1, an overview is shown which summarizes the progress in risk assessment of MNPs.
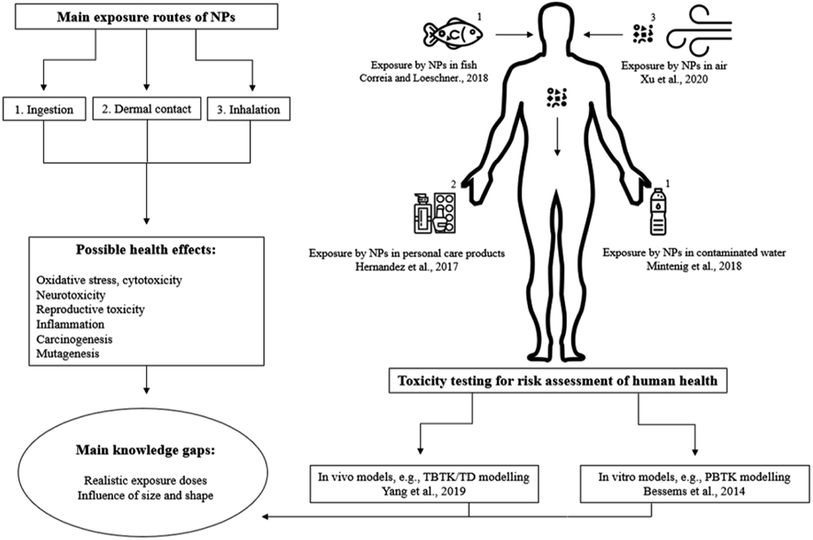 |
| Fig. 1 Overview of the possible exposure routes of humans to MNPs; encountered health effects, and examples of toxicity testing which provide the risk assessment of MNPs to human health. | |
3 Sampling and sample preparation
Contamination is the main issue in any sampling procedure for NP studies, because plastic equipment is widely used and therefore a significant risk for contamination is expected to be widespread. It is thus of great importance to identify potential sources that can contaminate the samples and prevent this as best as possible. Obviously, the role of negative blanks and positive controls is pivotal for data interpretation. Tools and setups should preferably not contain any plastics but rather non-polymer materials to avoid systematic contamination. Samples should be handled under a laminar flow hood and shielded against airborne contamination.
Standardized sampling procedures will make comparisons easier for all kinds of samples containing NPs. Hermsen et al.67 provided a standardized protocol for the detection of ingested microplastics in biota comprising specific requirements for each step in a method, from sampling to detection. This protocol also has potential for the extraction of nanoplastics from biota. It is recommended to follow these requirements before setting up a method, especially for contamination control and the use of positive (spiked samples) and negative controls (blanks). Reports involving extraction of sub-micron and nanoplastics from real samples, such as fish products from markets or environmental air samples, are currently still scarce.68 This section provides an overview of extraction methods that may potentially be used for the sub-micron and nano-sized plastics that are present in matrices relevant for the exposure routes ingestion, inhalation and dermal contact. Preconcentration and filtering methods that are needed for adequate collecting of sub-micron and nano sized plastics are summarized in Section 4.
3.1 Food and beverages
Any type of food and drink samples are relevant for MNP exposure through ingestion. Foods and drinks need to be treated under certain conditions and during sampling contamination with plastics must be avoided. Samples can be taken anywhere from markets, stores and the environment itself. Samples from living organisms have to be frozen at −21 °C according to the International Council for the Exploration of the Sea,69 or could also be preserved in fixatives like formaldehyde or ethanol.67 This is because a living organism will start decomposing after 30 minutes,70 hereafter the sample is not representative anymore.
There are some interesting studies that performed multiple digestion methods for microplastics extraction in fish. Dehaut et al. created a benchmark protocol for the extraction of microplastics (1–1000 μm) in fish species using 10% KOH solution.71 The incubation was performed at 60 °C for 24 hours. The most common polymers found were PE, polyester and rayon. This treatment was not efficient for gill samples. Karami et al.72 reported an incubation time of 72 hours at a lower temperature (40 °C) for the successful treatment of gill samples. It seems that treatments have to be altered for specific parts of the tissue which is not desired for a standardized protocol. Rist et al.73 tested multiple treatments on exposed Daphnia pulex to MNPs such as alkaline digestion with NaOH, 30% H2O2 treatment, acid digestion (nitric acid, HNO3), 25% tetramethyl ammonium hydroxide (TMAH) and an enzymatic digestion with Proteinase K. Although, Daphnia pulex is not indicated as eatable food, the findings in terms of sample treatment were interesting. Consequences of the treatments with NaOH, H2O2 and HNO3 were strong agglomeration of particles and loss of particle fluorescence. The use of TMAH resulted in an incomplete dissolution of the tissues and Proteinase K only gave minor agglomeration of the particles, however, the particle fluorescence signal was completely maintained. The protocol employed for enzymatic treatment (3 hours) was less time consuming compared to alkaline and acid digestion (few days).74 Alkaline digestion with KOH was not tested in this protocol, however, alkaline digestion with NaOH resulted in a significant loss of fluorescence and more agglomeration of the particles. Therefore, it appears that enzymatic digestion is more suitable for the analysis of MNPs with fluorescence detection. When using thermal fragmentation and spectroscopy techniques, digestion with KOH appears to be more suitable.71 Although, enzymatic treatment was not tested in this protocol as it was assumed to be difficult to implement and present digestion efficacy issues.
It is clear that for a reliable an accurate result, the sample treatment used should not alter the MNPs present in the samples. For example, with the use of optical microscopy and dynamic light scattering it has been shown that aggressive methods such as acid, alkaline or H2O2 treatment can cause aggregation of the particles.68 The aggregation could be caused by the significant change of the ionic strength. Furthermore, these treatments could also have negative effects on the fluorescence signal of labeled MNPs (e.g. in toxicology experiments). Enzymatic digestion is milder than acid digestion, alkaline and H2O2 treatment, and therefore, it is likely to be more suitable as treatment protocol before fluorescence or light scattering analysis, as it has been demonstrated to cause no or less aggregation of the particles in food matrices.68 In the study of Correia and Loeschner, the authors have successfully used an enzymatic digestion with Proteinase K for the characterization of spiked PS nanoplastics (600–60 nm) with asymmetrical flow-FFF-multi-angle light scattering (AF4-MALS) (method further elaborated in Section 5.1). The treatment of samples that are indicated as drinkable products such as drinking water is more straightforward as digestion procedures are not required. Murray and Örmeci tested multiple treatments for nanoplastics (<400 nm) from water, where bench-scale filtration, centrifugation, and ballasted flocculation were successfully used.75 All samples relevant for the ingestion route will need preconcentration and filtering steps for the collection of sub-micron and nanosized plastics (Section 4).
The above-mentioned studies that are efficient for the extraction of microplastics from complex samples, can be used as a starting point in future studies on the extraction and analysis of sub-micron and nano-sized plastics.
3.2 Airborne samples
Air samples can be collected through a stand-alone pump,76 vacuum cleaner,77 filters installed indoors or outdoors, or with innovative technologies such as a breathing thermal manikin.33 The limitation of each technique is related to the mesh sizes of filters used, which limits and impacts the collection of plastic samples especially difficult for the nanosized range ones. Therefore, additional preconcentration and filtering steps can be necessary (Section 4).
Compared to other sample types, air samples need to be treated extra carefully as they are mainly consisting of fibers. For this purpose, the filtration system employed needs to be thoroughly cleaned between samples and the used filters need to be exposed to very high temperatures in order to remove the fibers and other contaminants.78 Airborne contamination by synthetic fibers originating from atmospheric fall out, clothing or gear is probably the most difficult to avoid. To tackle this, blank samples and recovery studies using the proposed analytical method should be performed at all times.
There are some interesting studies that performed microplastic extraction from air samples and might be used in the future for sub-micron and nanosized plastics. For instance, the most common treatment reported involves density separation with ZnCl or NaI.76,79 Prata et al.78 used a different approach that involved an initial step to remove the organic matter by using 15% H2O2 during an 8 day treatment prior to filtration over a washed glass fiber filter and transferring to a NaI solution for density separation. Two procedural blanks were added and subjected to same treatment as the samples. The blanks contained 27 fiber particles which were likely released from the cotton lab coat and paper towels. This method delivered 94.4% recovery of PS spiked in common textile fibers and was applied to real indoor and outdoor samples. The method highlights the need for organic matter removal, providing a satisfactory recovery value. Nevertheless, the methods reported is very time consuming as the studies take several days.8–14
The studies mentioned above provide an insight in treatment procedures for air samples containing microplastics, which need to be adjusted for adequate collecting and treatment of sub-micron and nanosized plastics.
3.3 Personal care products
Samples that are representative for the exposure of NPs through dermal contact are mostly personal care products such as facial scrubs. Although there are limited studies on this matrix, in our belief such a matrix is relevant because nanoplastics could potentially transverse the dermal barrier.34 Hernandez et al. reported a study on the extraction and analysis of nanoplastics in facial scrubs.35 In this study, a simple extraction was performed by adding 10 mL of reverse osmosis water, which reduced the viscosity of the samples. This was followed by multiple consecutive filtering steps to isolate the NPs. The analysis was performed by dynamic light scattering. Again, such a study is limited to the mesh size of the filter (100 nm) and therefore nanoparticles around and below 100 nm cannot be isolated. This technique was also used by Gopinath et al., which automatically was restricted by the same size limit.36 Therefore, the isolation of NPs within this matrix also needs improvements in the preconcentration and filtering steps to isolate nanoplastics below 100 nm. Other potential sources of NP exposure by dermal contact could be sand or snow, which are matrices that have shown to contain NPs.80,81 Although it has not been proven that such matrices can transfer NPs across the dermal barrier and they are less likely to cause human exposure compared to personal care products.
4 Preconcentration and filtering
For all the relevant matrices, preconcentration and filtering steps are needed after extraction of sub-micron and nanosized plastics and to improve the limit of detection and limit of quantification (LOD and LOQ) of existing methods. The filtering techniques used within microplastic research are not applicable because of the high mesh size of the filters. Multiple studies showed that collecting nanoparticles can be rather tricky.82 In fact, all type of samples should undergo preconcentration and filtering steps due to the extremely low amounts of sub-micron and nano sized plastic particles. Multiple techniques can be used for this purpose, such as membrane filtration, ultrafiltration, ultracentrifugation, continuous flow centrifugation and cloud point extraction83–86 which can aid in the collection and enrichment of nanoparticles.87,88 Other standard techniques such as freeze-drying and evaporation of the solvent can also be used for this purpose dependent on the type of sample.
However, techniques like membrane filtration and ultrafiltration are limited to the sizes of their inner channels, which still complicates the collection of NPs with sizes below 100 nm. Liu et al., reported the use of surface tension gradients89 but this technique is limited by the amount of sample and it is also not able to distinguish the nanoplastics (<100 nm) from the sub-micron plastics (100–1000 nm). For this purpose, it is more likely that non-destructive separation techniques like FFF (Section 5.1) are more suitable to deliver fractions of particle size distributions (PSD).
5 Characterization and identification of MNPs
In this section the methods reported for the characterization and identification of submicron and nanosized plastics are reviewed and discussed. Fig. 2 shows an overview of the different strategies that can be used for the relevant matrices from sample treatment to analysis. Table 1 includes the relevant reports on the treatment and analysis of the sub-micron and nanosized plastics in such samples. In addition, it also contains reports that could be used in the future.
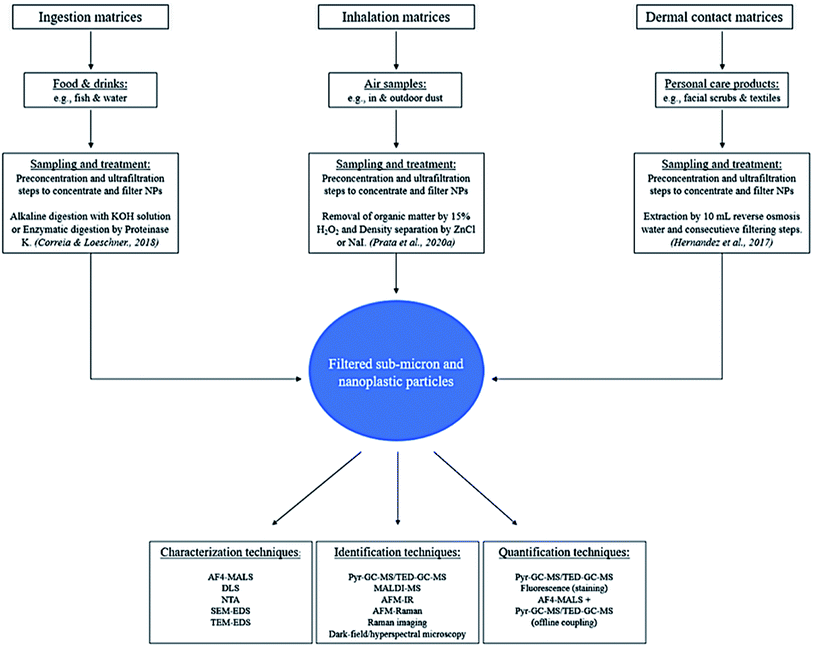 |
| Fig. 2 Overview of the used and possible analytical strategies from sample treatment till analysis for the indicated matrices relevant to the exposure routes (ingestion, inhalation and dermal contact). | |
Table 1 Overview of the recent studies for the characterization, identification and/or quantification of sub-micron and nanosized plastics as commercial standards or in relevant matricesa
Polymer, size range |
Sample |
Sample treatment |
Analysis techniques |
Quantitation (LOD/detected levels) |
Ref. |
PS: polystyrene; PO: polyolefins; PA: polyamide; PET: polyethylene terephthalate; PP: polypropylene; PE: polyethylene; PMMA: poly(methyl methacrylate); P(E-MMA): poly(ethylene-methyl methacrylate copolymer); PBMA: poly(n-butyl methacrylate); MBS: methyl methacrylate-butadiene-styrene copolymer; PVC: polyvinyl chloride; PTFE: polytetrafluoroethylene; TEM: transmission electron microscopy; SEM: scanning electron microscopy; FE-SEM: field emission-SEM; DLS: dynamic light scattering; MALS: multi-angle light scattering; MALDI-TOF: matrix-assisted laser desorption ionization-time of flight; MS: mass spectrometry; AF4: asymmetrical flow-field flow fractionation FFF: field flow fractionation; EDS: energy dispersive X-ray spectroscopy; FTIR: Fourier-transform infrared spectroscopy; ATR-FTIR: attenuated total reflectance-FTIR; XPS: X-ray photoelectron spectroscopy; pyrGC-MS: pyrolysis gas chromatography-mass spectrometry; ICP-MS: inductively coupled plasma-mass spectrometry; TD-PTR-MS: thermal desorption–photon transfer reaction-mass spectrometry; NTA: nanoparticle tracking analysis; SERS: surface-enhanced Raman spectroscopy; LOD: limit of detection.
Methods that have the potential to be used in the future for the indicated relevant matrices.
|
PS, 2 μm to 100 nm |
Blue mussel larvae |
Exposure of PS beads, 2 μm to 100 nm |
Fluorescence |
0.8, 0.3, and 0.5 ng per larvae of the 100 nm beads |
24
|
4.9, 3.4, 3.1 ng per larvae of the 2 μm beads |
PS, PET, 300–500 nm |
Fish |
1% KOH at 50 °C for 36 h |
TEM, DLS, MALDI-TOF MS |
LOD 25 mg L−1 |
23
|
NaCl solution, thermal treatment |
PS, 100 nm |
Spike fish samples |
Acid digestion and enzymatic digestion with proteinase K |
AF4 MALS, DLS, fluorescence |
LOD 52 μg g−1 |
68
|
PS, 60, 200, 600 nm |
Egg shell samples spiked |
Digestion with 0.01 HCl and ultra-centrifugation |
AF4 coupled online with MALS and UV |
— |
90
|
PS, 600, 300 100 nm |
Paint-polishing dust samples |
Removal of organic matter with a H2SO4 : H2O2 (2 : 1, v/v) solution |
Raman imaging, SEM-EDS |
— |
91
|
PE, <100 nm |
Commercial facial scrubs |
10 mL of reverse osmosis water, consecutive filtering |
ATR-FTIR, XPS, SEM, TEM, DLS |
— |
35
|
PS, PMMA, 360, 500 nm |
Air |
30% H2O2 |
SERS (define acronym) |
— |
92
|
PS, 100–600 nm |
Commercial spherical beads |
Dissolved in ultrapure water |
FFF-MALS-UV-online Raman with 2D tweezers |
— |
93
|
PS, 20–200 nm |
Commercial spherical beads |
Dissolved in ultrapure water |
TEM, AF4-MALS-UV |
LOD 15–33 μg mL−1 |
94
|
PP, PS, PVC, 100 μm to 100 nm |
Drink water |
PTFE membrane filtering |
Pyr-GC-MS |
LOD < 50 μg L−1 |
95
|
PS, 420 ± 20 nm |
Drink water |
Au particle labeling |
SEM, ICP-MS |
8.4 × 105 nanoparticles pert L |
96
|
PS, 40–400 nm |
Commercial spherical beads |
Nile red staining |
Fluorescence video microscopy, single particle tracking |
2 × 106 nanoparticles per mL |
97
|
PS, PET, < 200 nm |
Snow |
Melting and filtering with PFTE filer 0.2 μm pore size |
TD-PTR-MS |
PS < 1 ng |
81
|
PET 4.6–23.6 ng mL−1 |
PS, PVC, 1 μm to 200 nm |
Sand |
Sand water extraction, filtering with 0.8 & 0.2 μm filters |
TEM-EDS, DLS, Pyr-GC-MS, ICP-MS |
— |
80
|
PS, PET, <1 μm |
River sediment |
Density separation ZnCl2, filtering < 1 μm |
MALDI-TOF-MS |
PS 8.56 ± 0.04 and PET 28.71 ± 0.20 mg kg−1 |
98
|
PE, 10–398 nm |
Degraded MPs from commercial face scrubs |
Extraction with water and filtering with PVDF filer 0.22 μm |
FTIR/Raman, SEM, DLS, NTA |
1.4 × 1013 part. per g of scrub |
99
|
PS, ∼200 nm |
Microplastic degradation |
Four agitation methods: mixing, shaking, flowing, and standing without agitation |
FE-SEM, NTA |
— |
100
|
PS, 20–200 nm |
PS nanospheres |
Dilution in ionized water and gentle mixing |
AF4-MALS-UV |
50–1000 μg mL−1 |
101
|
PS, 100–1000 nm |
Aqueous media |
Metal particle labeling |
sp-ICP-MS |
4.6 × 108 nanoparticles per L (>269 nm) |
102
|
PS, PMMA, 50–500 nm |
Cucumber plants |
Alkaline digestion coupled with cellulose precipitation and ultrasonic leaching |
Pyr-GC-MS, SEM, ICP-MS |
PS LOD 2.31–4.15 μg g−1 |
103
|
PMMA LOD 3.87–8.20 μg g−1 |
PS, PO, PVC, PA, 58–255 nm |
Tap water |
Filtration with micro/nano-porous membrane, salt removal by 0.1 M HCl and digestion of organic matter by 30% H2O2 |
DLS, TEM-XPS, FTIR, AFM-IR, Pyr-GC-MS |
Tentatively quantified in the range of 1.67–2.08 μg L−1 |
104
|
5.1 Size and shape characterization
MNPs can be very different in physical and chemical properties such as hydrodynamic radius, zeta potential, geometry and surface characteristics. These parameters have an influence on their identification and quantitation, and therefore, detailed characterization is crucial. In this section multiple techniques that are able to characterize the size and shape of MNPs are discussed.
5.1.1 Microscopic techniques.
These techniques provide information on the morphology of a sample including the geometry and surface characteristics. Optical microscopy is a technique used for single particle analysis of microplastic particles, where the stereomicroscope is often reported in literature. However, it was reported that this technique is limited due to the difficulty of distinguishing microplastics from other small organic/inorganic debris particles which may lead to false positives and false negatives.105 In addition, a stereomicroscope is not capable of visualizing nanoplastics due to restricted diffraction limits. On the other hand, microscopy is often used in combination with fluorescence for the tracking and translocation of MNPs. Forte et al. performed an in vitro study where human gastric cells were exposed to unmodified PS nanoparticles.39 In this study, fluorescence microscopy was used to track and localize dyed PS nanoparticles and the observed intensities were compared with the maximal intensity to calculate exact concentrations. Rist et al. performed an in vivo study where mussels were exposed to PS beads of 2 μm and 100 nm and fluorescence was used for the same principle (see Table 1).24 The quantification of MNPs with use of fluorescence is discussed in Section 6.
Besides optical microscopy, electron microscopy (EM) is a very powerful technique for detailed information of MNPs. It can observe very small differences between the wavelengths of high energy electrons which illustrates its resolution, which makes it possible to image nanosized particles.106 EM can be divided into the TEM and SEM techniques. Both techniques have high resolution and are mostly coupled to energy dispersive X-ray spectrometer (EDS) allowing visualization of the sample whilst simultaneously gaining qualitative information on the elemental composition. SEM-EDS is powerful combination for the characterization of MNPs, although there are some limitations. The technique is expensive and very time consuming with many sample preparations steps, hence limiting the number of samples that may be analyzed in a given timeframe. In addition, SEM cannot provide colored images which means that the colors of particles cannot be used as identifiers. EDS can detect trace amounts of specific elements (including Na, Al, Ca etc.), and it may therefore determine the presence of additives by the chemical signature of these elements. The major limitation of EDS spectra is its inability to differentiate between elemental signatures originating from the polymer and elemental signatures originating from additives.107 In the discussed study of Correia and Loeschner, SEM was also used for the conformation of the particle size and morphology of the spherical PS nanoplastics.68 These techniques are very useful to visualize the presence of nanosized particles.
El Hadri et al. studied the degradation of primary plastics (PS, PE) into MNPs where plastics were mechanically degraded using a planetary ball mill.108 In addition, environmentally degraded plastics collected from the beach were also tested. The samples were fully characterized by TEM, DLS and AF4-MALS. It was shown that representative environmental samples can be obtained through mechanical degradation, however this should be tested on multiple and various environmental samples as well. The degradation process of plastics may differ in all kinds of environmental matrices.
In the study reported by Gigault et al. and discussed above, TEM analysis was used to determine the particle size and shape of all the types of nanoplastics PE nanoparticles (<100 nm) and PS nanoparticles (∼500 nm). The shapes observed were very heterogeneous, which encourages to perform studies on particles of different shapes and chemistries, and not exclusively on spherical PS particles as it is currently done in most of reported studies. In the research of Caputo et al., it was also stated that multiple complementary techniques are needed to provide accurate size and shape characterization of MNPs, which is also clear from the comparison of all the studies above. Until now, there is no technique that is capable of the complete characterization of both micro- and nanoplastics.109 Therefore, combinations of the mentioned techniques are needed for the characterization of MNPs and to bridge the gap between sub-micron and nanosized plastics.
5.1.2 Light scattering techniques.
Light scattering is a detection method used in many studies for the characterization of MNPs. For example, dynamic light scattering (DLS) can deliver a broad PSD in the range of 1 nm to 3 μm.110 Despite the broad range of particle sizes that can be measured, a mixture of particle sizes may cause problems, as the technique can only measure average hydrodynamic sizes. As a consequence, the measured radii can be skewed towards higher sizes. This is because larger particles will scatter with more intensity than smaller ones, and therefore, the signals of large particles will hinder the signals of the small particles which will be overlooked. Another problem might be caused by contamination of dust fibers or formed aggregates from the sample matrix. This could be a difficult issue for the relevant food, beverages, inhaled particles and personal care products, and therefore, strict measures should be taken for sample preparation when using this detection technique (Section 3). Another approach involves the use of (static) light scattering is multi-angle light scattering (MALS). This technique measures the scattered light from the sample by different angles and can determine the molecular weight and the size distribution (radii of gyration or root mean square radii) of molecules in solution. MALS is commonly used as online detector for size-based separation techniques such as size exclusion chromatography (SEC) or asymmetrical flow-field flow fractionation (AF4). The implementation of AF4-MALS for nanoplastics research is discussed in the next section.
Nanoparticle tracking analysis (NTA) is a light scattering technique complementary to DLS. Both techniques calculate the hydrodynamic size of particles based on the measured Brownian motion. NTA uses a microscope and a high-sensitivity video-camera which makes it possible to visualize (video image) and record every particle. Therefore, it can determine the hydrodynamic size of each individual particle instead of average size data as generated by DLS.111 On the other hand, very polydisperse particles (10 nm to 1 mm) or a very narrow size range (1–10 nm) cannot be measured with suitable accuracy, which makes NTA more limited on size range. However, this limitation can be overcome with the use of filtering, to narrow down the PSD. Hou et al. compared both techniques, where it turned out that DLS was more suitable to study sub-micron particles (>500–3000 nm), while NTA was more accurate in detecting small particles (>1000 nm).112 Therefore, it may be hypothesized that DLS is more suitable for micron and high-submicron plastics and NTA for nanoplastics, although the mentioned study did not focus on plastics, but on cerium oxide nanoparticles. Lambert and Wagner successfully characterized PS, PLA, PP, PE and PET in the range of 30–2000 nm in two studies by using NTA.113,114 It was shown that NTA is capable to quantitate MNPs with commonly used models due to its software. These models and their applications are further described in Bayat et al. 2015,115 Weipeng et al. 2015,116 and Yang et al. 2012.117
5.1.3 Field-flow fractionation (FFF).
FFF is one of the emerging techniques for the separation and size characterization of nanoplastics. The power of FFF is the broad range of particles that can be covered (1–1000 nm) and because it involves minimal to no shear stress and it is non-destructive.118 Because of the minimal to no shear stress involved, the agglomeration behavior of nanoparticles can be studied using this technique.119 The most common variant is asymmetrical flow-FFF (AF4), which is typically coupled to multiple detectors like UV-vis, refractive index, fluorescence, MALS and DLS.120 These detection techniques in combination with AF4 provide information on concentration, number of particles, particle size distributions and molar masses for the characterization of MNPs.
Monikh et al. reported the use of AF4-MALS to successfully fractionate and characterize PS nanoparticles (60, 200, 300, 600 nm) spiked in eggshells at 100 mg L−1 (see Table 1).90 The developed method had a sufficient recovery (>60%) for nanoplastics and could be able to deliver suitable fractions for further identification. However, the results might be different for various types and non-spherical nanoplastics that are weathered in the environment. Correia and Loeschner used AF4-MALS for the analysis of fish tissue samples which were spiked with 100 nm PS particles (at a final concentration of 5.2 μg mL−1) (see Table 1).68 As control, the authors have analyzed non-spiked fish samples. The authors reported the overlayed fractograms obtained by analyzing dye red aqueous fluorescent spherical polystyrene nanoparticles (FIPSNP) in ultrapure water and fish. It was observed that the particles extracted from the fish sample show minor deviation with the peak obtained from the PS standards. The results show the capability of AF4 to separate PS nanoparticles from such a complex matrix. Besides the study involving PS, the authors reported that after optimizing the carrier liquid composition for the AF4 experiments, it was also possible to analyze PE nanoparticles. For the PS nanoparticles, 0.47 mM NaHCO3 (pH 7.7–7.9) was used as the carrier liquid, while FL-70 concentrate was used for PE nanoparticles. However, it was not possible to detect the PE nanoparticles when spiked (10 μg μL−1) to fish samples. The authors attribute this to an elevated light scattering background signal from the organic fish residues in the AF4 running conditions. This could mean that a method developed for a certain type of polymer based nanoplastic may not be applicable to other types and this should be systematically investigated. This can make it complicated to standardize these protocols for multiple types of plastics, unless suitable studies are performed with a wide variety of MNPs of different chemistries. This technique is less likely to be useful when microplastics of sizes above 1 μm are present in the sample as the elution mode will be changed from normal to steric and the separation is jeopardized. In this case, the large particles (of micrometer range) undergo stronger forces from the laminar flow87 and will elute faster than the smaller particles. To prevent this, a filtration step at the inversion point is needed to exclude the larger particles which can be studied by complementary techniques. Methods such as ultrafiltration, ultracentrifugation/centrifugation, can be used.87 For example, Correia and Loeschner used centrifugation before AF4-MALS analysis of nanoplastics in fish.68
Gigault et al. reported the use of AF4-MALS for the characterization of nanoplastics (PS particles, 1 nm to 800 nm) in fish samples.121 It was found that the selectivity increased significantly when the size range was divided in subpopulations. Therefore, the elution profile was tuned into four different subfractions (1–100 nm, 100–200 nm, 200–450 nm, 450–800 nm). Additionally, in these subfraction methods, it was also found that constant cross-flow rates (0.1 and 0.3 mL min−1) enhanced the fractionation power compared to a programmed cross flow rate. The developed method and additional four subfraction methods combined, may be used to study all the submicron populations in fish samples. This study demonstrates the advantages of AF4 coupled to MALS, and the developed methods were also used in a more recent follow up study where the degradation of microplastics to nanoplastics was studied.108
5.2 Chemical identification
5.2.1 Spectroscopic techniques.
FTIR/μ-FTIR, Raman/μ-Raman spectroscopy are useful techniques for the identification of microplastics. However, the spatial resolution of FTIR is not sufficient to identify particles below 50 μm (ref. 122) and difficulties may arise from environmental matrix effects, unless proper sample preparation is used.123,124 Liu et al. reported the use of μ-FTIR to reveal the presence of many kinds of microplastics above 10 μm in air samples.125 Unfortunately, even when combined with microscopic techniques, a spatial resolution below 10 μm cannot be reached.126,127 Therefore, the identification of nanoplastics by FTIR is not currently possible. Compared to FTIR, Raman has a better spatial resolution due to the shorter laser wavelengths that can be utilized, therefore particles down to 10 μm can be analyzed. Additionally, in the case of μ-Raman particles down to 1 μm can be analyzed.124 In addition, Raman measurements have less interference from water and are not dependent on sample thickness.128 On the other hand, sample clean-up is essential for Raman measurements to increase the signal to noise ratio and eliminate potential fluorescence interferences from sample tissue or other compounds in the sample. UV degradation can also alter the Raman spectra, for example the intensity loss of the specific C–Cl bond in poly vinyl chloride (PVC).129 Alternatively, μ-Raman can be coupled to an Atomic Force Microscopy Based Tip-Enhanced Raman Spectroscopy (AFM-TERS) system which can deliver a spatial resolution of 10 nm.130 This may have potential for the identification of nanoplastics, but has not been yet reported. Recently, Sobhani et al.91 successfully analyzed NPs down to 100 nm by Raman imaging (see Table 1), where imaging particles can be visualized and identified. The produced method was also tested on real paint-polishing dust samples. These results are encouraging, but there are several limitations. The main limitation arises when the nanoplastic size is smaller than that of the laser spot. From the Raman image, the size of the imaged nanoplastic is actually determined and limited by the collected Raman signal, the stage-stepping resolution/pixel size and the laser spot size, rather than by the nanoplastic size itself. Therefore, the image resolution needs to be increased which is limited by the diffraction limit of the laser spot. This method was optimized in two recent follow-up studies. Firstly, the resolution was increased by decreasing the mapping pixel size in order to produce a high-resolution image.131 This made it possible to categorize imaged NPs by size groups via their Raman intensity. Secondly, multiple algorithms such as logic-OR, logic-AND and logic-SUBSTRACT were added and combined to prevent false positives and increase the mapping certainty for NP imaging.132 Until now, this approach is the most promising for adequate identification and visualization of sub-micron and nanosized plastics.
The coupling between atomic force microscopy (AFM) and IR spectroscopy allows to characterize nanoparticles and may have potential for nanoplastics.133 However, this coupling is not easy because AFM has a limited sample size which can cause problems for large microplastics that may be present in the sample. Additionally, it can only detect at the surface area of the sample, which means that a sufficient sample preparation is needed to discover smaller sized particles that could be present beyond the surface. AFM and its hyphenation to FTIR or Raman should be further explored for the characterization of MNPs. For instance, AFM-IR has been used for the identification of various types of nanoparticles (polylactic acid, silver & gold) already and has potential for quantification purposes.134,135 Merzel et al. showed the applicability of AFM-IR recently with the characterization of PS nanoplastics (beads of 1000 nm) in mussel siphons.136 However, improvements can be made by optimizing the sample preparation (see Section 3) to investigate nanoplastics beyond the surface.
In terms of imaging, hyperspectral imaging can turn a dark-field optical microscope into a powerful chemical characterization tool.137 This technique has been used for the identification of various nanoparticles around sizes of 5–100 nm. It has the major advantage of imaging particles in unfixed wet samples, which means no sample treatment is needed. However, the major limitation of this technique is the interpretation of complex spectra, therefore, instrumental advances are required such as deconvolution software. Recently, this technique has also been used for successful identification of sub-micron sized plastics (PS, 400–1000 nm) in Caenorhabditis elegans.138
5.2.2 Mass spectrometry (MS).
A different approach with respect to microscopy and spectroscopy are mass spectrometry-based methods. MS is a powerful technique for the identification of MNPs based on their m/z ratio. Techniques such as pyrolysis gas chromatography-MS (Pyr-GC-MS),139,140 thermal gravimetry/desorption gas chromatography MS (TED-GC-MS) or thermal desorption–proton transfer reaction-MS (TD-PTR-MS)81,141,142 and matrix-assisted laser desorption/ionization-MS (MALDI-MS) can be used. These techniques have the advantage that samples can be analyzed in bulk, which is a solution for the lack of sensitivity posed in single-particle analysis. In addition, MS is not limited by the low particle sizes of NPs. However, the main disadvantage of mass spectrometry techniques for NP analysis is the fact that information on particle sizes cannot be obtained. Additionally, the concentration of NPs in environmental samples needs to be sufficient as every MS approach has a certain detection limit (down to ppm or ppt).143 To overcome the limited knowledge on particle sizes, size-based separation techniques can be used prior the MS analysis to obtain a complete picture. In this context, chromatographic techniques such as size exclusion chromatography (SEC)144 and hydrodynamic chromatography (HDC)145 have been reported for the separation of engineered nanoparticles. Hence, they may be also suitable for the separation of nanoplastics. For instance, Pirok et al. combined HDC and SEC in a comprehensive 2D-LC system, where the combined two-dimensional distribution of particle sizes and molecular sizes of PS and polyacrylate particles was obtained successfully.146 Such methods could contribute to the characterization of MNPs and may be combined with MS based approaches as they are non-destructive. The limitation of detection limits is not that easy to overcome, besides making the analysis as sensitive as possible by sufficient sample preparation and preconcentration.
As an example, Lin et al. recently reported a method where thermal fragmentation in combination with MALDI-MS was used (see Table 1).23 Thermal fragmentation decomposes the sample and subsequently the MNPs are identified by fingerprint peaks in both low and high mass regions of the MALDI-MS spectra. Environmental samples are composed of heterogenous MNPs with different molecular weights which causes many variations in peak intensities. Therefore, the low MS responses of the fingerprint peaks need greater intensity considering the low concentration of MNPs in environmental samples. This was done by a thermal fragmentation step which enhanced the intensities of fingerprint peaks and made quantification possible.
The combination of pyrolysis-GC with mass spectrometry is promising and still in a developmental phase.147 Other MS based approaches such as inductively coupled plasma-mass spectrometry operated in single-particle mode (sp-ICP-MS)80,96 are interesting due to their identification and quantification (number of particles) qualities. In the approach of Jiménez-Lamana et al.,96 conjugated nanoplastics with Au-nanoparticles were used, which provides a very sensitive analysis. This technique was also widely discussed in the review of Velimirovic et al.143 The labelling of NPs with metal probes has also been studied by Marigliano et al., again Au-nanoparticles were used and showed most efficiency for NP identification and quantification.102 Imaging with TOF-secondary ion mass spectrometry could also be used for the chemical identification of NPs, as the spatial resolution (>100 nm) is suitable.148 Although it has limitations in long analysis times and only a small area can be covered by each analysis, while sufficient analysis of multiple spots are needed for representative data. This technique has not been used for NPs yet.13 It is clear that MS based approaches are a very powerful tool that can be used for NP analysis, but most likely not as a stand-alone technique. On- and offline combinations remain needed to solve this broad range of research questions along NP analysis.
Additionally, some MS based methods also reported quantification of nanoplastics, which are discussed in Section 6.
6 Quantification of MNPs
The quantification of MNPs in different sample types is not an easy task, as shown by the lack of quantification methods present in literature. The adequate quantitation of particle number, mass, volume and concentration of MNPs is still lacking. Fluorescence is a technique that is capable of the identification and quantification of MNPs based on staining. This technique was mostly reported in toxicology studies where organisms are exposed to labelled PS nanoparticles with a known concentration to investigate the translocation of the particles in organisms and to hypothesize cytotoxicity and health effects, for example in the study of Pitt et al.149 In a recent study of Molenaar et al., fluorescence video microscopy was used in combination with Nile red staining and single particle tracking (SPT).97 The developed method was able to detect 45 nm sized nanoparticles and concentrations of 2 × 106 nanoparticles per mL were reported. Despite of the successful quantification, only spherical PS particles were used and the method is not tested on environmental samples yet, which could cause difficulties due to matrix effects.
Analytical techniques in combination with mass spectrometry (GC-MS) are used for the identification of MNPs, but are also be used for quantification. Dümichen et al. reported multiple studies on the identification and quantification of microplastics with thermal fragmentation.141,142 In 2015, the first paper published using TED-GC-MS demonstrated its capability of identifying and quantifying PE standards, but it has not been tested on real samples.142 In 2019, an optimized method was published with an increased sample throughput and reproducible automated fractioned collection of decomposition products.141 Quantification was achieved by the linear regression curves of PS, PP and PE standards which showed excellent linearities and an internal standard solution was used to compensate for instrumental errors. This method reached lower limits of quantification by a factor 10 (LOQ 0.395 μg) compared to the method from 2015.142 The MS was used in a full scan mode and the method is expected to reach even lower limits in the single ion monitoring (SIM) mode. However, this method is not validated and tested on real samples yet, but it does shows promising results for routine analysis. Another successful quantification method with Pyr-GC-MS was developed by Sullivan et al.95 Micro- and nanoplastics (PP, PS, PVC, 100 μm to 100 nm) were quantified below 50 μg L−1 (LOD) within water samples. Additionally, Materić et al. used TD-PTR-MS for the quantification of PET (<200 nm, 4.6–23.6 ng mL−1) nanoplastics in snow samples81 (see Table 1).
The limitation of a stand-alone pyrolyis or thermal desorption-GC-MS method is the lack of information on particle sizes within environmental samples. This could be tackled, by using AF4-MALS in combination with offline Pyr(or TD)-GC-MS, as it can first separate MNP fractions according to their size and then chemical identification and quantitation can be further performed. This can be a very powerful combination for the detailed characterization, identification and quantification of MNPs, Fig. 3 illustrates this combination of techniques.
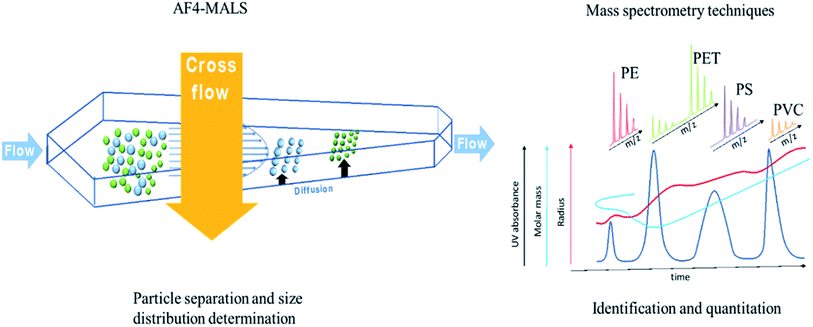 |
| Fig. 3 An example of on-line or off-line combination of size-based fractionation methods (FFF) with MS-based analytical methods (Pyr-GC-MS or TD-PTR-MS) for a complete characterization of sub-micron or nanosized plastic particles. | |
As regards AF4-MALS, Battistini et al. successfully validated an AF4-MALS method for the identification and quantification of nanosized PS particles (20–200 nm) at a LOD of 15–33 μg mL−1.94 The same accounts for the protocol of Bocca et al. that was also able to quantify PS NPs (20–200 nm) at a LOD of 50 μg mL−1.101 However, both methods are limited to nanoplastics that present UV absorbance.
7 Conclusions
It is clear that NPs are a serious environmental issue and a potential risk to human and ecosystem health. Multiple studies investigated the exposure of NPs to animals and negative health effects were found. Additionally, negative health effects were also found within in vitro studies using human cell lines. However, the exposure dose of NPs used in current studies are generally unrealistic as environmental samples are likely to contain low or trace amounts. The observed health effects in toxicity studies are mostly negative. Nevertheless, these studies show the intrinsic hazardous properties of NPs and the need of decreasing plastic debris around the world. The production of commercially available nanoplastic standards with variating shapes needs to be expanded to support the development of analytical studies. Contrary to MPs, NPs are hardly measured in real environmental matrices that are relevant for human health such as drinking water, fish, air and personal care products. More studies are thus urgently required to study sub-micron and nanosized plastics in relevant matrices that are correlated with the main exposure routes: ingestion, inhalation and dermal contact. Recently, some great advances were made in the successful identification of NPs by mostly imaging techniques such as (Raman imaging and dark-field/hyperspectral microscopy). Advances in MS based methods are promising in terms of identification and quantification of NPs, but combinations with other techniques are necessary to characterize them as well (see Future perspectives). More research and improvements are especially necessary in the sampling and sample treatment of NPs in all relevant matrices. This is extremely important to support the progress in analytical techniques for characterization, identification and quantification of sub-micron and nanosized plastics. Further development, harmonization and in time also standardization of quantitation protocols is needed to deliver realistic exposure doses which will lead to accurate risk assessment of NPs on human health.
8 Future perspectives
The characterization and quantitation of sub-micron- and nanosized plastics remains a challenge. Therefore, new combinations of multiple analytical techniques are needed, to make progress and provide more useful data. Combining suitable techniques seems the only way to fully characterize, identify and quantify NPs, as no stand-alone technique is capable of doing all. The latest developments also showed that different combinations can be successful and make significant progress (see Table 2 for an overview). For example, AFM-μ-FTIR or AFM-μ-Raman spectroscopy are combinations of techniques that could be promising for NP identification. Currently, FTIR and Raman spectroscopy can only identify microplastics, because they are limited due to their spatial resolution. Coupling AFM to FTIR or Raman may help to overcome this limitation and identify nanoplastics successfully. This combination is expected to identify nanoplastics down to sizes of 10 nm as the spatial resolution will be lowered significantly. On the other hand, recent advances in Raman imaging and dark-field/hyperspectral microscopy are already very promising as they have shown confident identification of NPs. It is expected to see more studies in the future that utilizes these techniques. For the characterization of nanosized-plastics (10–1000 nm) AF4-MALS has great potential and new studies keep appearing.150 It is capable to deliver the molecular weight, particle numbers, concentration by optional UV detection and particle size distribution. It has the advantage that this technique is non-destructive and it can collect fractions, which can be used for additional analysis with for example Pyr-GC-MS based approaches for identification and quantitation. AF4-MALS can also be combined with spectroscopic and microscopic techniques such as confocal Raman and SEM. This was shown recently by Valsesia et al., where characterization (SEM/AF4-MALS), identification (Raman) and quantification (particle counting software and UV absorbance) was achieved.151 Clustered particles on a chip were used to make the NPs detectable with confocal Raman and this study was successfully applied on C. Robusta. This approach has great potential, but it has to be mentioned that it was still only applied on PSNPs. Recently there was also an extensive combination made of DLS, TEM-XPS, FTIR, AFM-IR and Pyr-GC-MS to characterize and identify NPs (PO, PS, PVC and PA, 58–255 nm) in tap water.104 Even plastic additives such as P(E-MMA), MBS and PBMA were found which is a relevant capability of this method regarding the enhanced toxicity of NP-additive complexes. The indicated example studies provided different combinations of techniques that show the most potential to analyze NPs to date.
Table 2 Overview of the most promising techniques and combinations with their corresponding advantages and disadvantages
Promising techniques |
Advantages |
Disadvantages |
Ref. |
AFM-μ-FTIR and AFM-μ-Raman spectroscopy |
Decreased spatial resolution down to sizes of 10 nm particles |
Sample preparation of complex matrices remains a challenge. Technique is limited by surface detect |
133–135
|
Raman imaging |
Sensitive technique where nanoparticles (>10 nm) can be visualized and identified |
Image resolution needs to be optimized, limited by diffraction limit of the laser spot. Complex data analysis and interpretation |
91, 131 and 132 |
Dark-field/hyperspectral microscopy |
Nanoparticles (>5 nm) can be visualized and identified in unfixed wet samples. No sample preparation needed, which is a major advantage |
Still limited by very complex data analysis and spectra interpretation |
137 and 138 |
AF4-MALS-Pyr-GC-MS or Raman/SEM |
Combination of such techniques can potentially deliver characterization, identification and quantification of nanoplastics (1–1000 nm) |
Very selective for nanoplastics, cannot be used for larger plastic particles anymore (>1000 nm). More time consuming and expensive with additional techniques |
68, 81, 90, 94, 95, 141, 142 and 151 |
sp-ICP-MS |
Sensitive and specific identification, quantification possibilities based on number of particles |
Requires metal labelling and no information on particle sizes |
80, 96, 102 and 103 |
CE-MS |
Characterization of nanoparticle such as gold and fullerene. Potential for nanoplastics |
Not used for nanoplastics yet |
152–155
|
Other MS based approaches such as sp-ICP-MS can also provide very sensitive and specific identification and quantification (number of particles) as well.80,96,102 Capillary electrophoresis (CE) is a separation technique which separates analytes based on their charge to hydrodynamic radius ratio and could be suitable for the analysis of MNPs. CE has been reported for the analysis of different nanoparticles such as gold and fullerene nanoparticles.152–154 In addition, CE-MS was also used for the characterization of nanomaterial in protein corona, where even PS microplastics were found as contamination.155 Unfortunately, there is no CE study available yet that is focused on the analysis of MNPs to the best of our knowledge. There are still options and combinations of techniques that can be explored and may contribute to the analysis of sub-micron- or nanosized plastics. Besides making new combinations, specific sample preparations can simplify the analysis of NPs. For example, Li et al. used a unique extraction protocol with alkaline digestion and cellulose precipitation to characterize, identify and quantify NPs (PS and PMMA, 50–500 nm) in cucumber plants with a combination of Pyr-GC-MS, SEM and ICP-MS.103 Such approaches are desired to move forward in the field. In addition, newly developed or adapted data processing software is also necessary to strengthen the data analysis, which potential was shown in some recent studies. For example, Primpke et al. provided a new software tool (siMPle) for the systematic identification of microplastics within spectroscopic analysis.156 The future developments need to improve the capabilities of the current methodologies for the adequate analysis of these extremely challenging nanoplastic particles. This review focused explicitly on external exposure matrices that can contain NPs. This is because, these sources are more suitable for routine monitoring compared to internal exposure matrices (e.g. NP concentration in blood). However, the described strategies and techniques could also be applied for internal exposure matrices. For example, the research of Gray et al. describes the extraction and analysis of Ag and Au nanoparticles in biological tissues.157 A similar strategy with Proteinase K digestion and sp-ICP-MS analysis was used that also has potential for NPs as indicated in Sections 3.1 and 5.2. Internal exposure matrices will become more interesting within the future, as the concentration of NPs in the environment and exposure to organisms is still likely to increase in the upcoming years.
Conflicts of interest
There are no conflicts to declare.
References
-
Statista, Global plastic production. Plastic – The Facts, 2019 Search PubMed.
- H. K. Webb, J. Arnott, R. J. Crawford and E. P. Ivanova, Plastic degradation and its environmental implications with special reference to poly(ethylene terephthalate), Polymers, 2013, 5(1), 1–18 CrossRef.
-
S. Klein, I. K. Dimzon, J. Eubeler and T. P. Knepper, Analysis, occurrence, and degradation of microplastics in the aqueous environment, Handbook of Environmental Chemistry, 2018, vol. 58, pp. 51–67 Search PubMed.
-
N. Rudolph, R. Kiesel, C. Aumnate, N. Rudolph, R. Kiesel and C. Aumnate, Environmental Analysis of Plastic Waste Handling, in Understanding Plastics Recycling, 2017, ch. 5 Search PubMed.
- R. Geyer, J. R. Jambeck and K. L. Law, Production, use, and fate of all plastics ever made, Sci. Adv., 2017, 3(7), e1700782 CrossRef PubMed.
- J. Gigault, B. Pedrono, B. Maxit and A. Ter Halle, Marine plastic litter: the unanalyzed nano-fraction, Environ. Sci.: Nano, 2016, 3(2), 346–350 RSC.
- S. Zhang, X. Yang, H. Gertsen, P. Peters, T. Salánki and V. Geissen, A simple method for the extraction and identification of light density microplastics from soil, Sci. Total Environ., 2018, 616-617, 1056–1065 CrossRef CAS PubMed.
- Y. Pico, A. Alfarhan and D. Barcelo, Nano- and microplastic analysis: focus on their occurrence in freshwater ecosystems and remediation technologies, TrAC, Trends Anal. Chem., 2019, 113, 409–425 CrossRef CAS.
- P. Yao, B. Zhou, Y. Lu, Y. Yin, Y. Zong and M.-T. Chen,
et al., A review of microplastics in sediments: spatial and temporal occurrences, biological effects, and analytic methods, Quat. Int., 2019, 519, 274–281 CrossRef.
- C. G. Avio, S. Gorbi and F. Regoli, Experimental development of a new protocol for extraction and characterization of microplastics in fish tissues: first observations in commercial species from Adriatic Sea, Mar. Environ. Res., 2015, 111, 18–26 CrossRef CAS PubMed.
- R. Dris, J. Gasperi, M. Saad, C. Mirande and B. Tassin, Synthetic fibers in atmospheric fallout: a source of microplastics in the environment?, Mar. Pollut. Bull., 2016, 104(1–2), 290–293 CrossRef CAS PubMed.
- M. González-Pleiter, M. Tamayo-Belda, G. Pulido-Reyes, G. Amariei, F. Leganés and R. Rosal,
et al., Secondary nanoplastics released from a biodegradable microplastic severely impact freshwater environments, Environ. Sci.: Nano, 2019, 6(5), 1382–1392 RSC.
- I. Jakubowicz, J. Enebro and N. Yarahmadi, Challenges in the search for nanoplastics in the environment—a critical review from the polymer science perspective, Polym. Test., 2021, 93, 106953 CrossRef CAS.
- A. L. Andrady, The plastic in microplastics: a review, Mar. Pollut. Bull., 2017, 119(1), 12–22 CrossRef CAS PubMed.
- J. F. Wambaugh, R. W. Setzer, D. M. Reif, S. Gangwal, J. Mitchell-Blackwood and J. A. Arnot,
et al., High-throughput models for exposure-based chemical prioritization in the ExpoCast project, Environ. Sci. Technol., 2013, 47(15), 8479–8488 CAS.
- A. Dick Vethaak and J. Legler, Microplastics and human health: knowledge gaps should be addressed to ascertain the health risks of microplastics, Science, 2021, 371(6530), 672–674 CrossRef PubMed.
- K. D. Cox, G. A. Covernton, H. L. Davies, J. F. Dower, F. Juanes and S. E. Dudas, Human Consumption of Microplastics, Environ. Sci. Technol., 2019, 53(12), 7068–7074 CrossRef CAS PubMed.
- A. I. Catarino, V. Macchia, W. G. Sanderson, R. C. Thompson and T. B. Henry, Low levels of microplastics (MP) in wild mussels indicate that MP ingestion by humans is minimal compared to exposure via household fibres fallout during a meal, Environ. Pollut., 2018, 237, 675–684 CrossRef CAS PubMed.
- A. Karami, A. Golieskardi, C. Keong Choo, V. Larat, T. S. Galloway and B. Salamatinia, The presence of microplastics in commercial salts from different countries, Sci. Rep., 2017, 7, 46173 CrossRef CAS PubMed.
- Q. Zhang, E. G. Xu, J. Li, Q. Chen, L. Ma and E. Y. Zeng,
et al., A Review of Microplastics in Table Salt, Drinking Water, and Air: Direct Human Exposure, Environ. Sci. Technol., 2020, 54(7), 3740–3751 CrossRef CAS PubMed.
- A. A. Koelmans, N. H. Mohamed Nor, E. Hermsen, M. Kooi, S. M. Mintenig and J. De France, Microplastics in freshwaters and drinking water: critical review and assessment of data quality, Water Res., 2019, 155, 410–422 CrossRef CAS PubMed.
- M. Kosuth, S. A. Mason and E. V. Wattenberg, Anthropogenic contamination of tap water, beer, and sea salt, PLoS One, 2018, 13(4), 1–18 CrossRef PubMed.
- Y. Lin, X. Huang, Q. Liu, Z. Lin and G. Jiang, Thermal fragmentation enhanced identification and quantification of polystyrene micro/nanoplastics in complex media, Talanta, 2020, 208, 120478 CrossRef CAS PubMed.
- S. Rist, A. Baun, R. Almeda and N. B. Hartmann, Ingestion and effects of micro- and nanoplastics in blue mussel (Mytilus edulis) larvae, Mar. Pollut. Bull., 2019, 140, 423–430 CrossRef CAS PubMed.
- S. M. Mintenig, P. S. Bäuerlein, A. A. Koelmans, S. C. Dekker and A. P. Van Wezel, Closing the gap between small and smaller: towards a framework to analyse nano- and microplastics in aqueous environmental samples, Environ. Sci.: Nano, 2018, 5(7), 1640–1649 RSC.
- Y. Li, J. Li, J. Ding, Z. Song, B. Yang and C. Zhang,
et al., Degradation of nano-sized polystyrene plastics by ozonation or chlorination in drinking water disinfection processes, Chem. Eng. J., 2022, 427, 131690 CrossRef CAS.
- P. Zuccarello, M. Ferrante, A. Cristaldi, C. Copat, A. Grasso and D. Sangregorio,
et al., Exposure to microplastics (<10 Mm) associated to plastic bottles mineral water consumption: the first quantitative study, Water Res., 2019, 157, 365–371 CrossRef CAS PubMed.
- D. Schymanski, C. Goldbeck, H. U. Humpf and P. Fürst, Analysis of microplastics in water by micro-Raman spectroscopy: release of plastic particles from different packaging into mineral water, Water Res., 2018, 129, 154–162 CrossRef CAS PubMed.
- G. Chen, Q. Feng and J. Wang, Mini-review of microplastics in the atmosphere and their risks to humans, Sci. Total Environ., 2020, 703, 135504 CrossRef CAS PubMed.
- J. C. Prata, Airborne microplastics: consequences to human health?, Environ. Pollut., 2018, 234, 115–126 CrossRef CAS PubMed.
- R. Dris, J. Gasperi, V. Rocher, M. Saad, N. Renault and B. Tassin, Microplastic contamination in an urban area: a case study in Greater Paris, Environ. Chem., 2015, 12(5), 592–599 CrossRef CAS.
-
E. J. Hall, Guyton and hall textbook of medical physiology, Elsevier, 13th edn, 2011, ch. 7 Search PubMed.
- A. Vianello, R. L. Jensen, L. Liu and J. Vollertsen, Simulating human exposure to indoor airborne microplastics using a Breathing Thermal Manikin, Sci. Rep., 2019, 9, 8670 CrossRef PubMed.
- M. Revel, A. Châtel and C. Mouneyrac, Micro(nano)plastics: a threat to human health?, Curr. Opin. Environ. Sci. Health, 2018, 1, 17–23 CrossRef.
- L. M. Hernandez, N. Yousefi and N. Tufenkji, Are there nanoplastics in your personal care products?, Environ. Sci. Technol. Lett., 2017, 4(7), 280–285 CrossRef CAS.
- P. M. Gopinath, V. Saranya, S. Vijayakumar, M. Mythili Meera, S. Ruprekha and R. Kunal,
et al., Assessment on interactive prospectives of nanoplastics with plasma proteins and the toxicological impacts of virgin, coronated and environmentally released-nanoplastics, Sci. Rep., 2019, 9, 8860 CrossRef PubMed.
- X. Jiang, A. Musyanovych, C. Röcker, K. Landfester, V. Mailänder and G. U. Nienhaus, Specific effects of surface carboxyl groups on anionic polystyrene particles in their interactions with mesenchymal stem cells, Nanoscale, 2011, 3(5), 2028–2035 RSC.
- K. Mattsson, M. T. Ekvall, L. A. Hansson, S. Linse, A. Malmendal and T. Cedervall, Altered behavior, physiology, and metabolism in fish exposed to polystyrene nanoparticles, Environ. Sci. Technol., 2015, 49(1), 553–561 CrossRef CAS PubMed.
- M. Forte, G. Iachetta, M. Tussellino, R. Carotenuto, M. Prisco and M. De Falco,
et al., Polystyrene nanoparticles internalization in human gastric adenocarcinoma cells, Toxicol. In Vitro, 2016, 31, 126–136 CrossRef CAS PubMed.
- J. C. Prata, J. P. da Costa, I. Lopes, A. C. Duarte and T. Rocha-Santos, Environmental exposure to microplastics: an overview on possible human health effects, Sci. Total Environ., 2020, 702, 134455 CrossRef CAS PubMed.
- L. Canesi, C. Ciacci, E. Bergami, M. P. Monopoli, K. A. Dawson and S. Papa,
et al., Evidence for immunomodulation and apoptotic processes induced by cationic polystyrene nanoparticles in the hemocytes of the marine bivalve Mytilus, Mar. Environ. Res., 2015, 111, 34–40 CrossRef CAS PubMed.
- K. Murali, K. Kenesei, Y. Li, K. Demeter, Z. Környei and E. Madarász, Uptake and bio-reactivity of polystyrene nanoparticles is affected by surface modifications, ageing and LPS adsorption: in vitro studies on neural tissue cells, Nanoscale, 2015, 7(9), 4199–4210 RSC.
- G. Barshtein, L. Livshits, L. D. Shvartsman, N. O. Shlomai, S. Yedgar and D. Arbell, Polystyrene Nanoparticles Activate Erythrocyte Aggregation and Adhesion to Endothelial Cells, Cell Biochem. Biophys., 2016, 74(1), 19–27 CrossRef CAS PubMed.
- J. Hwang, D. Choi, S. Han, J. Choi and J. Hong, An assessment of the toxicity of polypropylene microplastics in human derived cells, Sci. Total Environ., 2019, 684, 657–669 CrossRef CAS PubMed.
- X. Meng, J. Zhang, W. Wang, G. Gonzalez-Gil, J. S. Vrouwenvelder and Z. Li, Effects of nano- and microplastics on kidney: physicochemical properties, bioaccumulation, oxidative stress and immunoreaction, Chemosphere, 2022, 288(3), 132631 CrossRef CAS PubMed.
- M. Shen, Y. Zhang, Y. Zhu, B. Song, G. Zeng and D. Hu,
et al., Recent advances in toxicological research of nanoplastics in the environment: a review, Environ. Pollut., 2019, 252, 511–521 CrossRef CAS PubMed.
- M. Cole, P. Lindeque, C. Halsband and T. S. Galloway, Microplastics as contaminants in the marine environment: a review, Mar. Pollut. Bull., 2011, 62(12), 2588–2597 CrossRef CAS PubMed.
- J. N. Hahladakis, C. A. Velis, R. Weber, E. Iacovidou and P. Purnell, An overview of chemical additives present in plastics: migration, release, fate and environmental impact during their use, disposal and recycling, J. Hazard. Mater., 2018, 344, 179–199 CrossRef CAS PubMed.
- A. M. Tsatsakis, I. Katsikantami, O.-I. Kalantzi, Ç. Sevim, K. Tsarouhas and D. Sarigiannis,
et al., Phthalates: Exposure and Health Effects, Encyclopedia of Environmental Health, 2019, 163–173 Search PubMed.
- B. Sun, Y. Hu, H. Cheng and S. Tao, Releases of brominated flame retardants (BFRs) from microplastics in aqueous medium: kinetics and molecular-size dependence of diffusion, Water Res., 2019, 151, 215–225 CrossRef CAS PubMed.
- Y. Ma, H. Liu, J. Wu, L. Yuan, Y. Wang and X. Du,
et al., The adverse health effects of bisphenol A and related toxicity mechanisms, Environ. Res., 2019, 176, 108575 CrossRef CAS PubMed.
- J. Gasperi, S. L. Wright, R. Dris, F. Collard, C. Mandin and M. Guerrouache,
et al., Microplastics in air: are we breathing it in?, Curr. Opin. Environ. Sci. Health, 2018, 1, 1–5 CrossRef.
- K. H. Kim, S. A. Jahan, E. Kabir and R. J. C. Brown, A review of airborne polycyclic aromatic hydrocarbons (PAHs) and their human health effects, Environ. Int., 2013, 60, 71–80 CrossRef CAS PubMed.
- L. Liu, R. Fokkink and A. A. Koelmans, Sorption of polycyclic aromatic hydrocarbons to polystyrene nanoplastic, Environ. Toxicol. Chem., 2016, 35(7), 1650–1655 CrossRef CAS PubMed.
- Y. Liao and J. Yang, Microplastic serves as a potential vector for Cr in an in vitro human digestive model, Sci. Total Environ., 2020, 703, 134805 CrossRef CAS PubMed.
- J. Domenech, C. Cortés, L. Vela, R. Marcos and A. Hernández, Polystyrene nanoplastics as carriers of metals. Interactions of polystyrene nanoparticles with silver nanoparticles and silver nitrate, and their effects on human intestinal caco-2 cells, Biomolecules, 2021, 11(6), 859 CrossRef CAS PubMed.
- F. Yu, C. Yang, Z. Zhu, X. Bai and J. Ma, Adsorption behavior of organic pollutants and metals on micro/nanoplastics in the aquatic environment, Sci. Total Environ., 2019, 694, 133643 CrossRef CAS PubMed.
- C. Q. Y. Yong, S. Valiyaveetill and B. L. Tang, Toxicity of microplastics and nanoplastics in mammalian systems, Int. J. Environ. Res. Public Health, 2020, 17(5), 1509 CrossRef CAS PubMed.
- T. Ö. Sökmen, E. Sulukan, M. Türkoğlu, A. Baran, M. Özkaraca and S. B. Ceyhun, Polystyrene nanoplastics (20 nm) are able to bioaccumulate and cause oxidative DNA damages in the brain tissue of zebrafish embryo (Danio rerio), Neurotoxicology, 2020, 77, 51–59 CrossRef PubMed.
- Y.-F. Yang, C.-Y. Chen, T.-H. Lu and C.-M. Liao, Toxicity-based toxicokinetic/toxicodynamic assessment for bioaccumulation of polystyrene microplastics in mice, J. Hazard. Mater., 2019, 366, 703–713 CrossRef CAS PubMed.
- M. Auguste, A. Lasa, T. Balbi, A. Pallavicini, L. Vezzulli and L. Canesi, Impact of nanoplastics on hemolymph immune parameters and microbiota composition in Mytilus galloprovincialis, Mar. Environ. Res., 2020, 159, 105017 CrossRef CAS PubMed.
- A. Elizalde-Velázquez, J. Crago, X. Zhao, M. J. Green and J. E. Cañas-Carrell, In vivo effects on the immune function of fathead minnow (Pimephales promelas) following ingestion and intraperitoneal injection of polystyrene nanoplastics, Sci. Total Environ., 2020, 735, 139461 CrossRef PubMed.
- Q. Wang, F. Huang, K. Liang, W. Niu, X. Duan and X. Jia,
et al., Polystyrene nanoplastics affect digestive function and growth in juvenile groupers, Sci. Total Environ., 2022, 808, 152098 CrossRef CAS PubMed.
- S. Ballesteros, J. Domenech, I. Barguilla, C. Cortés, R. Marcos and A. Hernández, Genotoxic and immunomodulatory effects in human white blood cells after: ex vivo exposure to polystyrene nanoplastics, Environ. Sci.: Nano, 2020,(7), 3431–3446 RSC.
- J. G. Bessems, G. Loizou, K. Krishnan, H. J. Clewell, C. Bernasconi and F. Bois,
et al., PBTK modelling platforms and parameter estimation tools to enable animal-free risk assessment. Recommendations from a joint EPAA-EURL ECVAM ADME workshop, Regul. Toxicol. Pharmacol., 2014, 68(1), 119–139 CrossRef CAS PubMed.
- A. D. Gray and J. E. Weinstein, Size- and shape-dependent effects of microplastic particles on adult daggerblade grass shrimp (Palaemonetes pugio), Environ. Toxicol. Chem., 2017, 36(11), 3074–3080 CrossRef CAS PubMed.
- E. Hermsen, S. M. Mintenig, E. Besseling and A. A. Koelmans, Quality Criteria for the Analysis of Microplastic in Biota Samples: A Critical Review, Environ. Sci. Technol., 2018, 52(18), 10230–10240 CrossRef CAS PubMed.
- M. Correia and K. Loeschner, Detection of nanoplastics in food by asymmetric flow field-flow fractionation coupled to multi-angle light scattering: possibilities, challenges and analytical limitations, Anal. Bioanal.
Chem., 2018,(410), 5603–5615 CrossRef CAS PubMed.
-
ICES, OSPAR request on development of a common monitoring protocol for plastic particles in fish stomachs and selected shellfish on the basis of existing fish disease surveys, IICES Advice, 2015, Book 1 Search PubMed.
- A. L. Lusher, N. A. Welden, P. Sobral and M. Cole, Sampling, isolating and identifying microplastics ingested by fish and invertebrates, Anal. Methods, 2017,(9), 1346–1360 RSC.
- A. Dehaut, A.-L. Cassone, L. Frère, L. Hermabessiere, C. Himber and E. Rinnert,
et al., Microplastics in seafood: Benchmark protocol for their extraction and characterization, Environ. Pollut., 2016, 215, 223–233 CrossRef CAS PubMed.
- A. Karami, A. Golieskardi, Y. B. Ho, V. Larat and B. Salamatinia, Microplastics in eviscerated flesh and excised organs of dried fish, Sci. Rep., 2017, 7, 5473 CrossRef PubMed.
- S. Rist, A. Baun and N. B. Hartmann, Ingestion of micro- and nanoplastics in daphnia magna – quantification of body burdens and assessment of feeding rates and reproduction, Environ. Pollut., 2017, 228, 398–407 CrossRef CAS PubMed.
- M. Cole, H. Webb, P. K. Lindeque, E. S. Fileman, C. Halsband and T. S. Galloway, Isolation of microplastics in biota-rich seawater samples and marine organisms, Sci. Rep., 2014, 4, 4528 CrossRef PubMed.
- A. Murray and B. Örmeci, Removal effectiveness of nanoplastics (<400 nm) with separation processes used for water and wastewater treatment, Water, 2020, 12(3), 635 CrossRef CAS.
- R. Dris, J. Gasperi, C. Mirande, C. Mandin, M. Guerrouache and V. Langlois,
et al., A first overview of textile fibers, including microplastics, in indoor and outdoor environments, Environ. Pollut., 2017, 221, 453–458 CrossRef CAS PubMed.
- J. Zhang, L. Wang and K. Kannan, Microplastics in house dust from 12 countries and associated human exposure, Environ. Int., 2020, 134, 105314 CrossRef CAS PubMed.
- J. C. Prata, J. L. Castro, J. P. da Costa, A. C. Duarte, M. Cerqueira and T. Rocha-Santos, An easy method for processing and identification of natural and synthetic microfibers and microplastics in indoor and outdoor air, MethodsX, 2020, 7, 100762 CrossRef PubMed.
- S. Allen, D. Allen, V. R. Phoenix, G. Le Roux, P. Durántez Jiménez and A. Simonneau,
et al., Atmospheric transport and deposition of microplastics in a remote mountain catchment, Nat. Geosci., 2019, 12, 339–344 CrossRef CAS.
- M. Davranche, C. Lory, C. L. Juge, F. Blancho, A. Dia and B. Grassl,
et al., Nanoplastics on the coast exposed to the North Atlantic Gyre: evidence and traceability, NanoImpact, 2020, 20, 100262 CrossRef.
- D. Materić, A. Kasper-Giebl, D. Kau, M. Anten, M. Greilinger and E. Ludewig,
et al., Micro-and Nanoplastics in Alpine Snow: A New Method for Chemical Identification and (Semi)Quantification in the Nanogram Range, Environ. Sci. Technol., 2020, 54(4), 2353–2359 CrossRef PubMed.
- C. Kanaoka, Fine particle filtration technology using fiber as dust collection medium, KONA Powder Part. J., 2019, 36, 88–113 CrossRef.
- J. B. Chao, J. F. Liu, S. J. Yu, Y. Di Feng, Z. Q. Tan and R. Liu,
et al., Speciation analysis of silver nanoparticles and silver ions in antibacterial products and environmental waters via cloud point extraction-based separation, Anal. Chem., 2011, 83(17), 6875–6882 CrossRef CAS PubMed.
- L. Hildebrandt, D. M. Mitrano, T. Zimmermann and D. Pröfrock, A Nanoplastic Sampling and Enrichment Approach by Continuous Flow Centrifugation, Front. Environ. Sci. Eng., 2020, 8, 89–101 CrossRef.
- X. Fang, J. Li, X. Li, S. Pan, X. Zhang and X. Sun,
et al., Internal pore decoration with polydopamine nanoparticle on polymeric ultrafiltration membrane for enhanced heavy metal removal, Chem. Eng. J., 2017, 314, 38–49 CrossRef CAS.
- A. Philippe, J. Košík, A. Welle, J. M. Guigner, O. Clemens and G. E. Schaumann, Extraction and characterization methods for titanium dioxide nanoparticles from commercialized sunscreens, Environ. Sci.: Nano, 2018, 5(1), 191–202 RSC.
- C. Schwaferts, R. Niessner, M. Elsner and N. P. Ivleva, Methods for the analysis of submicrometer- and nanoplastic particles in the environment, TrAC, Trends Anal. Chem., 2019, 112, 52–65 CrossRef CAS.
- H. Cai, E. G. Xu, F. Du, R. Li, J. Liu and H. Shi, Analysis of environmental nanoplastics: progress and challenges, Chem. Eng. J., 2021, 410, 128208 CrossRef CAS.
- D. Liu, D. Weng and J. Wang, Collection of nanoparticles at the air–liquid interface by surface tension gradients, Colloids Interface Sci. Commun., 2019, 33, 100205 CrossRef CAS.
- F. Abdolahpur Monikh, N. Grundschober, S. Romeijn, D. Arenas-Lago, M. G. Vijver and W. Jiskoot,
et al., Development of methods for extraction and analytical characterization of carbon-based nanomaterials (nanoplastics and carbon nanotubes) in biological and environmental matrices by asymmetrical flow field-flow fractionation, Environ. Pollut., 2019, 255(2), 113304 CrossRef CAS PubMed.
- Z. Sobhani, X. Zhang, C. Gibson, R. Naidu, M. Megharaj and C. Fang, Identification and visualisation of microplastics/nanoplastics by Raman imaging (i): down to 100 nm, Water Res., 2020, 174, 115658 CrossRef CAS PubMed.
- G. Xu, H. Cheng, R. Jones, Y. Feng, K. Gong and K. Li,
et al., Surface-Enhanced Raman Spectroscopy Facilitates the Detection of Microplastics <1 μm in the Environment, Environ. Sci. Technol., 2020, 54(24), 15594–15603 CrossRef CAS PubMed.
- C. Schwaferts, V. Sogne, R. Welz, F. Meier, T. Klein and R. Niessner,
et al., Nanoplastic Analysis by Online Coupling of Raman Microscopy and Field-Flow Fractionation Enabled by Optical Tweezers, Anal. Chem., 2020, 92(8), 5813–5820 CrossRef CAS PubMed.
- B. Battistini, F. Petrucci and B. Bocca, In-house validation of AF4-MALS-UV for polystyrene nanoplastic analysis, Anal. Bioanal. Chem., 2021, 413, 3027–3039 CrossRef CAS PubMed.
- G. L. Sullivan, J. D. Gallardo, E. W. Jones, P. J. Hollliman, T. M. Watson and S. Sarp, Detection of trace sub-micron (nano) plastics in water samples using pyrolysis-gas chromatography time of flight mass spectrometry (PY-GCToF), Chemosphere, 2020, 249, 126179 CrossRef CAS PubMed.
- J. Jiménez-Lamana, L. Marigliano, J. Allouche, B. Grassl, J. Szpunar and S. Reynaud, A Novel Strategy for the Detection and Quantification of Nanoplastics by Single Particle Inductively Coupled Plasma Mass Spectrometry (ICP-MS), Anal. Chem., 2020, 92(17), 11664–11672 CrossRef PubMed.
- R. Molenaar, S. Chatterjee, B. Kamphuis, I. M. J. Segers-Nolten, M. M. A. E. Claessens and C. Blum, Nanoplastic sizes and numbers: quantification by single particle tracking, Environ. Sci.: Nano, 2021, 8(3), 723–730 RSC.
- P. Wu, Y. Tang, G. Cao, J. Li, S. Wang and X. Chang,
et al., Determination of Environmental Micro(Nano)Plastics by Matrix-Assisted Laser Desorption/Ionization-Time-of-Flight Mass Spectrometry, Anal. Chem., 2020, 92(21), 14346–14356 CrossRef CAS PubMed.
- M. Enfrin, J. Lee, Y. Gibert, F. Basheer, L. Kong and L. F. Dumée, Release of hazardous nanoplastic contaminants due to microplastics fragmentation under shear stress forces, J. Hazard. Mater., 2020, 384, 121393 CrossRef CAS PubMed.
- H. Mekaru, Effect of Agitation Method on the Nanosized Degradation of Polystyrene Microplastics Dispersed in Water, ACS Omega, 2020, 5(7), 3218–3227 CrossRef CAS PubMed.
-
B. Bocca, B. Battistini and F. Petrucci, A protocol for size-based measurements of nanoplastics across
the range 20–200 nm, 2021, vol. 2416, p. 1.
- L. Marigliano, B. Grassl, J. Szpunar, S. Reynaud and J. Jiménez-Lamana, Nanoplastic labelling with metal probes: analytical strategies for their sensitive detection and quantification by icp mass spectrometry, Molecules, 2021, 26(23), 7093 CrossRef CAS PubMed.
- C. Li, Y. Gao, S. He, H. Y. Chi, Z. C. Li and X. X. Zhou,
et al., Quantification of Nanoplastic Uptake in Cucumber Plants by Pyrolysis Gas Chromatography/Mass Spectrometry, Environ. Sci. Technol. Lett., 2021, 8(8), 633–638 CrossRef CAS.
- Y. Li, Z. Wang and B. Guan, Separation and identification of nanoplastics in tap water, Environ. Res., 2022, 204(B), 112134 CrossRef CAS PubMed.
- Y. K. Song, S. H. Hong, M. Jang, G. M. Han, M. Rani and J. Lee,
et al., A comparison of microscopic and spectroscopic identification methods for analysis of microplastics in environmental samples, Mar. Pollut. Bull., 2015, 93(1–2), 202–209 CrossRef CAS PubMed.
-
G. H. Michler and W. Lebek, Electron microscopy of polymers, in Polymer Morphology: Principles, Characterization and Processing, 2016, ch. 3, pp. 37–53 Search PubMed.
- A. B. Silva, A. S. Bastos, C. I. L. Justino, J. P. da Costa, A. C. Duarte and T. A. P. Rocha-Santos, Microplastics in the environment: challenges in analytical chemistry – a review, Anal. Chim. Acta, 2018, 1017, 1–19 CrossRef CAS PubMed.
- H. El Hadri, J. Gigault, B. Maxit, B. Grassl and S. Reynaud, Nanoplastic from mechanically degraded primary and secondary microplastics for environmental assessments, NanoImpact, 2020, 17, 100206 CrossRef.
- F. Caputo, R. Vogel, J. Savage, G. Vella, A. Law and G. Della Camera,
et al., Measuring particle size distribution and mass concentration of nanoplastics and microplastics: addressing some analytical challenges in the sub-micron size range, J. Colloid Interface Sci., 2021, 588, 401–417 CrossRef CAS PubMed.
- R. Finsy, Particle Sizing in the Submicron Range by Dynamic Light Scattering, KONA Powder Part. J., 1993, 11, 17–32 CrossRef.
- W. Fu, J. Min, W. Jiang, Y. Li and W. Zhang, Separation, characterization and identification of microplastics and nanoplastics in the environment, Sci. Total Environ., 2020, 721, 137561 CrossRef CAS PubMed.
- J. Hou, H. Ci, P. Wang, C. Wang, B. Lv and L. Miao,
et al., Nanoparticle tracking analysis versus dynamic light scattering: case study on the effect of Ca2+ and alginate on the aggregation of cerium oxide nanoparticles, J. Hazard. Mater., 2018, 360, 319–328 CrossRef CAS PubMed.
- S. Lambert and M. Wagner, Characterisation of nanoplastics during the degradation of polystyrene, Chemosphere, 2016, 145, 265–268 CrossRef CAS PubMed.
- S. Lambert and M. Wagner, Formation of microscopic particles during the degradation of different polymers, Chemosphere, 2016, 161, 510–517 CrossRef CAS PubMed.
- H. Bayat, M. Rastgo, M. Mansouri Zadeh and H. Vereecken, Particle size distribution models, their characteristics and fitting capability, J. Hydrol., 2015, 529(3), 872–889 CrossRef.
- W. Weipeng, L. Jianli, Z. Bingzi, Z. Jiabao, L. Xiaopeng and Y. Yifan, Critical evaluation of particle size distribution models using soil data obtained with a Laser diffraction method, PLoS One, 2015, 10(4) CrossRef PubMed.
- X. Yang, J. Lee, D. E. Barker, X. Wang and Y. Zhang, Comparison of six particle size distribution models on the goodness-of-fit to particulate matter sampled from animal buildings, J. Air Waste Manage. Assoc., 2012, 62(6), 725–735 CrossRef PubMed.
- K. G. Wahlund, Flow field-flow fractionation: critical overview, J. Chromatogr. A, 2013, 1287, 97–112 CrossRef CAS PubMed.
- L. Chekli, M. Roy, L. D. Tijing, E. Donner, E. Lombi and H. K. Shon, Agglomeration behaviour of titanium dioxide nanoparticles in river waters: a multi-method approach combining light scattering and field-flow fractionation techniques, J. Environ. Manage., 2015, 159, 135–142 CrossRef CAS PubMed.
- Z. Gajdosechova and Z. Mester, Recent trends in analysis of nanoparticles in biological matrices, Anal. Bioanal. Chem., 2019, 411, 4277–4292 CrossRef CAS PubMed.
- J. Gigault, H. El Hadri, S. Reynaud, E. Deniau and B. Grassl, Asymmetrical flow field flow fractionation methods to characterize submicron particles: application to carbon-based aggregates and nanoplastics, Anal. Bioanal. Chem., 2017, 409, 6761–6769 CrossRef CAS PubMed.
- I. Ferreira, C. Venâncio, I. Lopes and M. Oliveira, Nanoplastics and marine organisms: what has been studied?, Environ. Toxicol. Pharmacol., 2019, 67, 1–7 CrossRef CAS PubMed.
-
M. G. J. Löder and G. Gerdts, Methodology used for the detection and identification of microplastics—a critical appraisal, in Marine Anthropogenic Litter, 2015, pp. 201–227 Search PubMed.
- A. Käppler, D. Fischer, S. Oberbeckmann, G. Schernewski, M. Labrenz and K. J. Eichhorn,
et al., Analysis of environmental microplastics by vibrational microspectroscopy: FTIR, Raman or both?, Anal. Bioanal. Chem., 2016, 408, 8377–8391 CrossRef PubMed.
- K. Liu, X. Wang, T. Fang, P. Xu, L. Zhu and D. Li, Source and potential risk assessment of suspended atmospheric microplastics in Shanghai, Sci. Total Environ., 2019, 675, 462–471 CrossRef CAS PubMed.
- S. Huppertsberg and T. P. Knepper, Instrumental analysis of microplastics—benefits and challenges, Anal. Bioanal. Chem., 2018, 410(25), 6343–6352 CrossRef CAS PubMed.
- M. G. J. Löder, M. Kuczera, S. Mintenig, C. Lorenz and G. Gerdts, Focal plane array detector-based micro-Fourier-transform infrared imaging for the analysis of microplastics in environmental samples, Environ. Chem., 2015, 12(5), 563–581 CrossRef.
- A. M. Elert, R. Becker, E. Dümichen, P. Eisentraut, J. Falkenhagen and H. Sturm,
et al., Comparison of different methods for MP detection: what can we learn from them, and why asking the right question before measurements matters?, Environ. Pollut., 2017, 231(part 2), 1256–1264 CrossRef CAS PubMed.
- R. Lenz, K. Enders, C. A. Stedmon, D. M. A. Mackenzie and T. G. Nielsen, A critical assessment of visual identification of marine microplastic using Raman spectroscopy for analysis improvement, Mar. Pollut. Bull., 2015, 100(1), 82–91 CrossRef CAS PubMed.
- N. Kumar, S. Mignuzzi, W. Su and D. Roy, Tip-enhanced Raman spectroscopy: principles and applications, EPJ Quantum Technol., 2015, 2(9), 1–23 Search PubMed.
- C. Fang, Z. Sobhani, X. Zhang, C. T. Gibson, Y. Tang and R. Naidu, Identification and visualisation of microplastics/nanoplastics by Raman imaging (ii): smaller than the diffraction limit of laser?, Water Res., 2020, 183, 116046 CrossRef CAS PubMed.
- C. Fang, Z. Sobhani, X. Zhang, L. McCourt, B. Routley and C. T. Gibson,
et al., Identification and visualisation of microplastics/nanoplastics by Raman imaging (iii): algorithm to cross-check multi-images, Water Res., 2021, 194, 116913 CrossRef CAS PubMed.
- A. Dazzi, C. B. Prater, Q. Hu, D. B. Chase, J. F. Rabolt and C. Marcott, AFM-IR: combining atomic force microscopy and infrared spectroscopy for nanoscale chemical characterization, Appl. Spectrosc., 2012, 66(12), 1365–1384 CrossRef CAS PubMed.
- N. Piergies, A. Dazzi, A. Deniset-Besseau, J. Mathurin, M. Oćwieja and C. Paluszkiewicz,
et al., Nanoscale image of the drug/metal mono-layer interaction: tapping AFM-IR investigations, Nano Res., 2020, 13(4), 1020–1028 CrossRef CAS.
- J. Mathurin, E. Pancani, A. Deniset-Besseau, K. Kjoller, C. B. Prater and R. Gref,
et al., How to unravel the chemical structure and component localization of individual drug-loaded polymeric nanoparticles by using tapping AFM-IR, Analyst, 2018, 143(24), 5940–5949 RSC.
- R. L. Merzel, L. Purser, T. L. Soucy, M. Olszewski, I. Colón-Bernal and M. Duhaime,
et al., Uptake and Retention of Nanoplastics in Quagga Mussels, Global Challenges, 2020, 4(6), 1800104 CrossRef PubMed.
- R. Fakhrullin, L. Nigamatzyanova and G. Fakhrullina, Dark-field/hyperspectral microscopy for detecting nanoscale particles in environmental nanotoxicology research, Sci. Total Environ., 2021, 772, 145478 CrossRef CAS PubMed.
- L. Nigamatzyanova and R. Fakhrullin, Dark-field hyperspectral microscopy for label-free microplastics and nanoplastics detection and identification in vivo: a Caenorhabditis elegans study, Environ. Pollut., 2021, 271, 166337 CrossRef PubMed.
- F. Blancho, M. Davranche, H. E. Hadri, B. Grassl and J. Gigault, Nanoplastics Identification in Complex Environmental Matrices: Strategies for Polystyrene and Polypropylene, Environ. Sci. Technol., 2021, 55(13), 8753–8759 CrossRef CAS PubMed.
- M. Fischer and B. M. Scholz-Böttcher, Simultaneous Trace Identification and Quantification of Common Types of Microplastics in Environmental Samples by Pyrolysis-Gas Chromatography-Mass Spectrometry, Environ. Sci. Technol., 2017, 5052–5060 CrossRef CAS PubMed.
- E. Dümichen, P. Eisentraut, M. Celina and U. Braun, Automated thermal extraction–desorption gas chromatography mass spectrometry: a multifunctional tool for comprehensive characterization of polymers and their degradation products, J. Chromatogr. A, 2019, 133–142 CrossRef PubMed.
- E. Dümichen, A. K. Barthel, U. Braun, C. G. Bannick, K. Brand and M. Jekel,
et al., Analysis of polyethylene microplastics in environmental samples, using a thermal decomposition method, Water Res., 2015, 451–457 CrossRef PubMed.
- M. Velimirovic, K. Tirez, S. Voorspoels and F. Vanhaecke, Recent developments in mass spectrometry for the characterization of micro- and nanoscale plastic debris in the environment, Anal. Bioanal. Chem., 2021, 7–15 CrossRef CAS PubMed.
- X. X. Zhou, R. Liu and J. F. Liu, Rapid chromatographic separation of dissoluble Ag(I) and silver-containing nanoparticles of 1–100 nanometer in antibacterial products and environmental waters, Environ. Sci. Technol., 2014, 14516–14524 CrossRef CAS PubMed.
- E. P. Gray, T. A. Bruton, C. P. Higgins, R. U. Halden, P. Westerhoff and J. F. Ranville, Analysis of gold nanoparticle mixtures: a comparison of hydrodynamic chromatography (HDC) and asymmetrical flow field-flow fractionation (AF4) coupled to ICP-MS, J. Anal. At. Spectrom., 2012, 1532–1539 RSC.
- B. W. J. Pirok, N. Abdulhussain, T. Aalbers, B. Wouters, R. A. H. Peters and P. J. Schoenmakers, Nanoparticle Analysis by Online Comprehensive Two-Dimensional Liquid Chromatography combining Hydrodynamic Chromatography and Size-Exclusion Chromatography with Intermediate Sample Transformation, Anal. Chem., 2017, 9167–9174 CrossRef CAS PubMed.
- L. Hermabessiere, C. Himber, B. Boricaud, M. Kazour, R. Amara and A. L. Cassone,
et al., Optimization, performance, and application of a pyrolysis-GC/MS method for the identification of microplastics, Anal. Bioanal. Chem., 2018, 6663–6676 CrossRef CAS PubMed.
- J. Kokesch-Himmelreich, B. Woltmann, B. Torger, M. Rohnke, S. Arnhold and U. Hempel,
et al., Detection of organic nanoparticles in human bone marrow-derived stromal cells using ToF-SIMS and PCA, Anal. Bioanal. Chem., 2015, 407(16), 4555–4565 CrossRef CAS PubMed.
- J. A. Pitt, J. S. Kozal, N. Jayasundara, A. Massarsky, R. Trevisan and N. Geitner,
et al., Uptake, tissue distribution, and toxicity of polystyrene nanoparticles in developing zebrafish (Danio rerio), Aquat. Toxicol., 2018, 185–194 CrossRef CAS PubMed.
- M. Mowla, S. Shakiba and S. M. Louie, Selective quantification of nanoplastics in environmental matrices by asymmetric flow field-flow fractionation with total organic carbon detection, Chem. Commun., 2021, 57(96), 12940–12943 RSC.
- A. Valsesia, J. Parot, J. Ponti, D. Mehn, R. Marino and D. Melillo,
et al., Detection, counting and characterization of nanoplastics in marine bioindicators: a proof of principle study, Microplastics and Nanoplastics, 2021,(1), 5 CrossRef.
- H. Qu, S. W. Linder and T. K. Mudalige, Surface coating and matrix effect on the electrophoretic mobility of gold nanoparticles: a capillary electrophoresis-inductively coupled plasma mass spectrometry study, Anal. Bioanal. Chem., 2017, 979–988 CrossRef CAS PubMed.
- A. Astefanei, O. Núñez and M. T. Galceran, Characterisation and determination of fullerenes: a critical review, Anal. Chim. Acta, 2015, 1–21 CrossRef CAS PubMed.
- A. Astefanei, O. Núñez, M. T. Galceran, W. T. Kok and P. J. Schoenmakers, Aggregation behavior of fullerenes in aqueous solutions: a capillary electrophoresis and asymmetric flow field-flow fractionation study, Anal. Bioanal. Chem., 2015, 8035–8045 CrossRef CAS PubMed.
- K. Faserl, A. J. Chetwynd, I. Lynch, J. A. Thorn and H. H. Lindner, Corona isolation method matters: capillary electrophoresis mass spectrometry based comparison of protein corona compositions following on-particle versus in-solution or in-gel digestion, Nanomaterials, 2019, 898 CrossRef CAS PubMed.
- S. Primpke, R. K. Cross, S. M. Mintenig, M. Simon, A. Vianello and G. Gerdts,
et al., Toward the Systematic Identification of Microplastics in the Environment: Evaluation of a New Independent Software Tool (siMPle) for Spectroscopic Analysis, Appl. Spectrosc., 2020, 74(9), 1127–1138 CrossRef CAS PubMed.
- E. P. Gray, J. G. Coleman, A. J. Bednar, A. J. Kennedy, J. F. Ranville and C. P. Higgins, Extraction and analysis of silver and gold nanoparticles from biological tissues using single particle inductively coupled plasma mass spectrometry, Environ. Sci. Technol., 2013, 47(24), 14315–14323 CrossRef CAS PubMed.
|
This journal is © The Royal Society of Chemistry 2022 |
Click here to see how this site uses Cookies. View our privacy policy here.