DOI:
10.1039/D1TC04302A
(Review Article)
J. Mater. Chem. C, 2022,
10, 2390-2399
Methylamine gas healing of perovskite films: a short review and perspective
Received
9th September 2021
, Accepted 4th November 2021
First published on 5th November 2021
Abstract
The first challenge in the commercialization of perovskite solar cells (PSCs) is how to easily fabricate large-scale, high-quality perovskite films. Although some methods have been developed for the fabrication of large-scale films, the film quality still needs to be improved. The MA0 healing method, as a post-processing technique, can be perfectly combined with other large-scale methods. However, determining the mechanism of the whole process is crucial for commercial production and subsequent research. Herein, we summarize the research progress on the MA0 healing method. Firstly, we introduce the interaction during the liquification process including the interaction of amines with Pb2+, the generation of hydrogen bonds, the influence of H2O, and the reactions between different amines. Then, we systematically discuss the dynamic mechanism of adsorption and desorption. Finally, several aspects that need to be further clarified are proposed.
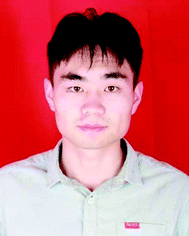
Lianzheng Hao
| Lianzheng Hao received his BEng Degree from the School of Materials Science and Engineering, Qingdao University of Science and Technology in 2019. He is currently a Master's student in Prof. Guanglei Cui's group at Qingdao Institute of Bioenergy and Bioprocess Technology, Chinese Academy of Sciences. His current research focuses on perovskite materials and solar cells. |
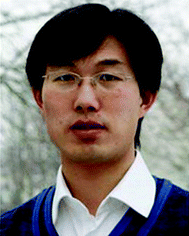
Shuping Pang
| Shuping Pang joined the group of Prof. K. Müllen at the Max Planck Institute for Polymer Research (MPIP) and obtained his PhD Degree in September 2011. Then he moved back to China and worked as a Professor at the Qingdao Institute of Bioenergy and Bioprocess Technology (QIBEBT), Chinese Academy of Sciences (CAS). His current research focus is on photoelectric devices. |
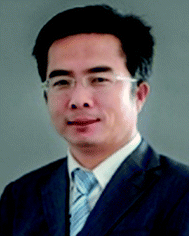
Guanglei Cui
| Guanglei Cui received his PhD Degree from the Institute of Chemistry, CAS, in 2005. He then conducted postdoctoral research at the Max Planck Institute for Polymer Research and Solid States Research before joining QIBEBT, CAS, in 2009. He is currently a Professor and Leader of the Biomimetics for Energy Storage group, Director of the Applied Energy Technology Division, and Deputy Director of the Academic Committee of the QIBEBT. His research topics include sustainable and highly efficient energy-storage materials, all-solid-state batteries, and novel energy devices. |
Introduction
Perovskite solar cells (PSCs) have attracted extensive attention from both the research community and industry due to their advantage of facile fabrication via solution method. Recently, the efficiency of solution-processed small-area PSCs has reached 25.5% certified by NREL,1 while the certified module (802 cm2) just reached 17.9%.2 It remains a great challenge to simply fabricate large-scale, high-quality perovskite films and PSCs with high consistency. Various methods have been developed to fabricate large-scale perovskite films, including but not limited to D-bar and blade coating,3,4 slot-die coating,5–8 spray coating,9–14 dip coating,6,15 and air-knife-assisted coating.16 Generally, solution-processed perovskite polycrystalline films have large roughness and pin hole structures, which is one of the important reasons why the efficiency of the modules is far below that of the small-sized devices.
Considering the uniformity of the perovskite film, methylamine gas (MA0) healing is one of the most promising methods due to its easy processing and high consistency.17–22 The discovery of MA0 healing method was inspired by the reversible transformation of MAPbI3 perovskite phase during the MA0 absorbing and degassing process.21 Generally, the rough MAPbI3 perovskite film with low coverage is visually translucent and matte. After quick exposure to MA0, the film bleached, forming an intermediate liquid phase of MAPbI3·xMA0. Subsequently, MAPbI3·xMA0 could quickly return to the highly uniform dark perovskite phase when the MA0 environment was removed.17 A schematic diagram of this process is shown in Fig. 1a, and scanning electron microscope (SEM) images of the surface morphology before and after MA0 healing are shown in Fig. 1b. This process is generally represented by the following equation:
| MAPbI3 + xMA0 → MAPbI3·xMA0 → MAPbI3 + xMA0 | (1) |
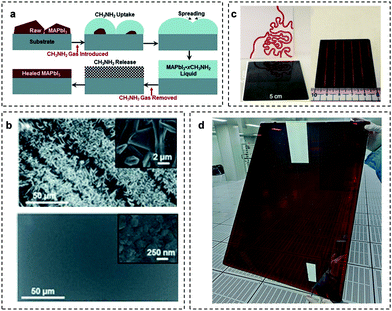 |
| Fig. 1 (a) Schematic diagram of the MA0 healing process. (b) SEM micrographs of MAPbI3 film before (top) and after (bottom) the MA0 healing process. Copyright 2015 Wiley-VCH Verlag GmbH & Co. KGaA, Weinheim. (c) The MAPbI3 module fabricated in the laboratory through MA0 healing method. Copyright 2018 Springer Nature. (d) Large-sized MAPbI3 film (45 × 65 cm2) prepared by MA0 healing method (provided by Suzhou GCL Nano Co. Ltd). | |
This method essentially belongs to post-processing technique and it hardly has high requirements for the initial film. Therefore, the MA0 healing method can perfectly combine the above-mentioned large-scale methods to easily prepare large-sized, highly uniform and lattice-oriented MAPbI3 films. Fig. 1c shows the MAPbI3 module fabricated in the laboratory by this method, where the film has a mirror-smooth surface. Han et al. utilized the complex precursors of MA0·MAI and MA0·PbI2 combined with the pressure processing method to fabricate a 36.1 cm2 MAPbI3 module, reaching a PCE of 12.1%.23 Park et al. applied D-bar coating to fabricate MAPbI3 modules on an area of over 100 cm2, reaching a PCE of 17.82% based on the MA0-assisted MAPbI3 solution.3 The company Suzhou GCL Nano Co. Ltd successfully applied slot-die printing technology combined with MA0 healing method to fabricate large-scale (45 × 65 cm2) highly uniform MAPbI3 films (Fig. 1d). In the initial stage, researches were mainly focused on how to fabricate high-quality perovskite films through this method. Qi et al. reported that introducing MA0 in the annealing process can reduce impurities at the perovskite grain boundaries (GBs) and promote continuity between adjacent grains, and thus reduce defects at the GBs, reaching a high PCE of 18.4%.24 Increasing the degassing temperature of the liquid–solid phase transition from MAPbI3·xMA0 to MAPbI3 could also enhance the quality of the perovskite films with crystal grains in the order of tens of micrometers.25 Additive engineering (such as MACl) is also compatible with the MA0 healing method,26 with the formation of a new intermediate phase, MAPbI3·MACl·xMA0 (PCE = 19.4%). The transformation of the as-prepared MAPbI3 thin film from MAPbI3·MACl·xMA0 to MAPbI3 is a two-step decomposition process, resulting in large, oriented grains spanning the film thickness and ultralow-density GB-network with only vertical GBs. Besides the gas healing of the rough MAPbI3 perovskite films, MA0 was also employed as a cosolvent with the mixing of acetonitrile (ACN) or DMF (PCE > 18%).27–29 Recently, it was further discovered that extending the degassing time at an elevated temperature could increase the grain size, reaching millimeters (PCE = 21.36%).30 Large-grain perovskite films possess a low trap-state density, long charge carrier lifetime, and excellent environmental stability. Through MA0 healing treatment, the device stability also has great enhancement due to the improvement in film quality. For example, Qi et al. reported that unencapsulated PSCs retained 90% of their initial efficiency after 800 h continuous light illumination under an N2 atmosphere, while the control device decayed to 90% of its initial efficiency in just 300 h.31 Song et al. reported that MAPbI3 devices fabricated using the optimized MA0 healing method with ultra-large grains retained 85% of their initial PCEs under illumination after 1000 h in an N2 atmosphere, whereas the control devices decayed to about 60% of their original PCEs.30 Due to the high uniformity of the perovskite films and the device consistency, this MA0 healing method represents a major advance in the scale-up fabrication of high-quality perovskite films and devices.3,22,23,31–34However, the limitation of the MA0 healing method is that it cannot be used for the FAPbI3 Recently, some research groups have started studying the related mechanisms of the whole process.35–37
Herein, we summarize the research progress of the MA0 healing method. This process can be divided into two segments, namely, liquification and recrystallization. In the case of the liquification process, we summarize the internal chemical interaction, which mainly include the interaction of amines with Pb2+, the generation of hydrogen bonds, the influence of H2O and the reactions between different amines. For the recrystallization process, the dynamic mechanism of adsorption and desorption is systematically discussed. Finally, we propose several issues that need to be further investigated.
The internal chemical interaction
The liquification of perovskite materials in MA0 atmosphere is the basis of gas-healing technology. The understanding of underlying chemical reaction and intermolecular interaction is constantly being updated. Initially, Zhou et al.17 supposed that the lone-electron pair in the nitrogen (N) atom of MA0 molecules interacts with the PbI6 octahedra, which leads to a liquefied state. Jen et al.38 applied MAPbBr3 and MASnI3 as the raw film to determine whether the B-site metal ions and X-site halogen make a difference. The transformation phenomenon of MAPbBr3 and MASnI3 is very similar with MAPbI3, which indicates that these two aspects are not determinant. Then, the interaction between MAPbI3 and MA0 was considered as a neutral ligand coordinated with the Pb(II) changing the three-dimensional lead halide framework.39,40 Simultaneously, Rand et al.41 reported that proton transfer occurs from PbI2 to alkylamines, which results in the formation of Pb–MA0 bonds and is responsible for the liquefaction. Also, the presence of an MAPbI3·xMA0 complex has also been proved by witnessing the Tyndall effect in this complex.36,39,42,43 Cahoon et al.44 supposed that the gas healing process should be interpreted as amino-deliquescence and amino-efflorescence, and the process of amino-deliquescence is driven by the highly exothermic dissolution of MAPbI3 in MA0. Some reports have indicated that the existence of hydrogen bonding between NH3+ in MAPbI3 and NH2 in MA0 is also very significant.35,36,39 The formation of MA0–MA+ dimers yields a situation comparable to ionic liquids, which is also extremely important for the liquefaction of perovskite materials.37 Thus, here we introduce the liquification process in detail.
(1) The reaction of amine and Pb2+
MA0 or other amine gases can not only liquify organic–inorganic perovskite structure, but also liquefy the PbI2 structure.23,39,41,45 Qi et al.45 reported that MA0 can penetrate the 2D layered structure of PbI2 and react with the PbI64+ octahedra. Yan et al.39 identified that the MA0 molecules act as a type of ligand coordinating with Pb2+. They supposed that the coordination was the filling of the empty lead 6p orbital (6s26p2) by the lone electron pair from the nitrogen of MA0 (2s2). The Pb–N binding energy calculated by first principles calculation is about 80.04 kJ mol−1. Recently, Hillebrecht et al.35,37 studied the structure of the intermediate phase and the change in chemical bonds in detail. They noted that there are actually two intermediate phases under the reversible reaction of MA0 and MAPbI3. When a stream of MA0 passes over the PbI2 or MAPbI3 powder, a highly viscous liquid is formed, followed by pale-yellow platelets crystallized from it. Through the characterization of single-crystal X-ray diffraction, they obtained a neutral octahedral complex-(MA0)4PbI2. As shown in Fig. 2a, in this structure, it can be seen that four MA0 molecules act as the classical donor ligand to Pb2+, and the two iodine anions exist in a cis-position. If a stronger MA0 stream is injected from a cold trap, the PbI2 or MAPbI3 powder quickly liquefies and colorless crystals are precipitated from it. This colorless crystal is a type of new intermediate phase, which is stable at room temperature if it is kept in a closed vial with pure MA0 as an equilibrium gas phase. The direct measurement of the crystal indicated the existence of multiple twinning. Through further structure refinement of the cubic data, they found that its structure model is the well-known K2PtCl6 type46 with octahedral [Pb(MA0)6]2+ complexes and isolated I− anions (Fig. 2b). In this structure, Pb2+ is only surrounded by the neutral MA0 ligand. When the MA0 is gradually evaporated, [Pb(MA0)6]I2 can be converted to (MA0)4PbI2. The above-mentioned crystal structures provide a crucial basis for understanding the interaction between MA0 and the inorganic framework in perovskite materials. The formation of the liquid state is due to the fact that the MA0 molecules coordinate with Pb2+ as the ligand destroys the initial perovskite crystal structure, and then change its physical state, but there is no evidence that the MA0 healing of perovskite films pass through [Pb(MA0)6]I2 and (MA0)4PbI2 to MAPbI3 finally. Therefore, other liquid intermediates should exist with a disordered structure, which coordinate with a different number of MA0 molecules.
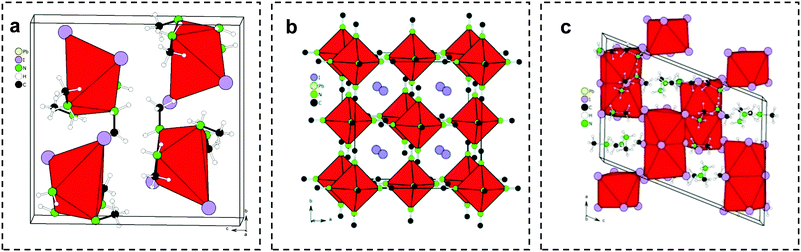 |
| Fig. 2 (a) Crystal structure of (MA0)4PbI2. Copyright 2021 Wiley-VCH GmbH. (b) Crystal structure of [Pb(MA0)6]I2. Copyright The Royal Society of Chemistry 2020. (c) Crystal structure of (MA)5(MA0)2Pb2I9 in (010) direction. Copyright 2021 Wiley-VCH GmbH. | |
(2) The interaction of MA0 and MA+ (generation of hydrogen bonds)
The crystal structure of MAPbI3 can be regarded as [PbI6]4− octahedra in three-dimensional (3D) space, where the MA+ ions fill the gaps among the octahedra.47–49 Some experiments found that organic iodides such as MAI and MACl could also absorb MA0 to form a liquid phase, which is much faster than that in perovskite materials.26,43,50 Thus, the interaction between MA0 and MA+ cannot be ignored. Zheng et al.36 confirmed the existence of MA0–MA+ dimers by applying 1H NMR. They dispersed MAPbI3 powder, MA0–MAPbI3 crystals and MA0 in DMSO-d6 for room-temperature 1H NMR measurements. As shown in Fig. 3a, the 1H NMR signals of MAPbI3 appeared at 7.47 and 2.38 ppm, corresponding to the resonance of the protons adjacent to nitrogen and carbon, respectively, while the 1H NMR signal of the MA0–MAPbI3 sample appeared at 4.51 ppm, which is halfway between 7.47 ppm for MAPbI3 and 1.06 ppm for MA0. They attributed this chemical shift to the hydrogen bonding between NH3+ in MAPbI3 and NH2 in MA0. The formation of hydrogen bonding was also verified by temperature-dependent 1H NMR, as shown in Fig. 3b. The negative correlation between hydrogen bonds and temperature agrees well with the fact that the strength of hydrogen bonding is diminished at elevated temperature.51,52 They also found a similar phenomenon in the MA0–MAPbCl3 and MA0–MAPbBr3 systems. The diameter of the MA0–MA+ dimers is 445 pm, which is about twice that of MA (217 pm). This will easily cause the collapse of the 3D perovskite structures.53
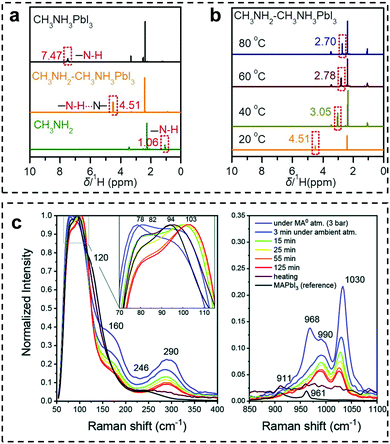 |
| Fig. 3 (a) 1H NMR spectra of MAPbI3 powder, MA0–MAPbI3 crystals and MA0 dispersed in DMSO-d6. (b) Temperature-dependent 1H NMR spectra of MA0–MAPbI3 crystals dispersed in DMSO-d6. The NMR tube with the precursor sample was heated from 20 °C to 80 °C and tested at the corresponding temperatures in situ. Copyright 2020, the American Chemical Society. (c) Raman spectra of a liquified MAPbI3·xMA0 complex during liquid–solid conversion as the MA0 leaves the complex. Copyright The Royal Society of Chemistry, 2020. | |
Around the same time, Andreas Hinsch et al. also confirmed the existence of MA0–MA+ dimers through Raman spectra.35 They exposed the MA0–MAPbI3 complex in the air and measured the change in peaks in the Raman spectra. The only band at 290 cm−1 in the liquid state under high pressure (Fig. 3c) is related to the rotational mode of MA+, which is in agreement with the result from Park et al.3 This rotational mode is normally inactive, and the detectable rotational mode of MA+ can be attributed to the lower local symmetry of the PbI3 framework or the formation of MA0–MA+. During the process of MA0 evaporation, the MA+ rotational mode gradually disappeared.54 In addition, the bands representing the CH3–NH3 rocking and C–N stretching vibrations of the free MA+ under vacuum55 gradually vanished during crystallization, as shown in Fig. 3c. These results demonstrate the existence of MA0–MA+ dimers. According to the above-mentioned two types of reaction, the liquification of MAPbI3 may be driven by two effects, MA0 bonding to Pb2+ atom via the lone electron pair along with the formation of MA0–MA+ dimers. Thus, the chemical reaction for liquification can be described as follows:
| MAPbI3 + xMA0 → Pb(MA0)x−1I2 + [MA0–MA]+I− | (2) |
(3) The influence of H2O
MA0 healing is normally performed in a dry environment, which is due to the negative effect of H2O on the film quality. Qi et al.45 reported that PbI2 can be converted to MAPbI3 in the presence of H2O during the reaction of PbI2 and MA0. Hillebrecht et al.35 also studied this reaction, and they reported that H2O could react with MA0 to produce MA+ cations and OH− anions, generating a new compound, [(MA)5(MA0)2Pb2I9], in the MA0 healing process. This compound contains [Pb2I9]5+ units with two face-sharing octahedra and MA0–MA+ dimers bridged by a hydrogen bond (Fig. 2c). The charge balance of the system is achieved by MA+ and the dimeric (MA0–MA)+ cations. (MA)5(MA0)2Pb2I9 will convert to MAPbI3 by further degassing, but Pb(OH)I remains on the film as a by-product. Walsh et al. analyzed the effect of H2O on the decomposition of MAPbI3. They found that the kinetic barrier of I-ion migration was greatly reduced and all types of vacancy defects were easier to create deep transition levels in the presence of water. These effects could aggravate the decomposition of the perovskite.56 These results emphasize the importance of avoiding humidity during the MA0 healing process, and the liquification process can be represented by eqn (3) with the existence of H2O. | 4MAPbI3 + 4MA0 + 2H2O → (MA)5(MA0)2Pb2I9 + 2Pb(OH)I + MAI | (3) |
(4) The reactions between different amines
(i) Substitution reaction between different amines.
Initially, Zhou et al. reported that ethylamine (C2H5NH2) or n-butylamine (CH3(CH2)3NH2) can liquefy MAPbI3 films, but the film cannot completely recover to the MAPbI3 perovskite phase after the degassing process.17 Thus, they gave the following equation: | MAI + R−NH2 → R−NH3I + MA0 | (4) |
Then Zong et al.57 transformed a rough, polycrystalline NH4PbI3 non-perovskite thin film into a dense, ultrasmooth, textured MAPbI3 perovskite thin film through the MA0 healing process. It is interesting that the yellow NH4PbI3 film initially converts to a black MAPbI3 phase rather than being liquefied directly, and then transforms to MAPbI3·xMA0 under the MA0 environment (Fig. 4a). The whole reaction process can be described by the following two equations: | NH4PbI3 + MA0 → MAPbI3 + NH3 | (5) |
| MAPbI3 + xMA0 → MAPbI3·xMA0 → MAPbI3 + xMA0 | (1) |
Simultaneously, Pang et al.58 replaced NH4PbI3 with “HPbI3” ((CH3)2NH2PbI3)59,60 to fabricate high-quality MAPbI3 films. It is clear that (CH3)2NH2PbI3 (DMAPbI3) is directly liquefied (Fig. 4b), which is different from the behavior of the NH4PbI3 crystals in the MA0 environment. Thus, the reaction can be described as the following equation: | DMAPbI3 + xMA0 → DMAPbI3·xMA0 → MAPbI3 + (x − 1)MA0 + DMA | (6) |
Actually, the conversion of organic–inorganic hybrid perovskites depends on the environment they are kept in. If a type of amine (monoamine) is adequate in the environment, the perovskite will be converted to the corresponding amine structure. The transformation time depends on the volatilization of the amine in the initial perovskite structure. For example, Wang et al.61 confirmed that MAPbI3, BA2PbI4, and OA2PbI4 can transform into each other in the corresponding A site cation gas environment. Chen et al.62 also reported the mutual transformation of MAPbI3 and EAPbI3. Raga et al.45 gave the transformation of different halide perovskites, as shown in Fig. 4c.
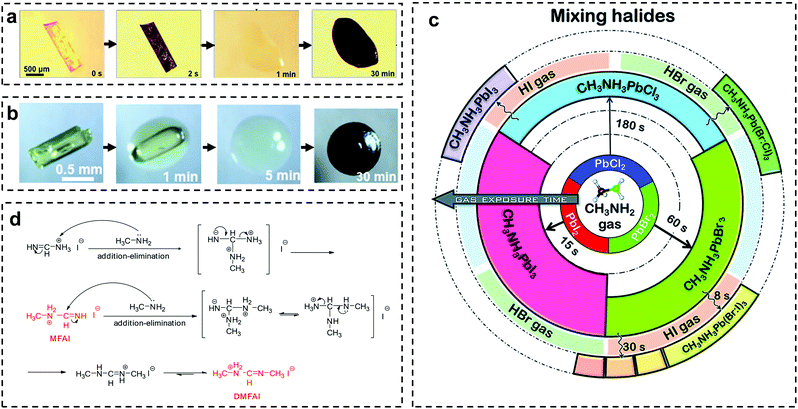 |
| Fig. 4 (a) Optical photos of the morphological changes of NH4PbI3 crystal during the MA0 healing process. Copyright 2016, the American Chemical Society. (b) Optical photos of the morphological changes of “HPbI3” (DMAPbI3) crystal during the MA0 healing process. Copyright 2016, Wiley-VCH Verlag GmbH &Co. KGaA, Weinheim. (c) Perovskite conversion from PbX2 (X = I, Br, and Cl) films exposed to MA gas and to HI and HBr gases. Copyright, The Royal Society of Chemistry, 2016. (d) Addition–elimination reactions between MA0 and FAI. Copyright 2020, Elsevier Inc. | |
(ii) Addition–elimination reaction.
Formamidinium lead iodide (FAPbI3) has become the most widely studied light-absorbing perovskite material, which is ascribed to its suitable bandgap. However, the FAPbI3 perovskite phase cannot be obtained by the MA0 healing method, and its underlying mechanism remained unclear for a long time. Zhou et al.63 directly replaced MA+ with FA gas at 150 °C, where FA0 needed to be in situ prepared due to its instability. Moreover, the FA0 molecules will form the s-triazine structure spontaneously by the addition–elimination reaction.64 This high-temperature gas treatment is only a transformation process without any modification of the film morphology. Zhang et al.65 performed MA0 healing on a mixed organic cation MAxFA1−xPbI3 perovskite film, forming MAPbI3 perovskite films with a second non-perovskite phase similar to the δ-FAPbI3 structure. Recently,66 Wang et al. discovered that FAI will undergo addition–elimination reactions with MA0. The reaction mechanism of the reaction is shown in Fig. 4d. The lone-pair electrons of the N atom in MA0 have nucleophilicity and the imine bond in FAI is an active electrophilic group, which leads to an addition–elimination reaction to form N-methyl formamidinium iodide (MFAI) and NH3. Similarly, the formed MFAI also has an imine bond, which can undergo a second addition–elimination reaction with MA0 to form N,N′-dimethyl formamidinium iodide (DMFAI). Therefore, the gas healing of the rough FAPbI3- or FA-containing perovskite films to a highly uniform one is still a big challenge.
The dynamics of the recrystallization
During the MA0 healing process, the next step is desorption-induced recrystallization of the perovskite film. Control of this step is also very important in the quality of perovskite films. Firstly, the absorption capacity determines the state of the intermediate and affects the subsequent recrystallization process. Then, the desorption of MA0 affects the generation of a crystal nucleus and its growth process. Thus, it is necessary to determine the dynamic mechanism of these processes.
(1) Adsorption of MA0 gas
The first factor affecting the adsorption capacity of MAPbI3 to absorb MA0 is the pressure. Jen et al.38 observed that MAPbI3 films preferred to completely liquefy under a high MA0 pressure compared with low pressure. Under high MA0 vapor pressure, the diffusion of MA0 molecules could quickly break the physical bonds of the initial material (Fig. 5a). In contrast, under a low MA0 vapor pressure, the diffusion of MA0 is constrained to the initial physical extent (Fig. 5b). This is consistent with the conclusion of Hillebrecht et al.,35 who found that the intermediate phase is [Pb(CH3NH2)6]I2 under high MA0 vapor, and that under low MA0 vapor pressure is (CH3NH2)4PbI2.
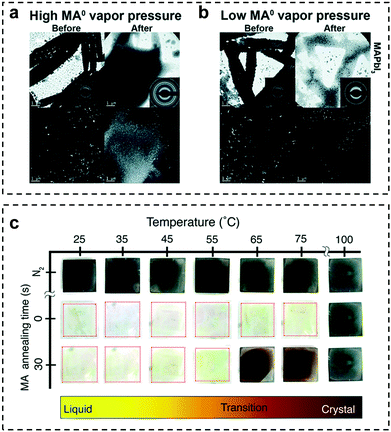 |
| Fig. 5 TEM images of MAPbI3 before and after exposure to MA0 vapor. Each pane contains four pictures, the top line are the bright field images and select area electron diffraction (inset). The bottom line are the dark field images. (a) High MA0 vapor pressure and (b) low MA0 vapor pressure. Copyright, The Royal Society of Chemistry, 2016. (c) Temperature-dependent annealing of MAPbI3 under MA gas atmosphere. First line: images of the films under an N2 flow after 5 minutes. Second line: images of the films immediately after exposure to MA atmosphere. Third line: images of the samples after 30 seconds under a continual flowing MA atmosphere. Copyright, The Royal Society of Chemistry 2016. | |
Temperature is another factor affecting the adsorbing capacity of MAPbI3 films to absorb MA0. Zang et al.25 discovered the phenomenon that the liquid MAPbI3·xMA0 intermediate phase under steady MA0 atmosphere still transform to solid state at elevated temperature. As shown in Fig. 5c, all the MAPbI3 samples are exposed to nitrogen or MA0 atmosphere maintained from 25 °C to 100 °C respectively. It is clear that high temperature is conducive to the degassing of perovskite films, appearing a black perovskite phase. When the temperature exceeds 55 °C, the liquid phase starts transitioning back to the solid state even under MA atmosphere. At 100 °C, no liquid intermediate phase exists.
(2) Desorption of MA0 gas
Temperature also has a great effect on the degassing process. Zang et al.25 heated the liquid phase at 100 °C under an MA0 atmosphere and the grain size of the film reached tens of microns. Besides, the release speed of MA0 is directly related to crystallization. Song et al.30 studied the influence of the MA0 release speed on the crystallization process from MAPbI3·xMA0 to MAPbI3. In the traditional MA0 gas healing process, the pressure of MA0 quickly decreases (close to 0) by removing the MA0 environment as soon as possible. The quick formation of supersaturation leads to the formation of massive nuclei and fine-grained (∼100 nm in size) thin films. Thus, high supersaturation is detrimental to the formation of large grains. Accordingly, slowing the release speed of MA0 molecules from the liquid intermediate could lead to a low nucleation density and long growth time of the grains. As shown in Fig. 6a, the grain size is strongly dependent on the release time. The degassing process was conducted at 120 °C, and the grain size increased to millimetres when the degassing time was 30 s. With a further increase in the degassing time, the grain size seemed to no longer expand. Besides, it has been proven that the as-prepared perovskite films possessed a (110)-uniaxial crystallographic orientation and small full width of half maximum (FWHM), exhibiting a very low trap density, long carrier lifetime, and excellent environmental stability.
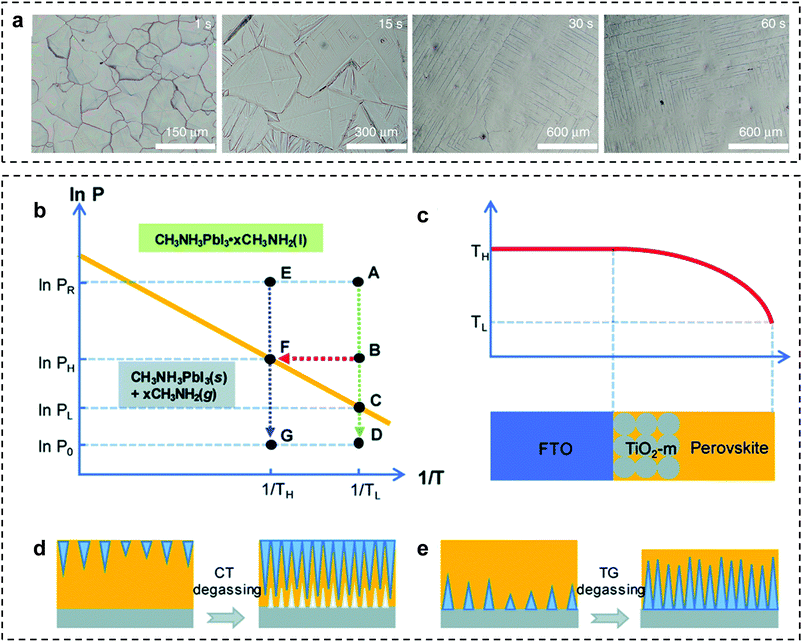 |
| Fig. 6 (a) Optical microscopic images of the MAPbI3 perovskite films prepared from the liquid intermediate with different degassing times (1, 15, 30, and 60 s). Copyright 2020, Springer Nature. (b) Thermodynamic model of the MA0 desorption process. PR is the pressure of MA0 reacting with the perovskite film. P0 is the actual pressure of the gas desorption process. (c) Diagram of the temperature gradient in the device. Schematic of the crystal growth model with (d) CT-MA (conventional MA0 healing) method and (e) TG-MA (temperature gradient-induced MA0 healing) method. Copyright The Royal Society of Chemistry, 2020. | |
It is clear that the fast removal of the solvent can highly enhance the uniformity of perovskite films.24,27,67,68 Considering this, in solar cell devices, the carrier transport is mainly in the vertical direction. The directional growth of the perovskite layer to form a grain structure in the through-thickness direction is necessary. According to the Clausius–Clapeyron equation69,70 (Fig. 6b), the point on the oblique line represents that MAPbI3·xMA0 is in an equilibrium state. When the MA0 pressure is lower than the value on the line, MAPbI3·xMA0 begins to degas. In the conventional MA0 gas healing process at room temperature, the process from A to D happens instantaneously. Supersaturation is the driving force behind the crystallization, corresponding to the C–D process in Fig. 6b. After MA0 is desorbed from the surface, the perovskite film starts the nucleation process from the surface and continues to nucleate throughout the whole film, which will cause the formation of pinhole structures in the perovskite film (Fig. 6d). It is clear that the higher the temperature, the easier the desorption reaction will occur, as shown in Fig. 6b with the corresponding gas desorption process from E to G. In this case, a longitudinal temperature gradient (TG degassing) was formed to induce the directional growth of perovskite film.71 The raw FTO/TiO2/MAPbI3 substrate was preheated to 60 °C, and then subjected to the MA0 healing process with cold MA0. The surface temperature of the perovskite film quickly decreased, while the bottom of the film remained at a relatively higher temperature due to the large enthalpy of the glass substrate, as shown in Fig. 6c. It was proven that the crystallization started from the bottom of the film, and then grew upward, as shown in Fig. 6e, resulting in a reduction in defects in the perovskite film and improved carrier injection efficiency in the electron selective layer.
Conclusion and outlook
In this review, we summarized the knowledge on the MA0 healing method. Firstly, we introduced the internal chemical interaction. In the current perception, MAPbI3 liquefaction may be driven by two effects, MA0 bonding to the Pb2+ atom via its lone electron pair along with the formation of MA0–MA+. (Moreover, humidity should be avoided during the reaction, given that H2O will participate in the reaction and produce Pb(OH)I as the by-product. Also different types of R–NH2PbI3 can transform mutually if the corresponding amine is adequate.) Then, we introduced the dynamics of recrystallization. The temperature, MA0 pressure, and release speed of MA0 all have a great effect on the recrystallization. Although, the chemical basis of the reaction between MA0 and MAPbI3 is relatively clear, there are still some areas that are undefined and need further investigation.
1. In the process of industrial production, adequate fluidity is needed for the intermediate phase to repair the hole defect in the initial film. We mentioned above that the MA0 molecule, acting as a ligand, coordinates with Pb2+. However, the influence of the number of MA0 molecules coordinated with Pb2+ on the viscosity and surface tension of the intermediate is still unclear. Thus, a detailed investigation on this will be helpful for industrial production.
2. It has been reported that some MA0 molecules can remain at the grain boundary of the MAPbI3 layers after the MA0 healing treatment.32 It is still unclear whether the MA0 molecules are embedded in the lattice and their impact on the long-term stability of the encapsulated devices.
3. There are some pathways to obtain large grain films such as increasing the temperature, retarding the degassing speed, and adding additives. The obtained perovskite film normally has large grains and excellent uniaxial orientation. However, whether each grain is a single crystal is still under debate. Thus, the defect density in the large gains should be further investigated.
4. Recently, FA-rich perovskite materials have been shown to be more stable and highly efficient photovoltaic materials. However, direct MA0 gas healing to repair FAPbI3 materials is not feasible due to the reaction between FAI and MA0, and there are also difficulties in repairing FAPbI3 materials through direct FA0 healing due to the instability of FA0. Therefore, a suitable gas healing method for FA-containing perovskite films is still lacking.
Conflicts of interest
There are no conflicts to declare.
Acknowledgements
This work was supported by the funding of Shandong Energy Institute (SEI I202129), Youth Innovation Promotion Association CAS (Y201944), Natural Science Foundation of Shandong Province (ZR2020KB001 & ZR2018ZB0315). We also thank Suzhou GCL Nano Co. Ltd for the fabrication of large sized perovskite films, and the Qingdao Key Lab of Solar Energy Utilization & Energy Storage Technology.
References
- N. R. E. Laboratory, Best research-cell efficiency chart https://www.nrel.gov/pv/cell-efficiency.html.
- N. R. E. Laboratory, Champion Photovoltaic Module Efficiency Chart, https://www.nrel.gov/pv/module-efficiency.html.
- D.-N. Jeong, D.-K. Lee, S. Seo, S. Y. Lim, Y. Zhang, H. Shin, H. Cheong and N.-G. Park, ACS Energy Lett., 2019, 4, 1189–1195 CrossRef CAS.
- Y. Deng, X. Zheng, Y. Bai, Q. Wang, J. Zhao and J. Huang, Nat. Energy, 2018, 3, 560–566 CrossRef CAS.
- J.-E. Kim, Y.-S. Jung, Y.-J. Heo, K. Hwang, T. Qin, D.-Y. Kim and D. Vak, Sol. Energy Mater. Sol. Cells, 2018, 179, 80–86 CrossRef CAS.
- L. Huang, C. Li, X. Sun, R. Xu, Y. Du, J. Ni, H. Cai, J. Li, Z. Hu and J. Zhang, Org. Electron., 2017, 40, 13–23 CrossRef CAS.
- M. He, B. Li, X. Cui, B. Jiang, Y. He, Y. Chen, D. O’Neil, P. Szymanski, M. A. Ei-Sayed, J. Huang and Z. Lin, Nat. Commun., 2017, 8, 16045 CrossRef CAS PubMed.
- F. Ye, H. Chen, F. Xie, W. Tang, M. Yin, J. He, E. Bi, Y. Wang, X. Yang and L. Han, Energy Environ. Sci., 2016, 9, 2295–2301 RSC.
- M. Park, W. Cho, G. Lee, S. C. Hong, M. C. Kim, J. Yoon, N. Ahn and M. Choi, Small, 2019, 15, e1804005 CrossRef PubMed.
- W. C. Chang, D. H. Lan, K. M. Lee, X. F. Wang and C. L. Liu, ChemSusChem, 2017, 10, 1405–1412 CrossRef CAS PubMed.
- S. Das, B. Yang, G. Gu, P. C. Joshi, I. N. Ivanov, C. M. Rouleau, T. Aytug, D. B. Geohegan and K. Xiao, ACS Photonics, 2015, 2, 680–686 CrossRef CAS.
- Z. Liang, S. Zhang, X. Xu, N. Wang, J. Wang, X. Wang, Z. Bi, G. Xu, N. Yuan and J. Ding, RSC Adv., 2015, 5, 60562–60569 RSC.
- J. H. Heo, M. H. Lee, M. H. Jang and S. H. Im, J. Mater. Chem. A, 2016, 4, 17636–17642 RSC.
- D. K. Mohamad, J. Griffin, C. Bracher, A. T. Barrows and D. G. Lidzey, Adv. Energy Mater., 2016, 6, 1066994 Search PubMed.
- H. C. Liao, P. Guo, C. P. Hsu, M. Lin, B. Wang, L. Zeng, W. Huang, C. M. M. Soe, W. F. Su, M. J. Bedzyk, M. R. Wasielewski, A. Facchetti, R. P. H. Chang, M. G. Kanatzidis and T. J. Marks, Adv. Energy Mater., 2016, 7, 1601660 CrossRef.
- L.-L. Gao, C.-X. Li, C.-J. Li and G.-J. Yang, J. Mater. Chem. A, 2017, 5, 1548–1557 RSC.
- Z. Zhou, Z. Wang, Y. Zhou, S. Pang, D. Wang, H. Xu, Z. Liu, N. P. Padture and G. Cui, Angew. Chem., Int. Ed., 2015, 54, 9705–9709 CrossRef CAS PubMed.
- S. R. Raga, Y. Jiang, L. K. Ono and Y. Qi, Energy Technol., 2017, 5, 1750–1761 CrossRef CAS.
- C. Li, S. Pang, H. Xu and G. Cui, Solar RRL, 2017, 1, 1700076 CrossRef.
- J.-a. Yang, T. Qin, L. Xie, K. Liao, T. Li and F. Hao, J. Mater. Chem. C, 2019, 7, 10724–10742 RSC.
- Y. Zhao and K. Zhu, Chem. Commun., 2014, 50, 1605–1607 RSC.
- Y. Zhou and N. P. Padture, ACS Energy Lett., 2017, 2, 2166–2176 CrossRef CAS.
- H. Chen, F. Ye, W. Tang, J. He, M. Yin, Y. Wang, F. Xie, E. Bi, X. Yang, M. Gratzel and L. Han, Nature, 2017, 550, 92–95 CrossRef CAS PubMed.
- Y. Jiang, E. J. Juarez-Perez, Q. Ge, S. Wang, M. R. Leyden, L. K. Ono, S. R. Raga, J. Hu and Y. Qi, Mater. Horiz., 2016, 3, 548–555 RSC.
- D. L. Jacobs and L. Zang, Chem. Commun., 2016, 52, 10743–10746 RSC.
- F. Ji, S. Pang, L. Zhang, Y. Zong, G. Cui, N. P. Padture and Y. Zhou, ACS Energy Lett., 2017, 2, 2727–2733 CrossRef CAS.
- N. K. Noel, S. N. Habisreutinger, B. Wenger, M. T. Klug, M. T. Hörantner, M. B. Johnston, R. J. Nicholas, D. T. Moore and H. J. Snaith, Energy Environ. Sci., 2017, 10, 145–152 RSC.
- A. J. Ramadan, N. K. Noel, S. Fearn, N. Young, M. Walker, L. A. Rochford and H. J. Snaith, Chem. Mater., 2018, 30, 7737–7743 CrossRef CAS.
- Z. Liu, J. Hu, H. Jiao, L. Li, G. Zheng, Y. Chen, Y. Huang, Q. Zhang, C. Shen, Q. Chen and H. Zhou, Adv. Mater., 2017, 29, 1606774 CrossRef PubMed.
- H. Fan, F. Li, P. Wang, Z. Gu, J. H. Huang, K. J. Jiang, B. Guan, L. M. Yang, X. Zhou and Y. Song, Nat. Commun., 2020, 11, 5402 CrossRef CAS PubMed.
- Z. Liu, L. Qiu, E. J. Juarez-Perez, Z. Hawash, T. Kim, Y. Jiang, Z. Wu, S. R. Raga, L. K. Ono, S. Liu and Y. Qi, Nat. Commun., 2018, 9, 1–11 CrossRef PubMed.
- K. Huang, C. Wang, C. Zhang, S. Tong, H. Li, B. Liu, Y. Gao, Y. Dong, Y. Gao, Y. Peng and J. Yang, Org. Electron., 2018, 55, 140–145 CrossRef CAS.
- L. Hong, Y. Hu, A. Mei, Y. Sheng, P. Jiang, C. Tian, Y. Rong and H. Han, Adv. Funct. Mater., 2017, 27, 1703060 CrossRef.
- Z. Shao, Z. Wang, Z. Li, Y. Fan, H. Meng, R. Liu, Y. Wang, A. Hagfeldt, G. Cui and S. Pang, Angew. Chem., Int. Ed., 2019, 58, 5587–5591 CrossRef CAS PubMed.
- D. Bogachuk, L. Wagner, S. Mastroianni, M. Daub, H. Hillebrecht and A. Hinsch, J. Mater. Chem. A, 2020, 8, 9788–9796 RSC.
- X. Huang, R. Chen, G. Deng, F. Han, P. Ruan, F. Cheng, J. Yin, B. Wu and N. Zheng, J. Am. Chem. Soc., 2020, 142, 6149–6157 CrossRef CAS PubMed.
- M. Daub and H. Hillebrecht, Eur. J. Inorg. Chem., 2021, 1490–1497 CrossRef CAS.
- T. Zhao, S. T. Williams, C.-C. Chueh, D. W. deQuilettes, P.-W. Liang, D. S. Ginger and A. K. Y. Jen, RSC Adv., 2016, 6, 27475–27484 RSC.
- M. Long, T. Zhang, H. Zhu, G. Li, F. Wang, W. Guo, Y. Chai, W. Chen, Q. Li, K. S. Wong, J. Xu and K. Yan, Nano Energy, 2017, 33, 485–496 CrossRef CAS.
- X. Y. Feng, K. W. Ng, S. P. Wang, W. Z. Chen, Z. Z. Zhang, W. Chen, Y. Y. Zhao, B. Tu, Z. K. Tang, H. Pan and Z. B. He, J. Mater. Chem. A, 2020, 8, 13585–13593 RSC.
- R. A. Kerner, T. H. Schloemer, P. Schulz, J. J. Berry, J. Schwartz, A. Sellinger and B. P. Rand, J. Mater. Chem. C, 2019, 7, 5251–5259 RSC.
- C. Wu, H. Li, Y. Yan, B. Chi, J. Pu, J. Li, M. Sanghadasa and S. Priya, Nano Energy, 2017, 36, 295–302 CrossRef CAS.
- R. G. Niemann, A. G. Kontos, D. Palles, E. I. Kamitsos, A. Kaltzoglou, F. Brivio, P. Falaras and P. J. Cameron, J. Phys. Chem. C, 2016, 120, 2509–2519 CrossRef CAS.
- J. K. Meyers, L. Y. Serafin, A. D. Orr and J. F. Cahoon, Chem. Mater., 2021, 33, 3814–3822 CrossRef CAS.
- S. R. Raga, L. K. Ono and Y. Qi, J. Mater. Chem. A, 2016, 4, 2494–2500 RSC.
- R. Eßmann, G. Kreiner, A. Niemann, D. Rechenbach, A. Schmieding, T. Sichla, U. Zachwieja and H. Jacobs, Z. Anorg. Allg. Chem., 1996, 622, 1161–1166 CrossRef.
- S. Luo and W. A. Daoud, Materials, 2016, 9, 123 CrossRef PubMed.
- X. Guo, C. McCleese, C. Kolodziej, A. C. Samia, Y. Zhao and C. Burda, Dalton Trans., 2016, 45, 3806–3813 RSC.
- M. W. Lufaso and P. M. Woodward, Acta Crystallogr., Sect. B: Struct. Sci., 2001, 57, 725–738 CrossRef CAS PubMed.
- Y. Chang, L. Wang, J. Zhang, Z. Zhou, C. Li, B. Chen, L. Etgar, G. Cui and S. Pang, J. Mater. Chem. A, 2017, 5, 4803–4808 RSC.
- S. H. Gellman, G. P. Dado, G. B. Liang and B. R. Adams, J. Am. Chem. Soc., 1991, 113, 1164–1173 CrossRef CAS.
- E. D. Walter, L. Qi, A. Chamas, H. S. Mehta, J. A. Sears, S. L. Scott and D. W. Hoyt, J. Phys. Chem. C, 2018, 122, 8209–8215 CrossRef CAS.
- W.-J. Yin, J.-H. Yang, J. Kang, Y. Yan and S.-H. Wei, J. Mater. Chem. A, 2015, 3, 8926–8942 RSC.
- C. Quarti, G. Grancini, E. Mosconi, P. Bruno, J. M. Ball, M. M. Lee, H. J. Snaith, A. Petrozza and F. D. Angelis, J. Phys. Chem. Lett., 2014, 5, 279–284 CrossRef CAS PubMed.
- T. Glaser, C. Muller, M. Sendner, C. Krekeler, O. E. Semonin, T. D. Hull, O. Yaffe, J. S. Owen, W. Kowalsky, A. Pucci and R. Lovrincic, J. Phys. Chem. Lett., 2015, 6, 2913–2918 CrossRef CAS PubMed.
- Y. H. Kye, C. J. Yu, U. G. Jong, Y. Chen and A. Walsh, J. Phys. Chem. Lett., 2018, 9, 2196–2201 CrossRef CAS PubMed.
- Y. Zong, Y. Zhou, M. Ju, H. F. Garces, A. R. Krause, F. Ji, G. Cui, X. C. Zeng, N. P. Padture and S. Pang, Angew. Chem., Int. Ed., 2016, 55, 14723–14727 CrossRef CAS PubMed.
- S. Pang, Y. Zhou, Z. Wang, M. Yang, A. R. Krause, Z. Zhou, K. Zhu, N. P. Padture and G. Cui, J. Am. Chem. Soc., 2016, 138, 750–753 CrossRef CAS PubMed.
- W. Ke, I. Spanopoulos, C. C. Stoumpos and M. G. Kanatzidis, Nat. Commun., 2018, 9, 4785 CrossRef PubMed.
- M. Daub and H. Hillebrecht, Z. Anorg. Allg. Chem., 2018, 644, 1393–1400 CrossRef CAS.
- J. Wang, X. Yao, W.-J. Xiao, S. Wang, G. Xu, X.-Q. Chen, S.-C. Wu, I. Visoly-Fisher, E. A. Katz, Y. Li, J. Lin, W.-S. Li and Y. Li, Solar RRL, 2018, 2, 1800125 CrossRef.
- F. Wang, W. Li, H. Liu, L. Zhu and H. Chen, Nanoscale, 2019, 11, 14465–14471 RSC.
- Y. Zhou, M. Yang, S. Pang, K. Zhu and N. P. Padture, J. Am. Chem. Soc., 2016, 138, 5535–5538 CrossRef CAS PubMed.
- C. Chen, Y. Rao, Z. Li, X. Wang, G. Cui, W. Wang and S. Pang, Solar RRL, 2021, 5, 2000715 CrossRef CAS.
- Y. Zhang, Z. Zhou, F. Ji, Z. Li, G. Cui, P. Gao, E. Oveisi, M. K. Nazeeruddin and S. Pang, Adv. Mater., 2018, 30, e1707143 CrossRef PubMed.
- X. Wang, Y. Fan, L. Wang, C. Chen, Z. Li, R. Liu, H. Meng, Z. Shao, X. Du, H. Zhang, G. Cui and S. Pang, Chemistry, 2020, 6, 1369–1378 CrossRef CAS.
- M. Long, T. Zhang, Y. Chai, C.-F. Ng, T. C. W. Mak, J. Xu and K. Yan, Nat. Commun., 2016, 7, 1–11 Search PubMed.
- T. Zhang, N. Guo, G. Li, X. Qian, L. Li and Y. Zhao, J. Mater. Chem. A, 2016, 4, 3245–3248 RSC.
- L. M. Wheeler, D. T. Moore, R. Ihly, N. J. Stanton, E. M. Miller, R. C. Tenent, J. L. Blackburn and N. R. Neale, Nat. Commun., 2017, 8, 1722 CrossRef PubMed.
- V. Goetz and A. Marty, Chem. Eng. Sci., 1992, 47, 4445–4454 CrossRef CAS.
- L. Hao, Z. Li, L. Wang, R. Liu, Z. Shao, Z. Zhou, X. Guo, G. Cui, S. F. Liu and S. Pang, J. Mater. Chem. A, 2020, 8, 17019–17024 RSC.
|
This journal is © The Royal Society of Chemistry 2022 |