Well-defined hierarchical teddy bear sunflower-like NiCo2O4 electrocatalyst for superior water oxidation†
Received
13th August 2022
, Accepted 3rd November 2022
First published on 9th November 2022
Abstract
The development of a robust and efficient electrocatalyst for water oxidation is challenging due to the large overpotential requirement to transfer four electrons. Herein, a novel spinel-type hierarchical teddy bear sunflower-like NiCo2O4 electrocatalyst was synthesized through the facile solvothermal process and evaluated for the challenging and demanding oxygen evolution reaction (OER) in the water electrolysis process. The teddy bear sunflower-like NiCo2O4 supported on nickel foam (NF) delivers a current density of 50 mA cm−2 at a small water oxidation overpotential (η50 = 319 mV) which is significantly lower than that of the corresponding spherical NiO/NF (η50 = 338 mV), and sea-urchin like Co3O4/NF (η50 = 357 mV). A large specific and electroactive surface area, as well as a high TOF value exhibited by the hierarchical teddy bear sunflower-like NiCo2O4 electrocatalyst, demonstrates the potential of NiCo2O4 to catalyze the water oxidation reaction efficiently. The impact of the near-Fermi-level d-orbital states in NiCo2O4 electrocatalyst for boosting OER activity was unveiled by the density functional theory calculation. The stable performance even after 16 h and high catalyst utilization of the hierarchical teddy bear sunflower-like NiCo2O4 through the OER indicates that the catalyst is highly suitable for the large-scale water oxidation process.
1. Introduction
At present, hydrogen generation via water splitting has been the subject of research for the reason that green energy plays a crucial role in environmental fortification.1 The hydrogen evolution reaction (HER) and oxygen evolution reaction (OER) occur at the cathode and anode, respectively and are the processes involved in electrochemical water-splitting. In this mechanism, the OER is of particular interest, as it is a four-electron transport reaction and demands high overpotential.2,3 In this context, it is imperative to develop highly efficient and stable water oxidation electrocatalysts that will reduce the overpotential value and accelerate the process. To date, noble metal-based catalysts such as the oxides of ruthenium (RuO2) and iridium (IrO2) are the most preferred catalysts for OER due to their low overpotential for OER.4,5 However, the global low reserves and high costs of these metals limit their use on an industrial scale. To overcome these issues, scientists have come up with low-cost transition metal hydroxides/oxides, phosphides, and sulfides which have shown better performance in water electrolysis.6–9 More specifically, the transition metals, particularly Co, Ni, Fe, and Mn-based materials, are being considered owing to their superior OER performance for potentially outperforming catalysts based on noble metals.10,11 To be practically advantageous, the catalyst needs to remain stable while approaching high current densities (up to 200 mA cm−2) by incurring low overpotentials. Thus, it is essential to investigate the electrocatalyst, which will operate at high current densities and need very little overpotential.
Amongst various transition metal compounds, nickel/cobalt-based hydroxide and oxides have been extensively studied for electrochemical water splitting owing to their multiple oxidation states, high active sites, high stability, spinel structure, high abundance, and low cost.12,13 However, their intrinsic insulating nature limits their practical use.14 Thus, various supports, such as Ni-foam, carbon cloth, carbon black, etc., have been used to increase the conductivity of the material.15,16 These kinds of supports provide electric pathways to nanoparticles, prevent agglomeration during the electrolysis of water, and increase the efficiency of catalyst utilization.17,18 Additionally, to catalyze the OER process, it is important to design the catalyst with a very high specific and active surface area and optimal electrical conductivity. Various approaches have emerged to improve the catalytic activity, such as tuning the morphology of the material, elemental doping, and interfacial engineering.19–23 Hierarchical structures generally offer higher surface area and active sites and provide better catalytic performance.24 Materials with hierarchical architectures have been extensively utilized for electrochemical applications due to the fast diffusion of ions and acceleration of surface reaction during electrolysis.25 Moreover, 3D architectures composed of 1D nanostructures are more beneficial in water-splitting reactions. Such complex structures promote the release of gas bubbles and display enhanced catalytic performance. Thus, it is of great interest to design such 3D hierarchical assemblies which consist of 1D nanostructures, thus making the water oxidation process more efficient.
Spinel AB2O4 has been considered a promising, efficient, and stable candidate for promoting water oxidation in alkaline solution. In this spinel structure, A and B occupy a combination of tetrahedral and octahedral sites with different oxidation states.26 A spinel NiCo2O4 has shown eye-catching potential in energy storage and energy conversion applications due to the existence of rich multiple oxidations states wherein, Ni2+ occupies the tetrahedral (Td) sites with eg4 t2g4 electronic configuration, and Co3+ occupies the octahedral (Oh) sites with eg0 t2g6 electronic configuration.27,28 The partially filled 3d orbitals in the spinel structure play a key role in generating outstanding electrochemical properties by facilitating the electron transfer process. Experimental observations and theoretical analysis have been performed to identify the active centers and role of contributing orbital.29,30 For instance, Baglio et al. suggested that the Co3+ and Ni3+ were accountable for better OER performance of NiCo2O4.31 Shanmugam and coworkers reported that the high concentration of Ni3+ in the NiCo2O4 electrode material enhanced the electrochemical performance.32 Liu et al. established improved OER performance by engineering NiO with Ni3+ ions.33 Thus, the determination of surface-active species with their oxidation states that are responsible for enhancing the catalytic activity becomes a significant interest. By employing physicochemical characterization tools such as X-ray photoelectron spectroscopy and cyclic voltammetry with low scan rates, the oxidation states of the surface-active species could be determined.
To develop a low-cost, efficient and stable OER electrocatalyst, we describe a facile approach to prepare hierarchical teddy bear sunflower-like NiCo2O4, spherical NiO, and sea-urchin-like Co3O4 using the surfactant-assisted solvothermal procedure. The prepared mesoporous hierarchical teddy bear sunflower-like NiCo2O4/NF catalyst displayed excellent water oxidation performance even at high current densities. To drive the current densities of 10 mA cm−2, 50 mA cm−2, and 200 mA cm−2, the NiCo2O4/NF catalyst requires low overpotential of 290 mV, 319 mV and 330 mV, respectively. Furthermore, the electronic structure and charge transport mechanism of all three structures were systematically investigated by assessing density functional theory (DFT) and supported by experimental studies. The developed highly efficient OER catalyst by facile solvothermal route holds great potential for large-scale industrial applications.
2. Experimental
2.1 Materials
Nickel(II) nitrate hexahydrate (Ni(NO3)2·6H2O), cobalt(II) nitrate hexahydrate (Co(NO3)2·6H2O), urea (CO(NH)2), cetyltrimethylammonium bromide (CH3(CH2)15N(CH3)3Br) (CTAB), cyclohexane (C6H12), and 5 wt% Nafion dispersion D520 were purchased from Sigma-Aldrich. All materials used in the synthesis and electrochemical testing were of analytical grade and used as received without further purification.
2.2 Synthesis of NiCo2O4, NiO, and Co3O4 catalysts
For the synthesis of the NiCo2O4 catalyst, Co(NO3)2·6H2O and Ni(NO3)2·6H2O metal precursors were used in a solvothermal method. At first, CO(NH)2, 0.66 M, and CTAB, 0.20 M were mixed in cyclohexane (C6H12, 35 mL). In 35 mL of distilled water, the metal precursors having 0.177 M of Co(NO3)2·6H2O and 0.190 M Ni(NO3)2·6H2O, were dissolved. A homogeneous mixture is obtained by adding precursor solution into cyclohexane by continuous stirring at 400 rpm for about 30 min. The solvothermal reaction was conducted in a hot-air oven at 120 °C for 4 h. The gained products in the powder form were then washed and dried in the air in an oven at 60 °C for 8 h followed by annealing at 450 °C for 4 h in the air to get the NiCo2O4 phase. The NiO and Co3O4 samples are prepared by the previously reported method.34,35 The Co3O4 and NiO catalysts were solvothermal synthesized using Co(Cl)2·6H2O, 0.177 M and Ni(NO3)2·6H2O, 0.177 M metal precursors, respectively. Both reactions were carried out by keeping all other preparative parameters invariant as that of the NiCo2O4 catalyst. The prepared catalysts were then used for further structural and surface analysis, followed by water oxidation performance investigations.
2.3 Characterization details
The prepared samples were analyzed by employing various structural and surface characterization techniques. The morphology of the samples was studied with scanning electron microscopy (FE-SEM, JSM-7600F, JEOL). Transmission electron microscopy (TEM, JEM 2100, 200 kV) was used to determine the microstructure of the samples. The lattice spacings in the crystal structure were determined by high-resolution transmission electron microscopy (HR-TEM, JEM 2100, 200 kV). The selected area electron diffraction (SAED) patterns were acquired using the same HR-TEM machine. EDX and elemental mapping were acquired by STEM-EDX JEM-F200(URP). The crystallinity and phase of the samples were determined using a powder X-ray diffractometer (XRD) (Rigaku Ultima IV) with Cu-kα irradiation. X-ray photoelectron spectroscopy (XPS, K ALPHA Thermo Fisher, Al Kα) was utilized to investigate the surface chemistry of the catalysts. With the help of Avantage software, the concentration of surface atoms of the prepared samples was estimated. Brunauer–Emmett–Teller (BET) surface area analyzer (Micromeritics ASAP 2000) was utilized to obtain the N2 adsorption–desorption isotherm and physical surface area.
2.4 DFT calculations
The ab initio modeling is performed using Quantum ESPRESSO (QE).36,37 The projector augmented wave method (PAW) is used for calculations to describe the ion–electron interaction. A primitive cell of normal spinel of Ni2Co4O8 (contains two formula units) is used with a wave function cut-off of 90 Ry to truncate the plane wave expansion. The energy cut-off was set to 10−6 Ry, and the cut-off for forces was set to 10−4 Ry bohr−1. Spin-polarized density functional theory (DFT) calculations are performed using generalized gradient approximation in which Perdew–Burke–Ernzerhof (PBE) functional describes the exchange and correlation.38 Dudarev et al. proposed the use of Hubbard U correction to account for the correlation effect between 3d electrons of transition metals.39 Thus, we first benchmark the value of Hubbard U correction for Ni and Co. We get an energy minimum for U values of 3.0 and 1.6 for Co and Ni, respectively. The k-mesh of 7 × 7 × 7 is used for geometry optimization of NiCo2O4, and 14 × 14 × 14 is used for density of state (DOS) and partial-DOS (pDOS) calculations. The high symmetry points are generated based on Aflow.40
2.5 Electrochemical study
The electrochemical water oxidation performance was investigated using linear scale and cyclic voltammetry (CV) techniques. Three-electrode system attached to the Autolab electrochemical workstation was used for electrochemical measurements. Initially, catalyst powder (5 mg) was dissolved in a mixture of Nafion (25 μL) and ethanol (1 ml), and the solution was sonicated for 10 min. Then 20 μL of prepared ink was drop cast onto the 1 cm2 surface of the nickel foam electrode (NF) with a mass loading of about 0.1 mg cm−2 and dried under the infra-red lamp and used as a working electrode. The Pt wire and Ag/AgCl filled with 3 M KCl electrolyte were used as counter and reference electrodes, respectively. The linear sweep voltammetry (LSV) scans were acquired at a scan rate of 1 mV s−1 in the potential window of 0 V to 0.8 V vs. Ag/AgCl. The CV curves at the various scan rates (40, 60, 80, 100, and 120 mV s−1) were recorded in the potential window of −0.01 to 0.05 V vs. Ag/AgCl before the redox reaction occurs to estimate the double-layer capacitance (Cdl) and the electrochemically active surface area (ECSA). The obtained potentials were calculated with respect to the reversible hydrogen electrode (RHE) by using the Nernst equation: ERHE = E0 + (0.059 × pH).
3. Results and discussion
Fig. 1 depicts the fabrication method of the NiCo2O4 sample. The NiCo2O4 is synthesized with the facile solvothermal method in which cationic surfactant (CTAB) plays an important role. As given in the Experimental section, a 1
:
1 ratio of cobalt nitrate and nickel nitrate was used for the synthesis of NiCo2O4 composition. Initially, with increasing temperature, urea decomposes and produces OH− ion. The Co2+ and Ni2+ ions react with hydroxide ions which are liberated from urea and generate a Ni–Co hydroxide composite. Next, the Ni–Co hydroxide composite gets arranged in a teddy bear sunflower-like spherical structure wherein several nano spines grown from the center could be observed.41 Lastly, after the annealing process, hydroxides of metal decompose completely and form crystalline NiCo2O4 metal oxide. It can be seen from Fig. 1 that a well-defined hierarchical teddy bear sunflower structure of NiCo2O4 catalyst is obtained. The surfactant (CTAB) plays a significant role in the growth mechanism of such spherical structures along with reaction parameters.42–44 The cationic surfactant CTAB contains a hydrophilic polar head and a hydrophobic tail giving rise to the spherical micellar structure. During the reaction, the inorganic anions from the cobalt salt and nickel salt intrinsically adsorbed on the surfactant and thus directed the final morphology. The final shape of the catalyst depends on the exact conditions of the synthesis. Consequently, a combination of different metal precursors and CTAB formulates diverse morphologies. The NiO and Co3O4 have followed a similar mechanism in forming spherical and sea-urchin-like structures, respectively.
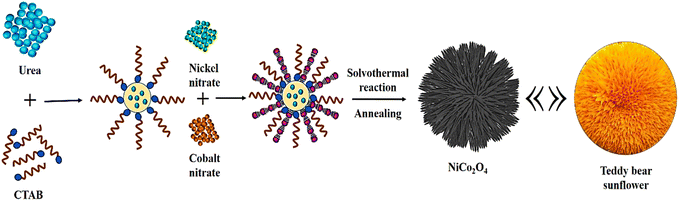 |
| Fig. 1 Schematic representation for the synthesis of hierarchical teddy bear sunflower-like NiCo2O4 catalyst. | |
The phase identification and the structure investigation of the prepared NiCo2O4, NiO, and Co3O4 samples were carried out using X-ray powder diffraction (XRD) analysis (Fig. 2). The prepared NiCo2O4 and Co3O4 samples exhibit FCC cubic spinel structure. The observed diffraction peaks match well with the JCPDS file no. 20-0781 for NiCo2O4 and JCPDS, no. 42-1467 for Co3O4.30,45 The smallest average crystallite size was obtained for NiCo2O4 (5.4 nm), which was determined by the Debye–Scherrer equation (Table S1†). The XRD pattern of the NiO sample show peaks indexed to the pure phase of FCC NiO (JCPDS file no. 78-0643).46 The cubic lattice constants for NiCo2O4, Co3O4, and NiO samples (Table S1†) are obtained from high-intensity peaks and are in accordance with the theoretical values indicating high phase purity of all the prepared samples.47
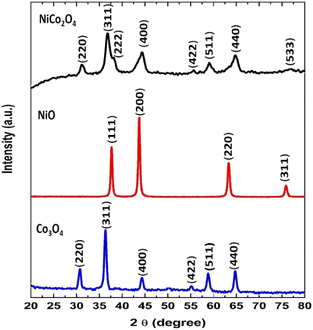 |
| Fig. 2 Indexed powder XRD patterns of NiCo2O4, NiO and Co3O4 samples. | |
The surface morphology of the NiCo2O4 catalyst was investigated by FE-SEM, displayed in Fig. 3(a)–(c). From low-magnification FE-SEM images (Fig. 3(a) and (b)), we can observe that the prepared NiCo2O4 sample displays uniform microspheres (4 μm diameter). The high-magnification FE-SEM image (Fig. 3(c)) depicts that these microspheres are composed of several nano spines with a length of 1 μm. The TEM image of the NiCo2O4 sample (Fig. 3(d)) agrees well with the obtained FE-SEM image. The nano spines in the NiCo2O4 are made up of numerous nanoparticles with diameters of 14–18 nm and resemble teddy bear sunflowers, as revealed by high-magnification TEM images (Fig. 3(e) and (f)). These nanoparticles interconnect and form the porous teddy bear sunflower-like structure that exhibits a high specific surface area. Such porous structures may adsorb enough oxygen and offer more active sites.48Fig. 3(g) displays the HRTEM image of encircled region of Fig. 3(f). The enlarged view (Fig. 3(h)) reveals the lattice plane with a spacing of 0.25 nm attributed to the (3 1 1) plane of the NiCo2O4 phase.49 The inset of Fig. 3(h) depicts the SAED pattern of the hierarchical teddy bear sunflower-like NiCo2O4 catalyst, which indicates the polycrystalline nature of the prepared catalyst and could be indexed to the spinel phase of NiCo2O4. The STEM-EDS spectra and mapping of the NiCo2O4 shows the even distribution of the Ni, Co, and O elements on the surface, shown in Fig. 3(i) and (j), respectively. FE-SEM images of NiO and Co3O4 samples exhibit spherical and sea-urchin-like morphology, which are displayed in Fig. S1(a) and (b).†
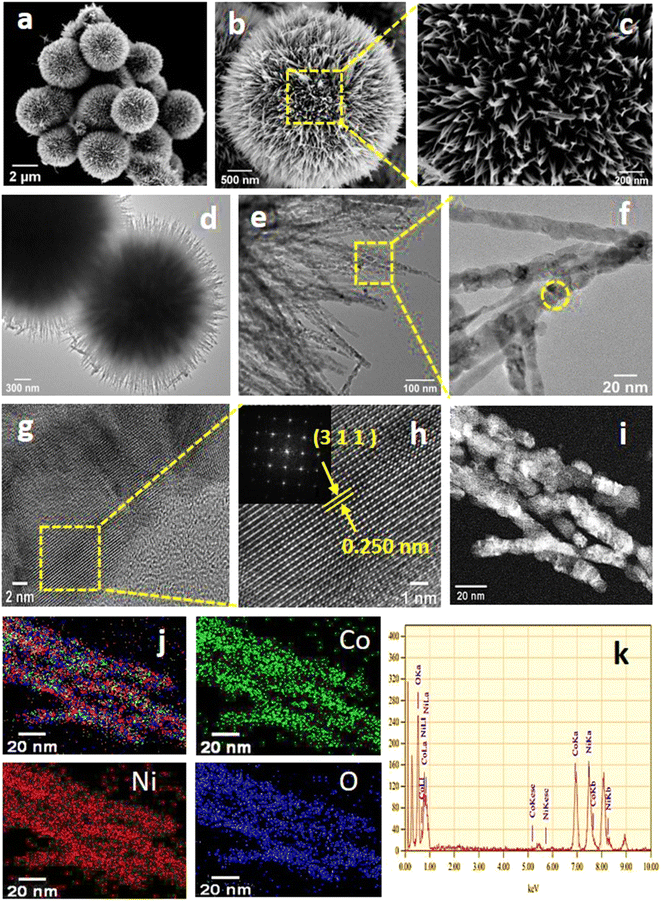 |
| Fig. 3 Low and high magnification FE-SEM images of hierarchical teddy bear sunflower-like NiCo2O4 (a–c, respectively). Low and high magnification TEM images of NiCo2O4 (d–f, respectively). HR-TEM (g and h), and STEM image (i), respectively. STEM-elemental mapping of Co, Ni, and O with an overlay of all three elements (j). EDX of NiCo2O4 (k). | |
The oxidation states and elemental composition of hierarchical teddy bear sunflower-like NiCo2O4, spherical NiO, and sea-urchin-like Co3O4 samples were investigated using the X-ray photoelectron spectroscopy technique (Fig. 4 and S2(a)–(f)†) and the analysis data is shown in Table S2.† The presence of only Ni, Co, and O elements in the survey spectrum (Fig. 4(a)) of the hierarchical teddy bear sunflower-like NiCo2O4 sample is in line with the STEM-EDX spectrum (Fig. 3(k)). The deconvoluted (Gaussian) Co 2p3/2, Ni 2p3/2, and O 1s XPS spectrum are shown in Fig. 4(b)–(d), respectively. The high-resolution Co 2p3/2 spectrum in Fig. 4(b) establishes that spin-doublets were attributed to Co3+ and Co2+.50 These characteristic peaks are also accompanied by Co 2p3/2 satellite peaks. Fig. 4(c) shows the high-resolution Ni 2p3/2 XPS spectrum. The binding energy peaks attributed to the Ni3+ 2p3/2 and Ni2+ 2p3/2, respectively.50,51 These oxidation states of Ni and Co in NiCo2O4 are compared with the Ni 2p and Co 2p3/2 line shapes from NiO and Co3O4 samples (Fig. S2(b) and (e)†, respectively) and it is observed that both Ni 2p3/2 and Co 2p3/2 in NiCo2O4 sample exhibit a small upward shift of 0.3 eV and extra-large shift of 1.1 eV towards higher binding energy, respectively indicates the significant electron donors adjacent to Co. A remarkable increase in the intensity ratio for Ni3+/Ni2+ was observed in NiCo2O4 (Fig. 4(c)) as compared to NiO (Fig. S2(b)†). Whereas no such change is detected in the case of Co oxidation states when compared to Co3O4. This directs that a large amount of Ni2+ ions were oxidized to Ni3+. The XPS spectrum for O 1s can be deconvoluted into three peaks which can be related to the lattice oxygen (OI), surface absorbed hydroxyl (OII), and surface C–O(OIII), respectively (Fig. 4(d)).52–54 The peak that appeared in the O 1s state of NiCo2O4 at 530.98 eV was ascribed to the coordination of oxygen–Ni2+, signifying the maximum Ni2+ content on the surface of the hierarchical teddy bear sunflower-like NiCo2O4 catalyst.54 Thus, the existence of high valence states of the metal cations as well as oxygen adsorption species endows the hierarchical teddy bear sunflower-like NiCo2O4 catalyst with greater electrical conductivity and rapid charge transport for water splitting.
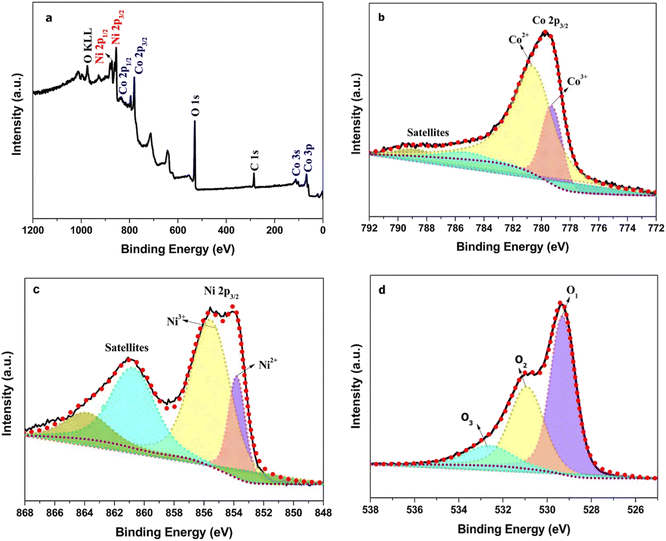 |
| Fig. 4 XPS survey scan of hierarchical teddy bear sunflower NiCo2O4 catalyst (a), high-resolution XPS spectra for Co 2p, Ni 2p, and O 1s states in NiCo2O4 (b–d, respectively). | |
Brunauer–Emmett–Teller (BET) study was performed for the hierarchical teddy bear sunflower-like NiCo2O4, spherical NiO, and sea-urchin-like Co3O4 samples to examine the surface area, shown in Fig. S3.† BET surface area of NiCo2O4 and NiO and Co3O4 catalysts were measured to be 100.79 m2 g−1, 68.53 m2 g−1, and 59.76 m2 g−1, respectively. The marginal difference in surface areas and morphologies suggests the large variety of surface areas contributes more to enhancing OER activities in NiCo2O4 and NiO and Co3O4 catalysts discussed in the following. The prominent hysteresis of type IV H3 was observed for the hierarchical teddy bear sunflower-like NiCo2O4 sample, demonstrated by BET adsorption–desorption. It is well established that the larger specific surface area of the catalyst provides more electroactive sites for the faradaic reactions.55,56 This high surface area of NiCo2O4 and well-defined nanoparticles can promote water oxidation performance effectively.
4. DFT calculation for density of states (DOS)
Before directly measuring the water oxidation performance of all three samples, we carried out a DFT calculation to gain the DOS statistics at the surface of each sample. To understand the contribution of different orbitals in catalyzing the OER process, we analyze the projected density of states (pDOS). A series of pDOS plots with up and down spins for NiCo2O4, NiO, and Co3O4 materials are shown in Fig. S4(a)–(d).† The partial and total DOS for NiCo2O4, NiO, and Co3O4 materials are shown in Fig. 5(a)–(c). We observed that a major contribution to the up-channel comes from the p orbitals of the O atom followed by the d-orbital of the Co atom in Co3O4 (Fig. S4(d)†). The contribution of O-p up-spin orbitals is almost twice that of Co-d up-spin orbitals. In NiO no significant contribution from the up-channel was detected (Fig. S4(c)†).
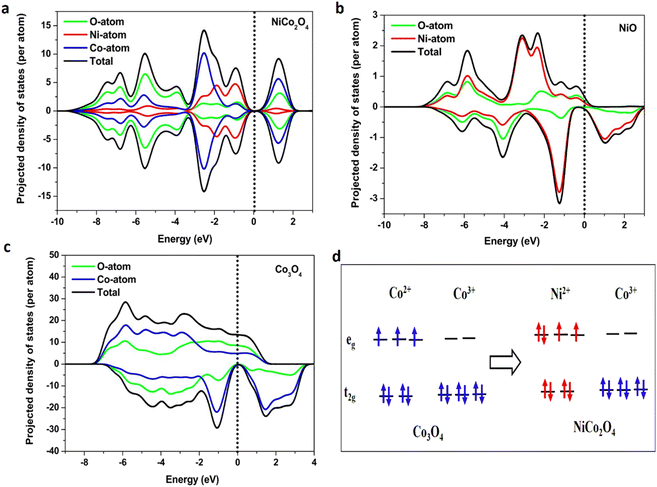 |
| Fig. 5 The partial and total projected density of states for NiCo2O4 (a), NiO (b), and Co3O4 (c), respectively, calculated using density functional theory (DFT)+U, (d) schematic diagram of the spin-states in the Co3O4 in the left and NiCo2O4 in the right. | |
In NiCo2O4, the up spin and down spin of each atom contribute equally to the entire system (Fig. 5(a)), indicating the composite effect in the electronic system. The 3d-orbitals from Ni atoms show a higher density of states near the Fermi level (Ef) than Co-2d (Fig. S4(a)†) in NiCo2O4. Our calculation shows that DOS around Ef is dominated by the Ni2+ cations occupying the tetrahedral sites. Fig. 5(d) shows the predicted electron occupation in d orbitals for the spinel Co3O4 and NiCo2O4 systems based on the oxidation states. The new electronic state is present in the conduction band of NiCo2O4 (Fig. 5(d)), indicating that more electrons are available to participate in the catalytic process. The electronic states prediction is compared with the literature, which matches our theoretical calculations and experimental results.47,57 The d-orbital occupancy integrated from the DOS results is presented in Table S3,† which is close to our prediction of electronic configuration. Hence, we postulate that the Ni atom has a major contribution to catalytic activity, whereas the Co atom is responsible for conductivity in NiCo2O4 material.
5. Water oxidation performance
The fabricated hierarchical teddy bear sunflower-like NiCo2O4, spherical NiO, and sea-urchin-like Co3O4 catalysts supported on nickel foam were subjected to electrochemical water oxidation performance through OER in 1 M KOH alkaline electrolyte as shown in Fig. 6. The OER performances of the NiCo2O4/NF, NiO/NF, and Co3O4/NF are evaluated by LSV polarization curves (Fig. 6(a)) at a scan rate of 1 mV s−1 after 15 cycles of CV measurement. The superior OER performance was observed for the hierarchical teddy bear sunflower-like NiCo2O4/NF catalyst (η10 = 290 mV) as compared to the spherical NiO/NF (η10 = 300 mV), the sea-urchin-like Co3O4/NF (η10 = 322 mV), and commercial RuO2 (η10 = 335 mV) to achieve a current density of 10 mA cm−2. The overpotential values with respect to different current densities (10 mA cm−2, 50 mA cm−2, and 200 mA cm−2) for NiCo2O4/NF, NiO/NF, and Co3O4/NF catalysts are plotted and presented in Fig. 6(b). The enhanced catalytic activity of NiCo2O4/NF catalyst relative to NiO/NF and Co3O4/NF is attributed to the porous hierarchical nanostructure exhibited by NiCo2O4/NF, which could provide high surface area and abundant active sites also facilitating the fast ion transport kinetics. Concerning the impinge of specific surface area on the intrinsic activity, BET surface area normalized LSV was plotted (Fig. S5†). The observed OER activity trend was similar to that of LSV concerning the geometrical surface area. Furthermore, as shown in Fig. 6(c), the hierarchical teddy bear sunflower-like NiCo2O4/NF catalyst exhibited the ultralow Tafel slope value of 37 mV dec−1, which is substantially smaller than the spherical NiO/NF (52 mV dec−1) and the sea-urchin like Co3O4/NF (96 mV dec−1). The low Tafel slope value in the case of the hierarchical teddy bear sunflower-like NiCo2O4/NF catalyst indicates the facile charge transport at the interface.
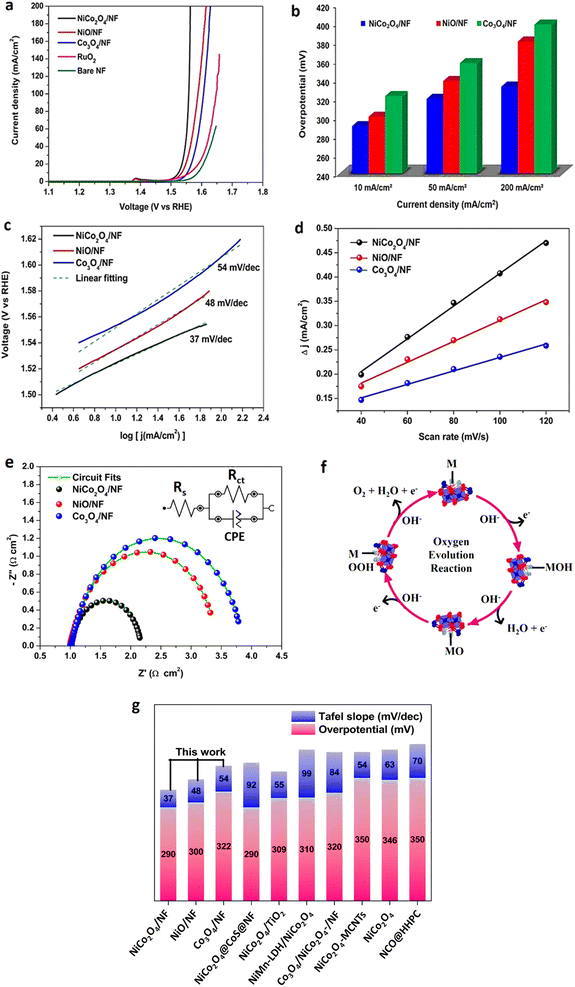 |
| Fig. 6 (a) Linear polarization curves at a scan rate of 1 mV s−1 NiCo2O4/NF, NiO/NF, Co3O4/NF, RuO2, and bare NF catalysts for OER. (b) Overpotential vs. current density plot of NiCo2O4/NF, NiO/NF, and Co3O4/NF catalysts. (c) Tafel plots of NiCo2O4/NF, NiO/NF, and Co3O4/NF. (d) Plot representing the relationship between the difference in the anodic and cathodic current densities and scan rate. The slope of the fitted line is the double-layer capacitance (Cdl) of NiCo2O4/NF, NiO/NF, and Co3O4/NF catalysts. (e) Electrochemical impedance spectra (Nyquist curves) of NiCo2O4/NF, NiO/NF, and Co3O4/NF catalysts; the inset shows the equivalent circuit for the data. (f) Schematic of the four-step reaction pathway for OER in alkaline electrolyte. (g) Comparison of the overpotential (η10) and Tafel slopes for the catalyst prepared in this work and recently reported NiCo2O4. | |
The electrochemical active surface area (ECSA) of electrocatalysts plays an imperative role in evaluating the activity of the catalyst.58 The ECSA was evaluated by dividing the obtained double-layer capacitance (Cdl) value by the specific capacitance (Cs) of the catalyst. To determine the Cdl of all three catalysts, CVs were obtained in the non-faradaic potential region at different scan rates (Fig. S6†). As shown in Table S4,† it illustrates that the Cdl of the hierarchical teddy bear sunflower-like NiCo2O4/NF, spherical NiO/NF, and sea-urchin-like Co3O4/NF catalysts are 1.68, 1.07, and 0.69 mF cm−2, respectively and presented in Fig. 6(d). The Cs value of 26 μF cm−2 and 25 μF cm−2 and 27 μF cm−2 are used to calculate the ECSA of hierarchical teddy bear sunflower-like NiCo2O4/NF, spherical NiO/NF, and sea-urchin Co3O4/NF catalysts.59 The higher ECSA of 64.61 cm2 for hierarchical teddy bear sunflower-like NiCo2O4 catalyst compared to spherical NiO/NF (42.8 cm2), and sea-urchin Co3O4/NF (25.55 cm2) catalysts confirms the close correlation between active sites and surface morphology. The electrochemical impedance spectroscopy (EIS) was conducted at a potential of 1.536 V vs. RHE in the frequency range of 0.01–100 kHz for all three catalysts to extract the charge transfer kinetics at the electrode–electrolyte interface. The Nyquist plots and the fitted equivalent circuit composed of a charge transfer resistance (Rct), electrolyte resistance (Rs), and an electrical double-layer capacitance (Cdl) are shown in Fig. 6(e) and inset of Fig. 6(e), respectively. The capacitance and resistance values are calculated and tabulated in Table S4.† The low Rct value of 1.15 Ω cm2 for hierarchical teddy bear sunflower-like NiCo2O4 compared to the other catalysts suggests a significantly higher charge-transfer kinetics resulting in enhanced OER performance.
The redox reactions for the hierarchical teddy bear sunflower-like NiCo2O4/NF, spherical NiO/NF, and sea-urchin-like Co3O4/NF catalysts were investigated to understand the surface reactivity by employing CVs between 1.036 to 1.836 V vs. RHE at a sweep rate of 2 mV s−1 in 1 M KOH (Fig. S7†). A pair of redox peaks were noticed in all three catalysts. The redox peaks are principally ascribed to the formation of MO/MOOH (M denotes Co/Ni/NiCo ions) reaction intermediate in the faradaic process.60,61 The occurrence of a single anodic oxidation peak at 1.38 V vs. RHE for both NiCo2O4/NF and NiO/NF catalysts attributed to the Ni2+/Ni3+ transition, confirming that a large amount of Ni2+ ions were oxidized to Ni3+ ions which is consistent with the XPS analysis.62 The sharp cathodic peaks at 1.29 V vs. RHE are observed for NiO/NF and NiCo2O4/NF catalysts, respectively.63 Moreover, both the surface analysis techniques (XPS and CV) revealed that the Ni cations are present in large amounts on the surface of NiCo2O4/NF catalyst compared to Co cations demonstrating that the Ni cations are mainly responsible for enhanced OER performance by forming NiOOH as a reaction intermediate and provide more active sites.50,54
Another influential parameter for catalyst performance evaluation is mass activity (MA). To eradicate the impact of mass loading, MA was determined by normalizing the current density with the mass loading of the material on the surface of the electrode.64 The MA values of 140 A g−1, 95 A g−1, and 44 A g−1 are obtained at a potential of 1.53 V vs. RHE for the hierarchical teddy bear sunflower-like NiCo2O4/NF, spherical NiO/NF, and sea-urchin-like Co3O4/NF catalyst, respectively. Manifestly, the trend is in good agreement with that of overpotential values for all three catalysts. Higher specific and mass activities of the hierarchical teddy bear sunflower-like NiCo2O4/NF catalyst reflect the higher electrocatalytic activity as compared to the other two catalysts (NiO/NF and Co3O4/NF). To further evaluate the efficiency of prepared catalysts, turnover frequency (TOF) values which is the measure of intrinsic activity were determined.65 Considering that all of the available surface metal atoms are catalytically active, TOF at a fixed potential of 1.53 V vs. RHE was determined as 0.61 s−1, 0.26 s−1, and 0.18 s−1 for the NiCo2O4/NF, NiO/NF, and Co3O4/NF catalysts, respectively, further suggesting that the hierarchical teddy bear sunflower-like NiCo2O4/NF is indeed an efficient and potent catalyst.
5.1 Correlation of the OER activity with electronic states in different structures
Herein, the theoretical calculations and experimental study complement each other for the study of OER mechanisms from the perspective of thermodynamics and kinetics. The water oxidation reaction in an alkaline solution is expressed as; | 4OH− → O2(g) + 2H2O(l) + 4e− | (1) |
This specific water oxidation process of OER can be described in terms of the adsorbate evolution mechanism (AEM) with four elementary steps as follows (Fig. 6(f));66
| MOH + OH− → MO + H2O + e− | (3) |
| MOOH + OH− → MO2 + H2O + e− | (5) |
where MOH, MO and MOOH represent adsorbed reaction intermediates with metal surface site M. Notably, the XPS and slow scan CV investigation provide evidence for the existence of more active Ni
3+ cations than Co on the surface of NiCo
2O
4 to trigger the formation of NiOOH which was critical for enhancing OER. Certainly, the above-analyzed DFT results manifest that the morphology of the material has a considerable effect on the electronic structure near the
Ef of the electrocatalysts. Co
3O
4 has a normal spinel structure, where Co
2+ is located at tetrahedral (
Td) sites and Co
3+ at (
Oh) sites; however, NiCo
2O
4 has an inverse spinel structure, where preferably Ni
2+ occupies
Oh sites and Co
3+ occupies both
Oh and
Td sites. Nevertheless, Co
3+ at
Td sites is thermodynamically unstable and likely to convert to a more stable Co
2+ state. This results in the oxidation of a similar amount of Ni
2+ to Ni
3+ to sustain charge neutrality. Therefore, the electronic state induced by morphology is the key to describing enhanced OER activity in NiCo
2O
4. According to the Gerischer–Marcus model of charge transfer, orbital overlapping occurs due to the high DOS near the
Ef which then could adsorb OH
− species and reduce the energy to accept or donate electrons at the adsorbate–catalyst interface reaction.
67 As mentioned above, 3D nanostructure morphology in NiCo
2O
4 exhibits far more active sites and could adsorb higher amounts of OH
− species, also providing a sufficient conduction path to prevent serious ohmic losses demonstrated by EIS investigations and adsorption isotherms. We, therefore, plot the ECSA-corrected LSV curves for all three electrocatalysts (Fig. S8
†). It is illustrated that porous nanostructure fairly enhances the intrinsic catalytic activity of NiCo
2O
4/NF. Moreover, the higher intrinsic activity with large electron transfer has been witnessed by catalyst utilization measurement. Catalyst utilization is as the ECSA of the active catalyst divided by the BET surface area of the catalyst (see ESI eqn (2)
†). The high efficiency of catalyst utilization (64.28%) in NiCo
2O
4/NF is attributed to the higher adsorption of OH
− ions and better charge conductivity at the electrode interface (Table S5
†).
Of note, all the aforementioned OER activity evaluation parameters, including electroactive surface area, charge transfer resistance, electrical conductivity, catalyst utilization, and TOF (Table 1) values suggest that the NiCo2O4/NF exhibiting teddy-bear sunflower-like morphology is highly active for the water oxidation process compared to spherical NiO/NF and sea-urchin like Co3O4/NF. Such hierarchical nanostructure unveiled by NiCo2O4/NF achieves high current density at low overpotential and delivers high intrinsic activity owing to facile electrolyte penetration along with the prominently high density of Ni 3d states near the Fermi level. Fig. 6(g) and Table S6† show the comparison of overpotential values at a current density of 10 mA cm−2 and Tafel slope for recently reported nickel and cobalt-based bimetallic catalysts.68–74 It is noteworthy that, the fabricated hierarchical teddy-bear sunflower-like NiCo2O4/NF in this work is the potential low-cost candidate as an OER catalyst for practical applicability in the water-splitting process.
Table 1 Summary of catalytic performances for the prepared NiCo2O4/NF, NiO/NF, and Co3O4/NF electrocatalysts
Electrocatalyst |
Overpotential (mV) at 10 mA cm−2 |
Tafel slope mV dec−1 |
ECSA m2 g−1 |
BET surface area m2 g−1 |
Mass activity (mA mg−1) |
TOF s−1 |
NiCo2O4/NF |
290 |
37 |
64.61 |
100.79 |
140 |
0.61 |
NiO/NF |
300 |
48 |
42.40 |
68.53 |
95 |
0.26 |
Co3O4/NF |
322 |
54 |
25.55 |
59.76 |
44 |
0.18 |
In addition to catalytic efficiency, stability is another important parameter for an OER catalyst. To confirm the stability of the hierarchical teddy bear sunflower-like NiCo2O4/NF catalyst, chronoamperometry measurement was carried out at 1.55 V vs. RHE for 16 h, shown in Fig. 7(a). It clearly indicates that the hierarchical teddy bear sunflower-like NiCo2O4/NF catalyst is highly stable in 1 M KOH. The LSV at 1 mV s−1 for the catalyst was recorded after stability testing and compared with the initial LSV (before stability), shown in Fig. 7(b). The hierarchical teddy bear sunflower-like NiCo2O4/NF catalyst displayed similar activity even after 16 h (Fig. 7(b)). The morphology of the hierarchical teddy bear sunflower-like NiCo2O4/NF catalyst after the OER operation was shown in Fig. S9(a)–(d).† The characteristic teddy bear sunflower-like structure was preserved even after 16 h, demonstrating the practical electrocatalytic durability of the product.
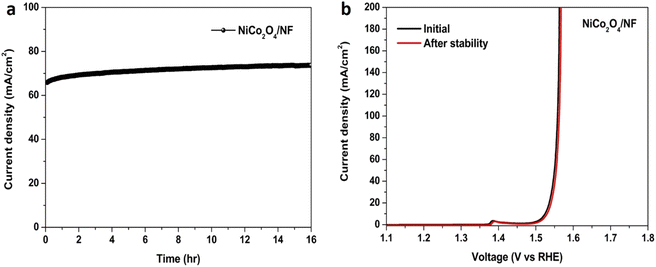 |
| Fig. 7 The chronoamperometric curve at 1.55 V vs. RHE of NiCo2O4/NF catalyst (a), LSV curves of NiCo2O4/NF catalyst before and after stability for OER in 1 M KOH at a scan rate of 1 mV s−1 (b). | |
6. Conclusions
In summary, we report the fabrication of the hieratical teddy bear sunflower-like NiCo2O4, spherical NiO/NF, and sea-urchin-like Co3O4 using a facile solvothermal and annealing route. A comparative investigation of all three catalysts supported on nickel foam for water oxidation through OER analysis was performed. The hierarchical teddy bear sunflower-like NiCo2O4/NF catalyst has displayed superior OER activity with a low water oxidation overpotential of 290 mV and 330 mV at respectively 10 mA cm−2 and 200 mA cm−2 current densities. The enhanced OER performance of the hieratical teddy bear sunflower-like NiCo2O4 catalyst was mainly attributed to the large specific and active surface area, high catalyst utilization, and high intrinsic activity (TOF). We also investigated the intrinsic contribution of the 3d orbital states of Ni and Co atoms to the OER activity of NiCo2O4. The practical utilization of the hieratical teddy bear sunflower-like NiCo2O4/NF was probed through the stability testing of the material. The stable electrochemical performance and sustained morphology, even after prolonged cycling, indicate that the catalyst is highly suitable for the large-scale water oxidation reaction. This work provides a systematic analysis of the structural and electrochemical performance supported by DFT calculation of the prepared catalyst toward their OER counterparts.
Conflicts of interest
There are no conflicts of interest to declare.
Acknowledgements
This work was supported by the Department of Science and Technology-WOS-A scheme (SR/WOS-A/PM-9/2018(G)).
References
- S. Chu and A. Majumdar, Nature, 2012, 488, 294–303 CrossRef CAS PubMed.
- I. C. Man, H. Y. Su, F. Calle-Vallejo, H. A. Hansen, J. I. Martínez, N. G. Inoglu, J. Kitchin, T. F. Jaramillo, J. K. Nørskov and J. Rossmeisl, ChemCatChem, 2011, 3, 1159–1165 CrossRef CAS.
- M. W. Kanan and D. G. Nocera, Science, 2008, 321, 1072–1075 CrossRef CAS PubMed.
- Y. Lee, J. Suntivich, K. J. May, E. E. Perry and Y. Shao-Horn, J. Phys. Chem. Lett., 2012, 3, 399–404 CrossRef CAS PubMed.
- T. Audichon, T. W. Napporn, C. Canaff, C. u. Morais, C. m. Comminges and K. B. Kokoh, J. Phys. Chem. C, 2016, 120, 2562–2573 CrossRef CAS.
- Y. Liu, H. Jiang, Y. Zhu, X. Yang and C. Li, J. Mater. Chem. A, 2016, 4, 1694–1701 RSC.
- K. Zhang and R. Zou, Small, 2021, 2100129 CrossRef CAS PubMed.
- P. M. Bodhankar, P. B. Sarawade, G. Singh, A. Vinu and D. S. Dhawale, J. Mater. Chem. A, 2021, 9, 3180–3208 RSC.
- P. M. Bodhankar, P. B. Sarawade, P. Kumar, A. Vinu, A. P. Kulkarni, C. D. Lokhande and D. S. Dhawale, Small, 2022, 2107572 CrossRef CAS PubMed.
- S. Anantharaj, S. R. Ede, K. Sakthikumar, K. Karthick, S. Mishra and S. Kundu, ACS Catal., 2016, 6, 8069–8097 CrossRef CAS.
- Z. Peng, D. Jia, A. M. Al-Enzi, A. A. Elzatahry and G. Zheng, Adv. Energy Mater., 2015, 5, 1402031 CrossRef.
- D. S. Dhawale, P. Bodhankar, N. Sonawane and P. B. Sarawade, Sustainable Energy Fuels, 2019, 3, 1713–1719 RSC.
- Y. Gao, D. Zheng, Q. Li, W. Xiao, T. Ma, Y. Fu, Z. Wu and L. Wang, Adv. Funct. Mater., 2022, 32, 2203206 CrossRef CAS.
- X. Bo, Y. Zhang, M. Li, A. Nsabimana and L. Guo, J. Power Sources, 2015, 288, 1–8 CrossRef CAS.
- Y. Zhang, J. Yang, Z. Yu, Y. Hou, R. Jiang, J. Huang, F. Yang, S. Yao, L. Gao and W. Tang, Chem. Eng. J., 2021, 416, 129124 CrossRef CAS.
- J. Liang, Y.-Z. Wang, C.-C. Wang and S.-Y. Lu, J. Mater. Chem. A, 2016, 4, 9797–9806 RSC.
- K. Artyushkova, S. Pylypenko, M. Dowlapalli and P. Atanassov, J. Power Sources, 2012, 214, 303–313 CrossRef CAS.
- D. G. Lee, O. Gwon, H. S. Park, S. H. Kim, J. Yang, S. K. Kwak, G. Kim and H. K. Song, Angew. Chem., 2015, 127, 15956–15959 CrossRef.
- M. Luo, M. Xuan, S. Huo, J. Fan, G. Chakraborty, Y. Wang, H. Zhao, A. Herrmann and L. Zheng, Angew. Chem., Int. Ed., 2020, 59, 17250–17255 CrossRef CAS PubMed.
- Y. Wang, Z. Chen, Q. Li, X. Wang, W. Xiao, Y. Fu, G. Xu, B. Li, Z. Li, Z. Wu and L. Wang, Nano Res., 2022 DOI:10.1007/s12274-022-4980-4.
- Y. Wang, Q. Sun, Z. Wang, W. Xiao, Y. Fu, T. Ma, Z. Wu and L. Wang, J. Mater. Chem. A, 2022, 10, 16236–16242 RSC.
- W. Yu, Z. Chen, W. Xiao, Y. Chai, B. Dong, Z. Wu and L. Wang, Inorg. Chem. Front., 2022, 9, 1847–1855 RSC.
- Z. Wang, Y. Wang, W. Xiao, X. Wang, Y. Fu, G. Xu, Z. Li, Z. Wu and L. Wang, J. Mater. Chem. A, 2022, 10, 15155–15160 RSC.
- X. Gao, H. Zhang, Q. Li, X. Yu, Z. Hong, X. Zhang, C. Liang and Z. Lin, Angew. Chem., Int. Ed., 2016, 55, 6290–6294 CrossRef CAS PubMed.
- X. Gao, G. Li, Y. Xu, Z. Hong, C. Liang and Z. Lin, Angew. Chem., 2015, 127, 14539–14543 CrossRef.
- R. W. Grimes, A. B. Anderson and A. H. Heuer, J. Am. Chem. Soc., 1989, 111, 1–7 CrossRef CAS.
- C. Guan, X. Liu, W. Ren, X. Li, C. Cheng and J. Wang, Adv. Energy Mater., 2017, 7, 1602391 CrossRef.
- A. Rashti, X. Lu, A. Dobson, E. Hassani, F. Feyzbar-Khalkhali-Nejad, K. He and T.-S. Oh, ACS Appl. Energy Mater., 2021, 4, 1537–1547 CrossRef CAS.
- Z. Wang, W. Xu, Y. Wang, X. Cui, A. M. Al-Enzi, Y. Tang and G. Zheng, J. Mater. Chem. A, 2017, 5, 7416–7422 RSC.
- X. Chen, L. Luo and Y. Zhang, Langmuir, 2021, 37, 6330–6336 CrossRef CAS PubMed.
- C. Alegre, C. Busacca, A. Di Blasi, O. Di Blasi, A. S. Aricò, V. Antonucci and V. Baglio, ChemElectroChem, 2020, 7, 124–130 CrossRef CAS.
- M. Prabu, K. Ketpang and S. Shanmugam, Nanoscale, 2014, 6, 3173–3181 RSC.
- X. Liu, Z.-Y. Zhai, Z. Chen, L.-Z. Zhang, X.-F. Zhao, F.-Z. Si and J.-H. Li, Catalysts, 2018, 8, 310 CrossRef.
- P. Bodhankar, A. Chunduri, N. Patel, D. S. Dhawale, A. Vinu, H. Johani and P. B. Sarawade, Sustainable Energy Fuels, 2021, 5, 1120–1128 RSC.
- P. M. Bodhankar, S. Jadhav and P. B. Sarawade, Macromol. Symp., 2021, 400, 2100144 CrossRef CAS.
- P. Giannozzi, S. Baroni, N. Bonini, M. Calandra, R. Car, C. Cavazzoni, D. Ceresoli, G. L. Chiarotti, M. Cococcioni and I. Dabo, J. Phys.: Condens. Matter, 2009, 21, 395502 CrossRef PubMed.
- P. Giannozzi, O. Andreussi, T. Brumme, O. Bunau, M. B. Nardelli, M. Calandra, R. Car, C. Cavazzoni, D. Ceresoli and M. Cococcioni, J. Phys.: Condens. Matter, 2017, 29, 465901 CrossRef CAS PubMed.
- J. Tao, J. P. Perdew, H. Tang and C. Shahi, J. Chem. Phys., 2018, 148, 074110 CrossRef PubMed.
- S. L. Dudarev, G. A. Botton, S. Y. Savrasov, C. Humphreys and A. P. Sutton, Phys. Rev. B: Condens. Matter Mater. Phys., 1998, 57, 1505 CrossRef CAS.
- S. Curtarolo, W. Setyawan, G. L. W. Hart, M. Jahnatek, R. V. Chepulskii, R. H. Taylor, S. Wang, J. Xue, K. Yang, O. Levy, M. J. Mehl, H. T. Stokes, D. O. Demchenko and D. Morgan, Comput. Mater. Sci., 2012, 58, 218–226 CrossRef CAS.
- P. Nayak, M. Sahoo and S. K. Nayak, Ceram. Int., 2020, 46, 3818–3826 CrossRef CAS.
- Y. K. Mishra, S. Kaps, A. Schuchardt, I. Paulowicz, X. Jin, D. Gedamu, S. Freitag, M. Claus, S. Wille and A. Kovalev, Part. Part. Syst. Charact., 2013, 30, 775–783 CrossRef CAS.
- S. K. Meher, P. Justin and G. Ranga Rao, ACS Appl. Mater. Interfaces, 2011, 3, 2063–2073 CrossRef CAS PubMed.
- M.-P. Pileni, Nat. Mater., 2003, 2, 145–150 CrossRef CAS PubMed.
- T. E. Kibona, J. Solid State Electrochem., 2020, 24, 1587–1598 CrossRef CAS.
- Z. Zhu, N. Wei, H. Liu and Z. He, Adv. Powder Technol., 2011, 22, 422–426 CrossRef CAS.
- T.-C. Chang, Y.-T. Lu, C.-H. Lee, J. K. Gupta, L. J. Hardwick, C.-C. Hu and H.-Y. T. Chen, ACS Omega, 2021, 6, 9692–9699 CrossRef CAS PubMed.
- E. Umeshbabu, G. Rajeshkhanna, P. Justin and G. R. Rao, Mater. Chem. Phys., 2015, 165, 235–244 CrossRef CAS.
- B. Wang, C.-W. Tsang, K. H. Li, Y. Tang, Y. Mao and X.-Y. Lu, Nanoscale Res. Lett., 2019, 14, 6 CrossRef PubMed.
- L. Yang, B. Zhang, B. Fang and L. Feng, Chem. Commun., 2018, 54, 13151–13154 RSC.
- L. Zhang, Y. Li, J. Peng and K. Peng, Electrochim. Acta, 2019, 318, 762–769 CrossRef CAS.
- M. Cheng, H. Fan, Y. Song, Y. Cui and R. Wang, Dalton Trans., 2017, 46, 9201–9209 RSC.
- L. Fang, Z. Jiang, H. Xu, L. Liu, X. Gu and Y. Wang, J. Catal., 2018, 357, 238–246 CrossRef.
- H.-Y. Wang, Y.-Y. Hsu, R. Chen, T.-S. Chan, H. M. Chen and B. Liu, Adv. Energy Mater., 2015, 5, 1500091 CrossRef.
- S. Sun, H. Li and Z. J. Xu, Joule, 2018, 2, 1024–1027 CrossRef.
- P. Acharya, J. Burrow, M. Abolhassani and L. F. Greenlee, ECS Trans., 2018, 85, 81 CrossRef CAS.
- J. Chen, X. Wu and A. Selloni, Phys. Rev. B, 2011, 83, 245204 CrossRef.
- S.-F. Hung, C.-W. Tung, T.-S. Chan and H. M. Chen, CrystEngComm, 2016, 18, 6008–6012 RSC.
- C. C. McCrory, S. Jung, J. C. Peters and T. F. Jaramillo, J. Am. Chem. Soc., 2013, 135, 16977–16987 CrossRef CAS PubMed.
- H. Wang, Q. Gao and L. Jiang, Small, 2011, 7, 2454–2459 CAS.
- Q. Liu, X. Zhang, B. Yang, J. Liu, R. Li, H. Zhang, L. Liu and J. Wang, J. Electrochem. Soc., 2015, 162, E319 CrossRef CAS.
- C. Zhu, S. Fu, D. Du and Y. Lin, Chem.–Eur. J., 2016, 22, 4000–4007 CrossRef CAS PubMed.
- J. Haenen, W. Visscher and E. Barendrecht, J. Electroanal. Chem. Interfacial Electrochem., 1986, 208, 273–296 CrossRef CAS.
- N.-U.-A. Babar and K. S. Joya, ACS Omega, 2020, 5(19), 10641–10650 CrossRef CAS PubMed.
- S. Anantharaj, S. Ede, K. Karthick, S. S. Sankar, K. Sangeetha, P. Karthik and S. Kundu, Energy Environ. Sci., 2018, 11, 744–771 RSC.
- J. Song, C. Wei, Z.-F. Huang, C. Liu, L. Zeng, X. Wang and Z. J. Xu, Chem. Soc. Rev., 2020, 49, 2196–2214 RSC.
- I. Heller, J. Kong, K. A. Williams, C. Dekker and S. G. Lemay, J. Am. Chem. Soc., 2006, 128, 7353–7359 CrossRef CAS PubMed.
- S. Adhikari, Y. Kwon and D.-H. Kim, Chem. Eng. J., 2020, 402, 126192 CrossRef CAS.
- R. Vadakkekara, R. Illathvalappil and S. Kurungot, ChemElectroChem, 2018, 5, 4000–4007 CrossRef CAS.
- M. Yang, W. Lu, R. Jin, X.-C. Liu, S. Song and Y. Xing, ACS Sustainable Chem. Eng., 2019, 7, 12214–12221 CAS.
- L. Yang, L. Chen, D. Yang, X. Yu, H. Xue and L. Feng, J. Power Sources, 2018, 392, 23–32 CrossRef CAS.
- E. Umeshbabu, P. Hari Krishna Charan, P. Justin and G. Ranga Rao, ChemPlusChem, 2020, 85, 183–194 CrossRef CAS.
- S. M. N. Jeghan and G. Lee, Nanotechnology, 2020, 31, 295405 CrossRef CAS PubMed.
- X. Xiao, X. Hu, Y. Liang, G. Zhang, X. Wang, Y. Yan, X. Li, G. Yan and J. Wang, J. Power Sources, 2020, 476, 228684 CrossRef CAS.
|
This journal is © The Royal Society of Chemistry 2022 |