DOI:
10.1039/D2SD00089J
(Tutorial Review)
Sens. Diagn., 2022,
1, 955-967
Ammonia breath analysis
Received
17th May 2022
, Accepted 10th August 2022
First published on 24th August 2022
Abstract
Breath analysis is an important emerging non-invasive tool for the diagnosis and monitoring of diseases because it can provide information about a vast array of biochemical processes occurring in the human body. Out of the thousands of compounds present in a breath sample, ammonia is of particular interest because of its link to a range of gastric, renal, hepatic, and pulmonary conditions. This review provides an introduction to human breath analysis with a focus on ammonia. The main analytical methods for the analysis of ammonia in breath are then covered with a particular focus on chemical sensing methods and their advantages over more established methods, for example those based on mass spectrometry or animal olfaction. The use of chemical sensors for breath analysis is promising because these sensors are small scale, relatively cheap and easy to use, and are therefore suitable for integration into point-of-care breath analysis devices. Although some chemical sensor-based devices for breath analysis are now being commercialised, challenges remain that are related to selectivity, sensitivity, stability, and the influence of humidity on the sensors.
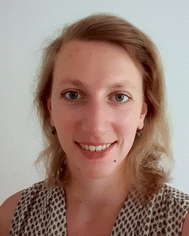 Merel J. Lefferts | Merel Lefferts completed her PhD in Materials Science at the University of Oxford in 2017. She is currently a post-doctoral researcher in Martin Castell's group at the University of Oxford. Her research is focused on creating highly sensitive chemiresistive gas sensors based on percolation networks of conductive polymers. These gas sensors can be used for amongst others ammonia sensing, and have applications in breath analysis as well as safety and security. |
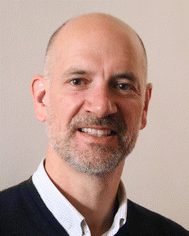 Martin R. Castell | Martin Castell is a Professor in the Department of Materials at the University of Oxford. His current main research activities are the atomic-scale characterisation of surfaces, molecular self-assembly on surfaces, and the development of high-sensitivity chemiresistive gas sensors that operate as electrical percolation networks. He completed his PhD at the University of Cambridge and has held visiting positions at the University of Toronto, Queen's University Belfast, the University of New South Wales, and the University of Canterbury (NZ). |
1. Introduction
Breath analysis is a versatile tool for medical diagnosis and monitoring, performed by analysing the composition of exhaled human breath and the concentrations of both organic and inorganic molecules therein. Breath analysis relies on the gas exchange between blood and air taking place in the alveoli, or air sacs in the lungs. This direct link between various metabolic processes taking place in the human body and the composition of the exhaled breath, makes it possible to study processes and identify diseased states by analysing a breath sample.1 However, it is important to note that although gas exchange in the lungs provides a direct link between blood and air, this is not the only factor that determines the ammonia concentration in the exhaled air. The concentration of mouth exhaled ammonia is higher than what would be expected based on the blood ammonia concentration due to the interaction between the air and the airway surfaces and due to the action of enzymes on urea in the saliva.2,3 Other factors such as temperature, pH, and breathing methods also affect the measured ammonia concentration.4
Other samples that can be analysed to obtain similar information about internal processes and diseased states include blood, saliva, urine, and faeces samples. Compared to breath samples, these methods are more invasive, cumbersome, or can be perceived as embarrassing by the subject or patient. The inherently non-invasive nature of breath analysis is an important advantage over other methods. Sometimes exhaled breath condensate samples, such as components dissolved in exhaled aerosols or in nose or throat mucus,5 are included in the definition of breath samples. Although such samples can provide valuable information, for example to be used for polymerase chain reaction (PCR) tests for the diagnosis of lung carcinoma6 or SARS-COV-2,7 this review will focus on analysis of breath samples in the vapour phase.
Perhaps the most well-known example of breath analysis is an alcohol breath test, or breathalyser, used to test if an individual is below the limit below which it is considered safe to drive. This test is used to estimate the blood alcohol content (BAC) through the measurement of the breath alcohol content (BrAC). The proportionality of BAC to BrAC is 2100
:
1.8 Early breathalysers used potassium dichromate, a strong oxidiser that can react with ethanol (amongst others analytes), and a photodetector. Newer breathalysers can be connected directly to a smartphone and contain a fuel cell that has an output that is proportional to the amount of ethanol in the breath.8 Small scale breath alcohol sensors based on organic electrochemical transistors on paper substrates have also been demonstrated.9 This demonstrates how much sensor technology for such a commonly used application of breath analysis has advanced.
Quantitative breath analysis is a relatively new diagnostic tool. However, other forms of breath analysis pre-date technological methods by thousands of years. The ancient Greeks, for example, already knew that breath smelling sweet, fruity or acetone-like is in an indicator of uncontrolled diabetes, a fishy smell indicates liver disease, and a urine-like smelling breath indicates kidney failure.10 However, one could say that more formally the field of breath analysis started to develop around 1970, when Pauling used liquid–gas partition chromatography to discover that a breath sample contains at least 250 different components.11 Over time more breath components were added to the list and it is now thought that a breath sample can contain around 3500 different compounds.12 The main components, nitrogen, oxygen, carbon dioxide, and water vapour make up the bulk of a breath sample and the thousands of other components are present at parts per million (ppm), parts per billion (ppb), or parts per trillion (ppt) concentrations.13–15
Out of the 3500 components typically found in a breath sample some of the most important markers for various diseases and disorders include ammonia, acetone, isoprene, nitric oxide, hydrogen sulphide, aldehydes, methane, ethane, and pentane.16–18 The focus of this review is on the detection of ammonia in breath samples. The ammonia content of a breath sample is of particular interest because it can provide information about various diseased states related to amongst others gastric, renal, hepatic, and pulmonary function.
Apart from information about diseased states and metabolic processes, breath samples are also affected by the composition of the inhaled air and by food or drinks ingested. This is why, although breath analysis can be an incredibly powerful tool, the samples are complex and extracting the required information can be a challenge.
Most established ammonia breath analysis methods require relatively large pieces of equipment. However, reliable sensing of small concentrations of ammonia has been demonstrated with modern, small scale technologies. This review will focus on novel sensor technologies for ammonia breath analysis that, because of their small scale and relatively low cost, are a promising solution for point-of-care breath analysis.
2. Ammonia sensing for diagnostics
Ammonia plays an important role in various processes in the human body. Therefore, analysis of the ammonia concentration in a breath sample can be used to identify a large range of acquired or inherited diseased states, related to many of the major organs.
Ammonia is a waste product from the breakdown of proteins. In a healthy individual ammonia and carbon dioxide are converted into urea via the urea cycle (Fig. 1). This conversion takes place in the mitochondria of the liver cells. Next, urea enters the bloodstream, is filtered by the kidneys and finally excreted in urine. In mammals 80% of nitrogen is excreted in the form of urea, therefore the correct functioning of all components of the urea cycle is crucial. Blood ammonia levels are typically 11–50 μM, but levels over 100 μM can affect many of the major organs as well as cause neurological and cognitive impairment.19 Apart from conditions affecting the urea cycle, the concentration of ammonia in exhaled breath can also be affected by for example oral microflora in the saliva, pulmonary dysfunction or dental issues. This means that mouth-exhaled ammonia concentrations cannot be assumed to be equivalent to alveolar or blood ammonia concentrations. Mouth-exhaled ammonia concentration for healthy individuals are typically 300–1500 ppbv, whereas nose-exhaled ammonia concentrations are typically only 100 bbpv.2 For example, one study reports median ammonia concentrations of 688 ppbv and 34 pbbv respectively for mouth- and nose-exhaled breaths in healthy individuals.3 Furthermore, it is important to note that one analyte can be a marker for multiple diseased states and that a diseased state can be characterised by changes in multiple markers.
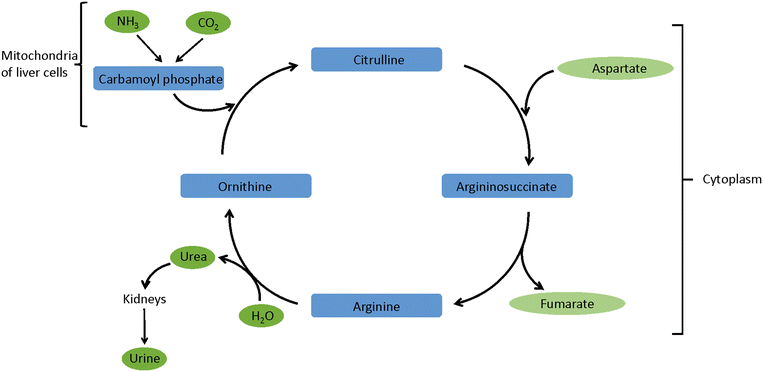 |
| Fig. 1 Schematic representation of the urea cycle. In the mitochondria of the liver cells ammonia and carbon dioxide are converted into carbamoyl phosphate. This is converted into urea via a multistep process in the cytoplasm of the liver cells. Finally, urea is transported via the bloodstream to the kidneys, where it is converted into urine. | |
2.1 Renal failure
When kidney failure occurs the urea cycle is functioning correctly, but the kidneys are unable to process the urea. This causes blood urea nitrogen (BUN) to accumulate in the body. Urea enzymes in the gastrointestinal tract then decompose the excess urea into ammonia and carbon dioxide which are excreted via the breath. In patients with renal disease breath ammonia levels before and after haemodialysis were around 2000 ppb and 200 ppb respectively, as measured with a laser-based breath sensor.18,20
Although breath analysis for this purpose has been demonstrated, diagnosis using blood, urine, or sputum samples remains clinical practice. One breath analysis example uses semiconducting metal oxide vapour sensors to diagnose kidney failure, measuring up to 120 ppm ammonia in individuals with kidney failure versus concentrations below 50 ppm for healthy indivduals.21
2.2 Hepatic encephalopathy
Hepatic encephalopathy (HE) is a neuropsychiatric condition caused by an increased ammonia concentration in the blood due to liver dysfunction. The ammonia toxicity caused by a disrupted urea cycle affects the brain tissue. Using an electronic sensor it was shown that breath analysis can be used to distinguish between the healthy control group and liver cirrhosis patients with HE, with average breath ammonia concentrations of 151 ppb and 184 ppb, respectively.22
2.3
Helicobacter pylori
Helicobacter pylori (H. pylori) is a type of bacterium that infects the stomach or duodenum and is a common cause of peptic ulcers. H. pylori infections are typically diagnosed through ammonia breath analysis after the patient ingests a tablet containing urea labelled with 13C. If H. pylori is present, urease produced by the bacteria rapidly breaks down the isotopically labelled urea and an increased concentration of CO2 containing 13C in the breath sample is measured. It is also possible to diagnose the presence of H. pylori through an increase in exhaled ammonia concentration after the ingestion of unlabelled urea, as was for example demonstrated using a fibreoptic sensor using a pH dye that is sensitive to ammonia.23 Eliciting a response through the ingestion of a urea tablet is an interesting approach because it makes it possible to do a control experiment on the same individual before the tablet is ingested. This is an important advantage compared to most diagnostic breath tests, where a test result is compared to typical ranges of values for healthy and diseased individuals instead of a control experiment on the same individual. This removes uncertainty caused by variations between individuals and environmental factors influencing the breath sample.
2.4 Halitosis
Halitosis, or chronic bad breath, can be caused by a range of conditions including mouth, nose or throat infections, dental issues, dry mouth, smoking or tobacco use, or underlying conditions such as gastric reflux, diabetes, and liver or kidney disease. About 85% of halitosis cases have an oral cause, whereas 15% have a non-oral cause and can be a symptom of a systemic disease.24 Apart from ammonia, other compounds such as hydrogen sulphide, hydrogen, sulphur dioxide, and various volatile organic compounds are also known markers of halitosis. The larger number of potential causes and markers of halitosis makes it an interesting challenge for breath analysis. Apart from analysing the different markers, determining the origin of each marker is of key importance. For this reason, research has been directed at quantifying the concentrations of halitosis markers in oral, nasal, and alveolar breath by using different sampling protocols.25
2.5 Pulmonary dysfunction
There is a range of lung conditions that can be diagnosed or monitored through ammonia breath analysis. For example, the metabolic crisis associated with hyperammonaemia can cause hyperventilation in order to improve carbon dioxide removal. Breath ammonia monitoring may also contribute to the monitoring of cystic fibrosis, but is unlikely to compete with the established diagnosis method based on salt in sweat. Furthermore, asthma, a chronic inflammatory disease of the airways, is usually diagnosed by a respiratory function test, but has the potential to be diagnosed by markers such as nitric oxide and ammonia. These examples highlight that for some applications breath ammonia analysis is an option, but other more established methods are available. For breath analysis to become more widely used it must offer significant advantages over the established methods.
One recent example shows that breath analysis offers the possibility of early and non-invasive diagnosis of interstitial lung disease, using an electronic nose with 7 different cross-reactive metal oxide sensors for breath analysis.26 The advantage of this method is that it could be used at point-of-care and would reduce the time to diagnosis.
3. Samples for breath analysis
Breath analysis is a powerful tool for a range of diagnostic applications. However, the variations in concentration of most markers indicating the difference between a healthy and a diseased state can be relatively small. Furthermore, those small variations are seen against a background of a complex breath sample. Therefore, in addition to sensitive and selective sensor technologies, it is important to consider the sampling method as well as any sample storage or transportation required before the sample is evaluated.27
It should also be noted that although sensors performance should be tested with real breath samples, often simulated breath samples are used at earlier stages of sensor development and testing. Simulated breath samples have a composition that is similar to that of real breath samples but allow for more experimental control and are often more easily available to the researchers.28
3.1 The breath sample
A breath sample consists of alveolar air and dead space air. Alveolar breath originates from the alveoli, deep in the lungs, and is in equilibrium with systemic blood. The marker concentrations found in alveolar air are similar to those found in end-tidal breath, defined as the last part of the exhaled breath. The term end-tidal breath is more applicable to a practical sampling situation and does not imply that it is identical to the equilibrated air inside the alveoli, although the composition is often similar. For highly soluble volatile organic compounds (VOCs) gas exchange occurs in the airways, causing a discrepancy between end-tidal breath and the alveolar breath. Furthermore, in addition to systemic origins, ammonia can originate from the oral cavity because the microflora in the saliva contributes strongly to the ammonia concentration, this alters the composition of the breath and results in a difference in ammonia concentration between mouth- and nose-exhaled breath.
Dead space air, on the other hand, is defined as the volume of air acting as a conducting pathway, originating from the nose, pharynx, larynx, and trachea. The concentration of the analyte in dead space air does not reflect the concentration of the analyte in the blood. However, dead space air does contain information about the inhaled breath and for example about infections in the oral cavity or throat. Therefore, it is important to consider how a sample is taken and how each part of the exhaled breath is interpreted. For example, to avoid diluting alveolar air with dead space air is it possible to discard the first part of the exhaled breath sample. The simplest way to do this is to discard the first approximately 500 mL of a breath sample, however this does not account for differences between individuals. It is more accurate to use CO2 concentration to differentiate between alveolar air and dead space air. The first part of a breath sample does not contain CO2, then there is a sharp increase in CO2 concentration until a plateau is reached, known as the alveolar plateau or expiratory plateau (Fig. 2).17
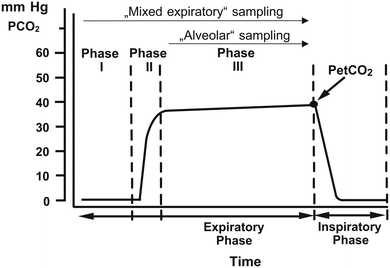 |
| Fig. 2 Schematic representation of the CO2 content of 1 breath cycle. Phase I consists of dead space air and does not contain CO2 or endogenous VOCs. Phase II is a transition phase from dead space air to alveolar air, characterised by a sharp increase in CO2 concentration. Phase III is known as the alveolar plateau or expiratory plateau. Finally, PetCO2 is the end tidal CO2 concentration. Reproduced with permission from Miekisch 2004.17 | |
Furthermore, changes in flow rate, ventilation, body posture, and stress affect the composition of a breath sample. It has been shown that breath holding significantly increases the concentration of gases like H2, CH4, and CO in exhaled breath.27 Averaging over multiple breath cycles and the use of controlled breathing patterns has been shown to decrease variability.
3.2 Practical sampling challenges
In addition to the differences between healthy and diseased states and sampling methods, factors that influence the composition of a breath sample include the environment or inhaled breath, ingested food and drink, age, gender, and pregnancy. This makes it challenging to define a baseline with respect to which samples are evaluated. Differences between individuals have to be minimised such that they are insignificant compared to typical differences between healthy and diseased states. Good sampling protocols will help reduce some variations. In early breath analysis studies Pauling used subjects who had fasted prior to the experiment to reduce variability.11 However, this is not realistic for most clinical breath analysis applications and largely negates the practical and non-invasive nature of breath analysis. A recent example of a device for breath analysis that controls for environmental variations is the SpiroNose.26 This set-up has internal as well as external sensors to take a control sample of the environmental air to which the breath sample is compared. Another way to address baseline issues caused by variations between individuals is to conduct a control experiment on the same individual, determining the baseline for that individual. Although this is not possible in the majority of applications of breath analysis, it is possible in the case of an H. pylori infection (section 2.3). First, the baseline for a patient is determined by taking a breath sample. Next, the patient ingests a urea tablet and after a short interval a second breath sample is taken. A comparison of the two samples is used to diagnose an infection of H. pylori, independent of environmental and inter-individual variations.
3.3 On- and off-line sampling
Finally, although on-line sample analysis is preferable, a lot of breath analysis methods still require off-line sample analysis. This is often due to large and costly equipment and specialist staff being required that are only available in a limited number of dedicated laboratories. If a sample cannot be analysed on-line, directly after and in the same set-up as the sample was taken, it has to be stored and transported before it can be analysed. This means that factors influencing sample degradation have to be considered, such as the material of the sample container. The material has to be stable enough to not contaminate the sample through the breakdown of the container and impermeable enough to prevent exchange of components between the interior and the exterior of the container, keeping the sample in and contaminants out. Typical sample containers for breath analysis are made of polymeric materials. Examples of frequently used sample containers include Tedlar bags, Nalophan bags, and aluminium coated bags.18 Furthermore, humidity can play an important role in sample storage. Human breath is warm and has an 80–90% relative humidity. As it cools down after exhalation some of that humidity condenses inside the sample container, changing the composition of the vapour sample.
Therefore, to prevent sample degradation, on-line sampling is preferable. Chemical sensors are small scale and relatively inexpensive and easy to use. This means that chemical sensors used for breath analysis have the potential to lead to the development of point-of-care breath analysis solutions that eliminate the requirement for sample storage and transportation.
4. Breath analysis methods and materials
4.1 Vapour sensing
Despite the availability of modern technological solutions, sniffer dogs are still considered the gold standard of vapour sensing. Dogs have a highly sensitive sense of smell and can be trained to indicate to their handler when they smell an analyte vapour. It has been shown that sniffer dogs can be successfully used for medical diagnosis, for example for various cancers.29 However, for most applications technological methods are more practical than trained dogs because dogs need training, care, and rest, and because of their attachment to their handler. Furthermore, although they are excellent qualitative detectors, sniffer dogs do not provide a quantitative response.
Most established technological vapour sensing methods rely on separation or ion detection methods, or on laser-based methods.30 Gas chromatography can be used to separate and analyse a variety of compounds. Compounds are vaporised without decomposing them. A carrier gas carries the vapour through a column and due to the difference in interactions with the stationary phase, a liquid or polymer coating on the inside of the column, different vapours have different retention times in the column. Detectors like for example an electron capture detector, a thermal conductivity detector, or a nitrogen–phosphorus detector can be used to detect the separated components of the vapour sample. With mass spectrometry, on the other hand, the analyte is split into charged fragments that are separated based on their mass-to-charge ratio in a magnetic and/or electric field. The combinations of gas chromatography and mass spectroscopy (GC/MS) is particularly useful because it can be used for the identification of hundreds of components of a breath sample. In ion mobility spectrometry (IMS) molecules are ionised and then split based on their mobility in a drift gas.30 There is also a range of different laser-based methods that can be used for vapour sensing. For breath analysis, rotational-vibrational absorption spectroscopy is the most commonly used method. Optical methods offer a high level of sensitivity and selectivity and can be used for real-time breath analysis. However, most optical methods are focused on analysing a single breath component, instead of monitoring multiple sample components such as is possible with GC/MS or a sensor array.31
These well-established methods for vapour sensing and breath analysis applications benefit from a large volume of knowledge available on these techniques and highly stable equipment that can provide reliable and repeatable results. However, these techniques require large and costly pieces of equipment and specialist analysis. This makes them unsuitable for point-of-care use, adding additional challenges with regards to sample storage and transportation.
4.2 Chemical sensors
Chemical sensors offer a promising alternative to the more established methods. Chemical sensors rely on an interaction between the sensor material and an analyte molecule that causes a measurable change to the sensor. Examples of different types of chemical sensors include chemiresitive sensors that rely on measuring a change in the electrical resistance through the sensor material upon interaction with the analyte molecules, optical sensors that make use of changes in the optical properties of the sensing materials, quartz crystal microbalances (QCMs) that make use of a change in the resonance frequency of the quartz crystal caused by the adsorption of the analyte molecules, and cantilevers that use changes in the mass, frequency, bending or bulk stress of the cantilever for vapour sensing. What these different types of sensors have in common is that the chemical interaction with or adsorption of the analyte onto the sensing material causes a measurable response.32
One important advantage of this approach is that by changing the properties of the sensor layer, the properties of the sensor can be changed. For example, changes to the chemical properties of the sensor material can change its interaction with the analyte and allows it to be tailored to specific sensing applications. Additionally, a change to the sensing layer can change the way the chemical interaction affects the sensor signal, for example increasing the sensitivity by changing the architecture of the sensing layer.
What all chemical sensors have in common is that they can be integrated in devices that are small scale, relatively cheap, and easy to use. This is a major advantage that chemical sensors have over the more established breath analysis methods and is a promising solution for point-of-care breath analysis. Chemical sensors for ammonia detection have been widely studied for a range of applications including safety, security, and environmental monitoring. Although chemical sensors for ammonia breath analysis are not as widely studied, they show significant promise for this application.33
4.3 Sensor materials
Apart from the variety of sensor mechanisms there are also many different types of sensor materials that can be used in chemical sensors. Possible sensor materials include semiconducting metal oxides, nanomaterials, polymer materials, composites, and biomimetic materials. All of these materials benefit from being small scale and relatively easy to tailor. However, they all have advantages and disadvantages. Semiconducting metal oxides are the most established group of materials for chemical sensors and are typically used in chemiresistive sensors. Most commercially available gas sensors make use of metal oxides as their sensing material. This is in large part due to their superior stability compared to for example sensing layers with organic components. Furthermore, because metal oxide-based sensors are operated at elevated temperatures, they are usually highly reversible and relatively unaffected by humidity in the sensing environment. This is a highly versatile group of materials and there are many parameters that can influence their sensing properties, including porosity, doping, crystal phase, operating temperature, humidity, and particle size and morphology.34 For example, it was shown that by operating a Si-doped α-MoO3 sensor for NH3 breath analysis at 400 °C it can be operated at 90% RH, as is required for breath analysis (Fig. 3).35 However, elevated temperatures can also be a disadvantage because of the increased power consumption and larger device size required to accommodate a heating element and a larger power supply.36
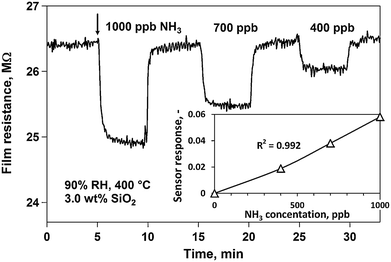 |
| Fig. 3 Resistance and sensor response (inset) of a Si-doped MoO3 sensor upon expose to 1000, 700, and 400 ppb ammonia. The experiments were conducted at 90% RH and a 400 °C sensor operating temperature. Reproduced with permission from Guntner 2016.35 | |
Conducting polymers are a promising material for chemical sensors because they allow for relatively easy and inexpensive processing. Furthermore, they are operated at room temperature and are highly versatile because their properties can be tailored for specific sensing applications. However, most polymer-based sensors are strongly affected by humidity. Nanomaterials are often used instead of layers of bulk materials. By increasing the surface-to-volume ratio of the sensor material it is possible to increase the sensitivity. Nanomaterials can also be used to increase the sensitivity of or add functionality to other sensor materials.37,38 One example uses a tube sensor based on a network of polyaniline/Eu3+ nanofibers with a large specific surface area for low-volume ammonia detection (Fig. 4).39 Sensors based on polymers or metal oxides are very versatile and their relative simplicity is appealing. Most of these sensing materials make use of an oxidation or reduction mechanism. The interaction with the analyte molecules causes an increase or a decrease in the majority charge carrier density, causing a measurable change in the resistance of the sensor material. For example, when p-doped polypyrrole (PPy) is exposed to an electron donating gas like ammonia, the majority charge carrier density of the PPy layer is reduced, causing an increase in the resistance of the PPy layer.40 Another example uses titanium dioxide films annealed at 873 K that show a decrease in resistance upon exposure to ammonia because in that case ammonia acts as a reducing agent.41 However, because the interactions with analyte molecules often rely on an unspecific interaction like a redox reaction, these sensors tend to suffer from low selectivity or specificity. One possible solution to increase selectivity, and sometimes also sensitivity, is to use composite materials. The use of composite materials significantly increases the possibilities to tailor a material, and therefore new sensors based on composite materials are developed frequently. However, the use of composite materials complicates processing and increases the cost of the sensors. The cost–benefit-balance can be especially important when keeping future large-scale processing and possible commercialisation in mind.
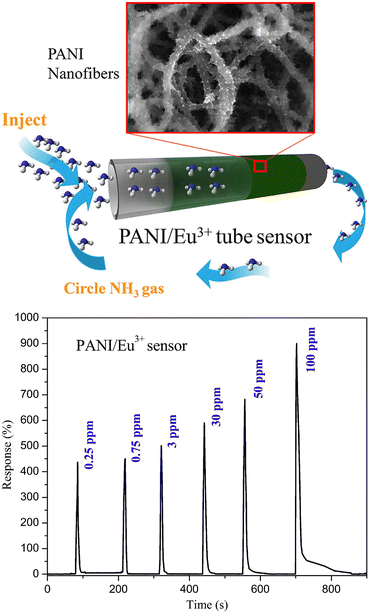 |
| Fig. 4 Polyaniline (PANI) fibers embedded with Eu3+ were used to form a tube sensor (top) for the detection of 0.25–100 ppm ammonia (bottom). Reproduced with permission from Zhang 2020.39 | |
Finally, biomimetic sensing materials are interesting because they make use of the specificity found in biological interactions. By mimicking rather than copying biological systems it is possible to make sensor production easier and more affordable without losing the advantage of biological specificity. Furthermore, it makes it possible to tailor the material to different environments, for example outside the human body. This approach is especially interesting for sensing and monitoring of biological processes and detection of larger molecules. To avoid overcomplicating the production process of biomimetic sensor materials, processes such as molecular imprinting and self-assembly are often used.42,43
4.4 Recent examples of chemical sensors for ammonia
Although chemical sensors for ammonia breath analysis are relatively new, chemical sensors for ammonia detection have been widely studied and examples of chemical sensors for ammonia breath analysis have been tested, although mostly in a laboratory environment. Examples include sensors based on barium hexaferrite for highly selective and stable ammonia sensing,44 gold nanoparticle sensitised V2O5/CuWO4 heterojunctions,45 polymer films with nanopores,46,47 and polyaniline nanoparticles that were inkjet-printed on interdigitated electrodes (Fig. 5).28 Examples that also focus on future sensor implementation include a prototype hand-held breathalyser using metal oxide-based sensors allowing for on-line analysis48 and a carbon nanotube composite-based sensor with an integrated read-out system.49 Apart from laboratory testing and clinical trials on human subjects, some sensors are also tested on animals. For example, organic nanojunctions for ammonia sensing to diagnose hepatic injury were successfully tested on rats (Fig. 6).50
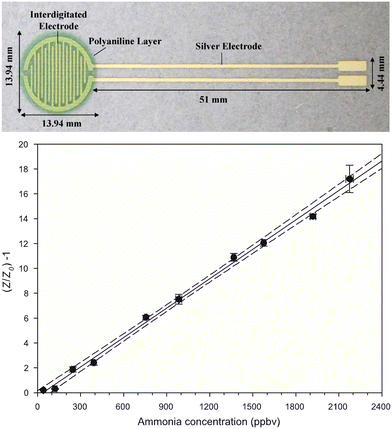 |
| Fig. 5 Top: screen printed silver interdigitated electrodes covered with multiple layers of an inkjet printed polyaniline nanoparticle dispersion, forming a green film. Bottom: ratiometric impedance response vs. ammonia concentration of such a sensor. Reproduced with permission from Hibbard 2013.28 | |
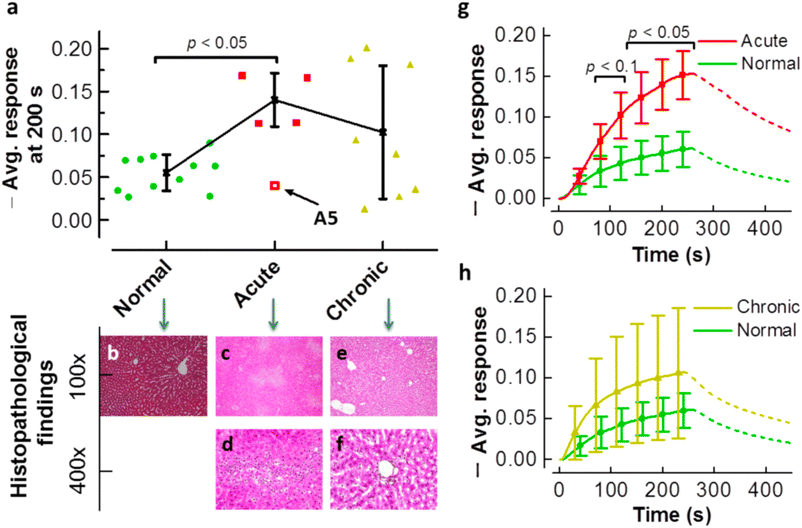 |
| Fig. 6 a) Average sensor response to ammonia of a poly(3-hexylthiophene-2,5-diyl) (P3HT)-based sensors tested on rats that were healthy (green), had acute (red), or chronic (yellow) hepatic injury. And hepatopathology alternation images of b) the healthy group, c) and d) the acute group and e) and f) the chronic group. Histological analysis shows necrosis and haemorrhage with inflammatory cell infiltration in the liver tissue of the acute group. Average sensing responses vs. time for g) the normal and acute group and h) the normal and chronic group. Showing an increased average sensor response when hepatic injury is present. Reproduced with permission from Dai 2013.50 | |
Despite this large range of examples being available, chemical sensors for breath analysis are still a rapidly developing field of research. A large body of research is available on chemical sensors for the detection of low concentrations of ammonia in general, and many of these technologies could potentially be suitable for breath analysis applications after modifications. These modifications would have to address the challenges that are unique to the field of breath analysis, such as very high levels of humidity, low analyte concentrations, and complex samples. This means that although chemical sensors for breath analysis are promising, further development is required before practical implementation is possible. One example of a breath analysis device based on chemical sensors that has been commercialised is the SpiroNose by Breathomix.51,52 It is an e-nose consisting of multiple metal-oxide-based sensors and has been used for the detection of volatile organic compounds for the diagnosis of various types of cancer, inflammatory and infectious diseases.
5. Challenges and potential solutions to chemical sensors for breath ammonia
Remaining challenges have to be resolved before they can be implemented or commercialised on a larger scale. The remaining challenges include sensitivity, selectivity, humidity, stability, and miniaturisation.
5.1 Sensitivity
Sensitivity is important because apart from detecting low concentrations of vapours, it is important to be able to distinguish between the concentrations indicating healthy and diseased states. This means that sensors need to distinguish between small variations in low concentrations of the analyte molecule. Sensitivity can be enhanced either through the choice of sensing material or by changing the architecture of the sensing layer, for example by using a thinner film, increasing the surface area by using nanoparticles, or by using a percolation network. It has been shown that by using a percolation network of conductive polymers instead of the more traditional conductive polymer thin film it is possible to significantly increase the sensitivity. This has been demonstrated in a laboratory set-up for NH3, NO2, and ammonium nitrate/fuel oil (ANFO) vapour sensing (Fig. 7).40,53,54
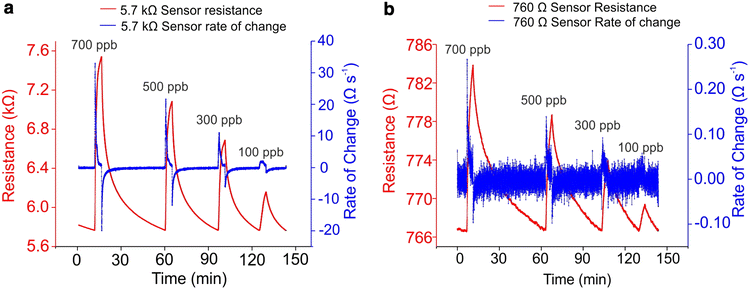 |
| Fig. 7 Comparison of sensing responses to 700, 500, 300, and 100 ppb ammonia of a) a sensor based on a polypyrrole (PPy) percolation network and b) a sensor based on a PPy thin film. The data shows a significantly increased sensitivity achieved with a percolation network compared to a thin film. At 700 ppb the response, defined as ΔR/R0 × 100%, is 33% for the percolation network-based sensor and 2.3% for the thin film-based sensor. Reproduced with permission from Armitage 2020.40 | |
5.2 Selectivity
Selectivity is a challenge with most chemiresitive sensors because the chemical interactions that they rely on are often not unique to a single chemical compound, such as those based on redox reactions. In breath analysis selectivity can be particularly important because breath samples can contain thousands of compounds. For this reason, selectivity is an important parameter of a good sensor or sensor system.
One possible solution is to use more unique interactions such as in the case of biological or biology inspired systems. However, in the case of small analyte molecules, such as NH3 and many other compounds in exhaled breath, this is often not possible.
Another solution is to use an electronic nose. An electronic nose consists of an array of different sensors that together provide a fingerprint pattern of responses to an analyte vapour. This helps provide the selectivity that cannot be achieved with one individual sensor. For example, it was shown that an electronic nose consisting of an array of 11 polyaniline nanocomposite sensors, in combination with feature selection and Support vector Machine, can improve diagnosis accuracy.55 Another recent example uses an array of metal oxide sensors to distinguish between three different lung diseases (Fig. 8).26 This is an interesting solution because the combination of multiple small and relatively cheap sensors still results in a small and affordable device. Finally, in some cases it is possible to minimise the selectivity challenge by conducting a control experiment in the same environment and on the same patient. This makes it possible to look for a difference between two measurements rather than an absolute value against a background of 3500 components.
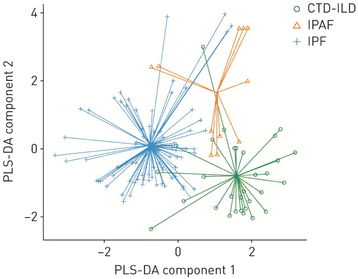 |
| Fig. 8 Electronic nose results obtained with a SpiroNose, combining 7 different metal oxide sensors and a spirometer. Statistical analysis using partial least square discriminant analysis (PLS-DA) demonstrates that this electronic nose can be used to differentiate between connective tissue disease -ILD (CTD-ILD), interstitial pneumonia with autoimmune features (IPAF), and idiopathic pulmonary fibrosis (IPF). Reproduced with permission from Moor 2021.26 | |
5.3 Humidity
A typical breath sample contains about 80–90% relative humidity. To avoid condensation of the sample on the sensor, the sensor should where possible be operated at or above body temperature. Furthermore, many chemical sensors are also sensitive to humidity. This causes two issues. First of all, if there is a lack of selectivity, it might not be possible to distinguish between the large water vapour signal and a smaller signal from the target molecule. Secondly, water molecules may block all the reactive sites, saturating the sensor. In this case the sensor is no longer able to interact with the target molecules.
Using an e-nose can help reduce the issues caused by humidity if they are due to selectivity alone. However, if the sensor is being saturated by high levels of humidity, additional solutions are needed. It is possible to use sample preparation steps, such as using a desiccant or protecting the sensor surface with a layer that is selectively permeable or works as a desiccant. One example uses a membrane of multi-walled carbon nanotubes for enhanced condensation to help dry the sample before it reaches the sensor surface, thereby reducing the effect of humidity on the sensor.56 Operating a sensor at an elevated temperature can also reduce issues caused by high levels of humidity. In one example, a sodium hydroxide filter was placed between the sample inlet and a conducting polymer nanojunction for ammonia breath analysis to avoid the high relative humidity of the sample affecting the sensor.58 In another example, non-conjugated hydrophilic polymers were used to make use of the high humidity, rather than it being a hindrance to the sensor performance. This allows the water to become part of the functional material and absorb and ionise the ammonia (Fig. 9).57
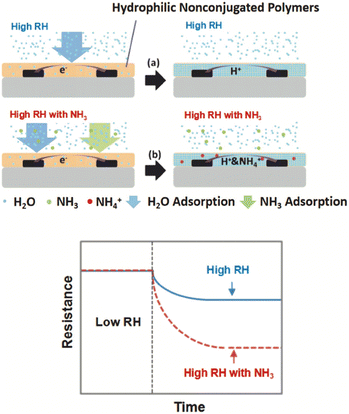 |
| Fig. 9 Schematic showing a sensor based on hydrophilic nonconjugated polymers responding to a) a high RH and b) a high RH and ammonia. These sensors make use of high levels of humidity to increase the sensor response by using the water to absorb and ionise the ammonia, rather than viewing high levels of humidity as a disadvantage. Reproduced with permission from Zhao 2020.57 | |
5.4 Stability
Sensor stability is perhaps not particularly important at a proof-of-concept stage, where a sensor can often be fabricated and tested within hours. However, for practical implementation and commercialisation, stability is a critical parameter. The established breath analysis technologies, such as those based on GC/MS, offer high levels of stability and reproducibility. Therefore, to be able to compete, chemical sensors need to offer enough stability to provide reliable results. If cheap single-use sensors are commercialised, the environmental impact must also be considered.
Metal oxide-based sensors are very stable over time. However, more novel materials, especially small-scale organics-based systems suffer from degradation. This is often due to exposure to oxygen, humidity, or light. Sensors can also degrade after multiple exposure and recovery cycles, if sensor recovery is incomplete, or due to an electrical current running through the sensor material for a prolonged period of time.
5.5 Miniaturisation
Finally, most chemical sensors have a small footprint and are inherently suitable for small scale applications. However, further miniaturisation is still an interesting challenge in this field. The challenge lies mostly in miniaturising components surrounding the sensors, not the sensing element itself. Device components such as power and heat sources, equipment needed for sample preparation, desiccation, pre-concentration steps, and technology needed to display or send the sensor data can limit miniaturisation. Although this can be relatively easily achieved for bench top or even handheld devices that could be used at point-of-care, reducing the size even further is a challenge. These challenges are especially important for personal monitoring applications and for sensor incorporation into smartphones or smartwatches.59 Therefore, it is desirable to develop sensors that do not require additional components that make miniaturisation challenging. One example of a metal oxide-based sensor uses a miniature hot plate to improve miniaturisation.60
6. Conclusions and perspectives
Ammonia breath analysis is a promising and evolving field of medical investigation that offers the potential for widely-available non-invasive point-of-care diagnosis. Chemical sensors for breath analysis have a distinct advantage over more established technologies by making diagnosis and patient monitoring more affordable, faster, easier and therefore more suitable for point-of-care devices.
Although much research activity is directed at using chemical sensors for medical breath analysis, commercialisation and practical implementation of these technologies are still in their early stages. To be able to more widely implement chemical sensors for breath analysis for a range of applications a number of challenges still have to be fully addressed, including improvements in sensitivity, selectivity, stability, and the reduction of the influence of humidity. Miniaturisation of some device components is also required.
The next steps in this field will most likely involve the further development of chemical sensors based on a broad range of materials that lead to improved e-nose devices. Ultimately, one could envisage an e-nose that was integrated into a smartphone and carried out breath analysis during a telephone conversation. This would enable daily personalised monitoring of a range of medical conditions to be carried out, and facilitate the early identification of new diseases.
Conflicts of interest
There are no conflicts to declare.
Acknowledgements
We thank the EPSRC for financial support through the Impact Acceleration Award EP/R511742/1.
References
- D. Smith and P. Španěl, The challenge of breath analysis for clinical diagnosis and therapeutic monitoring, Analyst, 2007, 132(5), 390–396 RSC.
- P. Španěl and D. Smith, What is the real utility of breath ammonia concentration measurements in medicine and physiology?, J. Breath Res., 2018, 12(2), 027102 CrossRef PubMed.
- F. Schmidt, O. Vaittinen, M. Metsälä, M. Lehto, C. Forsblom and P. Groop,
et al., Ammonia in breath and emitted from skin, J. Breath Res., 2013, 7(1), 017109 CrossRef CAS PubMed.
- S. F. Solga, M. Mudalel, L. A. Spacek, R. Lewicki, F. Tittel and C. Loccioni,
et al., Factors influencing breath ammonia determination, J. Breath Res., 2013, 7(3), 037101 CrossRef CAS PubMed.
- M. D. Davis, A. Montpetit and J. Hunt, Exhaled breath condensate—an overview, Immunol. Allergy Clin. North Am., 2012, 32(3), 363 CrossRef PubMed.
- C. Gessner, H. Kuhn, K. Toepfer, S. Hammerschmidt, J. Schauer and H. Wirtz, Detection of p53 gene mutations in exhaled breath condensate of non-small cell lung cancer patients, Lung Cancer, 2004, 43(2), 215–222 CrossRef PubMed.
- D. J. Ryan, S. Toomey, S. F. Madden, M. Casey, O. S. Breathnach and P. G. Morris,
et al., Use of exhaled breath condensate (EBC) in the diagnosis of SARS-COV-2 (COVID-19), Thorax, 2021, 76(1), 86–88 CrossRef PubMed.
- M. R. Rahman, J. T. Allan, M. Z. Ghavidel, L. E. Prest, F. S. Saleh and E. B. Easton, The application of power-generating fuel cell electrode materials and monitoring methods to breath alcohol sensors, Sens. Actuators, B, 2016, 228, 448–457 CrossRef CAS.
- E. Bihar, Y. Deng, T. Miyake, M. Saadaoui, G. G. Malliaras and M. Rolandi, A disposable paper breathalyzer with an alcohol sensing organic electrochemical transistor, Sci. Rep., 2016, 6(1), 27582 CrossRef CAS PubMed.
- W. Cao and Y. Duan, Current status of methods and techniques for breath analysis, Crit. Rev. Anal. Chem., 2007, 37(1), 3–13 CrossRef CAS.
- L. Pauling, A. B. Robinson, R. Teranishi and P. Cary, Quantitative analysis of urine vapor and breath by gas-liquid partition chromatography, Proc. Natl. Acad. Sci. U. S. A., 1971, 68(10), 2374–2376 CrossRef CAS PubMed.
- T. A. Popov, Human exhaled breath analysis, Ann. Allergy, Asthma, Immunol., 2011, 106(6), 451–456 CrossRef CAS PubMed.
- W. Filipiak, V. Ruzsanyi, P. Mochalski, A. Filipiak, A. Bajtarevic and C. Ager,
et al., Dependence of exhaled breath composition on exogenous factors, smoking habits and exposure to air pollutants, J. Breath Res., 2012, 6(3), 036008 CrossRef CAS PubMed.
- B. Buszewski, M. Kęsy, T. Ligor and A. Amann, Human exhaled air analytics: biomarkers of diseases, Biomed. Chromatogr., 2007, 21(6), 553–566 CrossRef CAS PubMed.
- T. Hibbard and A. J. Killard, Breath ammonia analysis: Clinical application and measurement, Crit. Rev. Anal. Chem., 2011, 41(1), 21–35 CrossRef CAS.
- S. Das and M. Pal, Non-invasive monitoring of human health by exhaled breath analysis: A comprehensive review, J. Electrochem. Soc., 2020, 167(3), 037562 CrossRef CAS.
- W. Miekisch, J. K. Schubert and G. F. Noeldge-Schomburg, Diagnostic potential of breath analysis—focus on volatile organic compounds, Clin. Chim. Acta, 2004, 347(1–2), 25–39 CrossRef CAS PubMed.
- S. T. Krishnan, J. P. Devadhasan and S. Kim, Recent analytical approaches to detect exhaled breath ammonia with special reference to renal patients, Anal. Bioanal. Chem., 2017, 409(1), 21–31 CrossRef CAS PubMed.
- N. Brannelly, J. Hamilton-Shield and A. Killard, The measurement of ammonia in
human breath and its potential in clinical diagnostics, Crit. Rev. Anal. Chem., 2016, 46(6), 490–501 CrossRef CAS PubMed.
- L. Narasimhan, W. Goodman and C. K. N. Patel, Correlation of breath ammonia with blood urea nitrogen and creatinine during hemodialysis, Proc. Natl. Acad. Sci. U. S. A., 2001, 98(8), 4617–4621 CrossRef CAS PubMed.
- I. O. Essiet, Diagnosis of kidney failure by analysis of the concentration of ammonia in exhaled human breath, J. Emerging Trends Eng. Appl. Sci., 2013, 4(6), 859–862 Search PubMed.
- R. Adrover, D. Cocozzella, E. Ridruejo, A. Garcia, J. Rome and J. Podesta, Breath-ammonia testing of healthy subjects and patients with cirrhosis, Dig. Dis. Sci., 2012, 57(1), 189–195 CrossRef CAS PubMed.
- D. J. Kearney, T. Hubbard and D. Putnam, Breath ammonia measurement in Helicobacter pylori infection, Dig. Dis. Sci., 2002, 47(11), 2523–2530 CrossRef CAS PubMed.
- M. Nakhleh, M. Quatredeniers and H. Haick, Detection of halitosis in breath: Between the past, present, and future, Oral Dis., 2018, 24(5), 685–695 CrossRef CAS PubMed.
- M. Aydin, M. E. Özen, B. Evlice, M. Ferguson and İ. Uzel, A new measurement protocol to differentiate sources of halitosis, Acta Odontol. Scand., 2016, 74(5), 380–384 CrossRef PubMed.
- C. C. Moor, J. C. Oppenheimer, G. Nakshbandi, J. G. Aerts, P. Brinkman and A.-H. Maitland-van der Zee,
et al. Exhaled breath analysis by use of eNose technology: a novel diagnostic tool for interstitial lung disease, Eur. Respir. J., 2021, 57(1), 2002042 Search PubMed.
- C. Lourenço and C. Turner, Breath analysis in disease diagnosis: methodological considerations and applications, Metabolites, 2014, 4(2), 465–498 CrossRef PubMed.
- T. Hibbard, K. Crowley and A. J. Killard, Direct measurement of ammonia in simulated human breath using an inkjet-printed polyaniline nanoparticle sensor, Anal. Chim. Acta, 2013, 779, 56–63 CrossRef CAS PubMed.
- F. Pirrone and M. Albertini, Olfactory detection of cancer by trained sniffer dogs: a systematic review of the literature, J. Vet. Behav., 2017, 19, 105–117 CrossRef.
- M. J. Lefferts and M. R. Castell, Vapour sensing of explosive materials, Anal. Methods, 2015, 7(21), 9005–9017 RSC.
- B. Henderson, A. Khodabakhsh, M. Metsälä, I. Ventrillard, F. M. Schmidt and D. Romanini,
et al., Laser spectroscopy for breath analysis: towards clinical implementation, Appl. Phys. B: Lasers Opt., 2018, 124(8), 161 CrossRef PubMed.
- C. Di Natale, R. Paolesse, E. Martinelli and R. Capuano, Solid-state gas sensors for breath analysis: A review, Anal. Chim. Acta, 2014, 824, 1–17 CrossRef CAS PubMed.
- B. Timmer, W. Olthuis and A. Van Den Berg, Ammonia sensors and their applications—a review, Sens. Actuators, B, 2005, 107(2), 666–677 CrossRef CAS.
- S. Das, S. Mojumder, D. Saha and M. Pal, Influence of major parameters on the sensing mechanism of semiconductor metal oxide based chemiresistive gas sensors: A review focused on personalized healthcare, Sens. Actuators, B, 2022, 352, 131066 CrossRef CAS.
- A. T. Güntner, M. Righettoni and S. E. Pratsinis, Selective sensing of NH3 by Si-doped α-MoO3 for breath analysis, Sens. Actuators, B, 2016, 223, 266–273 CrossRef.
- M. Righettoni, A. Amann and S. E. Pratsinis, Breath analysis by nanostructured metal oxides as chemo-resistive gas sensors, Mater. Today, 2015, 18(3), 163–171 CrossRef CAS.
- U. Tisch and H. Haick, Chemical sensors for breath gas analysis: the latest developments at the Breath Analysis Summit 2013, J. Breath Res., 2014, 8(2), 027103 CrossRef CAS PubMed.
- J. E. Ellis and A. Star, Carbon nanotube based gas sensors toward breath analysis, ChemPlusChem, 2016, 81(12), 1248 CrossRef CAS PubMed.
- W. Zhang, G. Li, C. She, J. Cheng, H. Li and S. Liu,
et al., High performance tube sensor based on PANI/Eu3+ nanofiber for low-volume NH3 detection, Anal. Chim. Acta, 2020, 1093, 115–122 CrossRef CAS PubMed.
- B. I. Armitage, K. Murugappan, M. J. Lefferts, A. Cowsik and M. R. Castell, Conducting polymer percolation gas sensor on a flexible substrate, J. Mater. Chem. C, 2020, 8(36), 12669–12676 RSC.
- B. Karunagaran, P. Uthirakumar, S. Chung, S. Velumani and E.-K. Suh, TiO2 thin film gas sensor for monitoring ammonia, Mater. Charact., 2007, 58(8–9), 680–684 CrossRef CAS.
- B. Bräuer, C. Unger, M. Werner and P. A. Lieberzeit, Biomimetic Sensors to Detect Bioanalytes in Real-Life Samples Using Molecularly Imprinted Polymers: A Review, Sensors, 2021, 21(16), 5550 CrossRef PubMed.
- M. Hussain, J. Wackerlig and P. A. Lieberzeit, Biomimetic strategies for sensing biological species, Biosensors, 2013, 3(1), 89–107 CrossRef PubMed.
- T. Das, S. Das, M. Karmakar, S. Chakraborty, D. Saha and M. Pal, Novel barium hexaferrite based highly selective and stable trace ammonia sensor for detection of renal disease by exhaled breath analysis, Sens. Actuators, B, 2020, 325, 128765 CrossRef CAS.
- H. Naderi, S. Hajati, M. Ghaedi, K. Dashtian and M. Sabzehmeidani, Sensitive, selective and rapid ammonia-sensing by gold nanoparticle-sensitized V2O5/CuWO4 heterojunctions for exhaled breath analysis, Appl. Surf. Sci., 2020, 501, 144270 CrossRef CAS.
- M.-Y. Chuang, C.-C. Chen, H.-W. Zan, H.-F. Meng and C.-J. Lu, Organic gas sensor with an improved lifetime for detecting breath ammonia in hemodialysis patients, ACS Sens., 2017, 2(12), 1788–1795 CrossRef CAS PubMed.
- S.-Y. Yu, T.-W. Tung, H.-Y. Yang, G.-Y. Chen, C.-C. Shih and Y.-C. Lee,
et al., A versatile method to enhance the operational current of air-stable organic gas sensor for monitoring of breath ammonia in hemodialysis patients, ACS Sens., 2019, 4(4), 1023–1031 CrossRef CAS PubMed.
- P. Gouma, K. Kalyanasundaram, X. Yun, M. Stanacevic and L. Wang, Nanosensor and breath analyzer for ammonia detection in exhaled human breath, IEEE Sens. J., 2009, 10(1), 49–53 Search PubMed.
- S. Abdulla, J. Dhakshinamoorthy, V. Mohan, D. V. Ponnuvelu, V. K. Kallidaikuruchi and L. M. Thalakkotil,
et al., Development of low-cost hybrid multi-walled carbon nanotube-based ammonia gas-sensing strips with an integrated sensor read-out system for clinical breath analyzer applications, J. Breath Res., 2019, 13(4), 046005 CrossRef CAS PubMed.
- M.-Z. Dai, Y.-L. Lin, H.-C. Lin, H.-W. Zan, K.-T. Chang and H.-F. Meng,
et al., Highly sensitive ammonia sensor with organic vertical nanojunctions for noninvasive detection of hepatic injury, Anal. Chem., 2013, 85(6), 3110–3117 CrossRef CAS PubMed.
- R. de Vries, R. M. Vigeveno, S. Mulder, N. Farzan, D. R. Vintges and J. J. Goeman,
et al. Ruling out SARS-CoV-2 infection using exhaled breath analysis by electronic nose in a public health setting, medRxiv, 2021, 1–34 Search PubMed.
- R. de Vries and P. J. Sterk, eNose breathprints as composite biomarker for real-time phenotyping of complex respiratory diseases, J. Allergy Clin. Immunol., 2020, 146(5), 995–996 CrossRef CAS PubMed.
- M. J. Lefferts, B. I. Armitage, K. Murugappan and M. R. Castell, PEDOT percolation networks for reversible chemiresistive sensing of NO2, RSC Adv., 2021, 11(37), 22789–22797 RSC.
- M. J. Lefferts, L. H. Humphreys, N. Mai, K. Murugappan, B. I. Armitage and J.-F. Pons,
et al., ANFO vapour detection with conducting polymer percolation network sensors and GC/MS, Analyst, 2021, 146(7), 2186–2193 RSC.
- P. Le Maout, J.-L. Wojkiewicz, N. Redon, C. Lahuec, F. Seguin and L. Dupont,
et al. Polyaniline nanocomposites based sensor array for breath ammonia analysis. Portable e-nose approach to non-invasive diagnosis of chronic kidney disease, Sens. Actuators, B, 2018, 274, 616–626 CrossRef CAS.
- D. Yoon, C. Lee, J. Yun, W. Jeon, B. J. Cha and S. Baik, Enhanced condensation, agglomeration, and rejection of water vapor by superhydrophobic aligned multiwalled carbon nanotube membranes, ACS Nano, 2012, 6(7), 5980–5987 CrossRef CAS PubMed.
- H. Zhao, L. Liu, X. Lin, J. Dai, S. Liu and T. Fei,
et al., Proton-conductive gas sensor: a new way to realize highly selective ammonia detection for analysis of exhaled human breath, ACS Sens., 2019, 5(2), 346–352 CrossRef PubMed.
- A. D. Aguilar, E. S. Forzani, L. A. Nagahara, I. Amlani, R. Tsui and N. Tao, A breath ammonia sensor based on conducting polymer nanojunctions, IEEE Sens. J., 2008, 8(3), 269–273 CAS.
- J.-W. Yoon and J.-H. Lee, Toward breath analysis on a chip for disease diagnosis using semiconductor-based chemiresistors: recent progress and future perspectives, Lab Chip, 2017, 17(21), 3537–3557 RSC.
- T.-C. Wu, A. De Luca, Q. Zhong, X. Zhu, O. Ogbeide and D.-S. Um,
et al. Inkjet-printed CMOS-integrated graphene–metal oxide sensors for breath analysis, npj 2D Mater. Appl., 2019, 3(1), 42 CrossRef.
|
This journal is © The Royal Society of Chemistry 2022 |
Click here to see how this site uses Cookies. View our privacy policy here.