DOI:
10.1039/D2SC05285G
(Edge Article)
Chem. Sci., 2022,
13, 13499-13506
A room-temperature antiferroelectric in hybrid perovskite enables highly efficient energy storage at low electric fields†
Received
22nd September 2022
, Accepted 27th October 2022
First published on 28th October 2022
Abstract
Molecular antiferroelectrics (AFEs) have taken a booming position in the miniaturization of energy storage devices due to their low critical electric fields. However, regarding intrinsic competitions between dipolar interaction and steric hindrance, it is a challenge to exploit room-temperature molecular AFEs with high energy storage efficiency. Here, we present a new 2D hybrid perovskite-type AFE, (i-BA)2(FA)Pb2Br7 (1), which shows ultrahigh energy storage efficiencies at room temperature. Most strikingly, the typical double P–E hysteresis loops afford an ultrahigh storage efficiency up to ∼91% at low critical electric fields (Ecr = 41 kV cm−1); this Ecr value is much lower than those of state-of-the-art AFE oxides, revealing the potential of 1 for miniaturized energy-storage devices. In terms of the energy storage mechanism, the dynamic ordering and antiparallel reorientation of organic cations trigger its AFE-type phase transition at 303 K, thus giving a large spontaneous electric polarization of ∼3.7 μC cm−2, while the increasement of steric hindrance of the organic branched-chain i-BA+ spacer cations stabilizes its antipolar sublattices. To the best of our knowledge, this exploration of achieving ultrahigh energy storage efficiency at such a low critical electric field is unprecedented in the AFE family, which paves a pathway for miniaturized energy storage applications.
Introduction
Solid-state energy storage materials have recently attracted increasing attention, due to the highly urgent demand for sustainable energy sources over the past decade.1–3 Conceptually, antiferroelectric (AFE) materials, characterized by the antiparallel arrangement of adjacent sublattice polarizations,4,5 are emerging as a remarkable candidate for efficient energy-storage applications.6 Benefiting from their typical polarization versus electric field (P–E) double hysteresis loops,7 the unique field-induced AFE-to-ferroelectric (FE) structural transition leads to prominent energy-storage performances,8 including superior energy density, fast charge–discharge rate, and excellent fatigue endurance. These advantages are far beyond linear dielectric and FE materials, as demonstrated by AFE oxides that possess high critical fields (Ecr), such as AgNbO3 (Ecr ∼150 kV cm−1), PbHfO3 (Ecr ∼270 kV cm−1), and PbZrO3 (Ecr ∼463 kV cm−1).9–11 By contrast, molecule-based soft AFEs with intrinsic merits of structural flexibility, facile processability and low operating voltage, are booming as alternatives for energy storage.12–16 It is emphasized that their low Ecr guarantees the possibility of high-level integration into miniaturized electronic devices. For most of the known molecular AFEs, however, an obvious drawback is their low energy-storage efficiency (η), such as TFMBI (Ecr ∼22 kV cm−1, η ∼ 44%) and TCMBI (Ecr ∼81 kV cm−1, η ∼ 62%).17,18 This is ascribed to the large hysteresis (ΔE) between forward and backward switching in the P–E loops. The low η value signifies high energy dissipation that transforms into thermal energy, thus drastically degrading the storage capability. Besides, the optimal η and lower Ecr values usually emerge near the Curie temperature (Tc) for the AFE-to-paraelectric phase transition, for molecular AFEs such as NH4H2PO4 (Tc = 148 K),19 2-difluoromethylbenzimidazole (Tc > 413 K)13 and cyclohexylmethylammonium bromide (Tc = 364 K),20 thus hindering their potential applications. In this context, it is urgently necessary to assemble new room-temperature AFE candidates that enable high energy-storage efficiency under low electric fields.
Recently, the family of 2D Ruddlesden–Popper hybrid perovskites, showing rich and intriguing physicochemical properties,21–24 have provided new entries into diverse applications, such as light-emitting diodes,25,26 solar cells,27,28 lasers,29–31 and photodetectors.32–35 The most fascinating virtue of these 2D members is the breakthrough of the structural limitation of the tolerance factor, which permits an infinite possibility for incorporating bulky spacer cations between inorganic sheets.36 Dynamic motions or orientations of organic spacers are controllable by applying an external electric field; this will greatly facilitate the generation of molecular dipoles and electric order. Previous studies have concentrated on the mixed-cation alloying of flexible moieties to assemble new FE materials, such as (4,4-difluorohexahydroazepine)2PbI4,37 (isoamylammonium)2(EA)2Pb3Br10,38 and (4-aminomethyl-1-cyclohexanecarboxylate)2(EA)2Pb3Br10.39 Contrary to the robust advance of FEs, however, antiferroelectricity in this 2D hybrid perovskite system is still a virgin land with bright prospects. Regarding the antipolar structural and free energy criteria for AFEs, the incorporation of branched-chain organic cations allows a dramatic increasement of steric hindrance in a confined space. Hence, the repulsive interactions caused by the steric hindrance effect would stabilize the antipolar array of dipoles, i.e., the formation of the AFE structure. As verified by organic molecular AFEs, the steric hindrance of organic moieties reduces the distance between adjacent dipoles, thereby creating antipolar motifs.40–42 Despite continued efforts, it remains a challenge to explore room-temperature molecular AFEs in this 2D family, owing to the lack of knowledge regarding the competition balance between steric hindrance and dipolar interactions in homogeneous sublattices.
Here, we have successfully achieved a room-temperature molecular AFE with ultrahigh energy storage efficiency by alloying the branched-chain cation as an interlayered spacer of 2D multilayered hybrid perovskites, (i-BA)2(FA)Pb2Br7 (1, where i-BA+ is iso-butylammonium, and FA+ is formamidinium). Strikingly, the characteristic double P–E hysteresis loops enable ultrahigh energy-storage efficiency of ∼91% under low Ecr about 41 kV cm−1. Such merits reveal its great potential for miniaturized energy-storage devices. The antiparallel reorientations of i-BA+ and FA+ cations lead to its room-temperature antiferroelectricity with a Curie point (Tc) of 303 K, while the increasement of steric hindrance of i-BA+ spacer cations plays an important role in stabilizing its antipolar AFE motif. As far as we know, 1 is the first molecular antiferroelectric to achieve ultrahigh energy storage efficiency at such a low critical electric field, shedding light on exploration of new AFE candidates toward practical applications, e.g., high energy-storage capacitors.
Results and discussion
Variable-temperature structure analyses
Bulk crystals of 1 were prepared from its HBr solution by the slow temperature-cooling method (Fig. S1†), and the purity of the phase was verified by using powder X-ray diffraction (Fig. S2†). Variable-temperature crystal structures of 1 at 200 K (low-temperature phase, LTP) and 310 K (high-temperature phase, HTP) were determined by X-ray diffraction to understand the mechanism of phase transition and the origin of its antiferroelectricity. In the LTP, the structure of 1 is found to be in the orthorhombic space group Pnma (Table S1†). As depicted in Fig. 1a, the basic crystal architectures consist of two perovskite slabs of PbBr6 octahedra, separated by a thickness of two i-BA+ organic cations. A highly contorted perovskite framework verified by the unequal Br–Pb–Br bond angles (84.7–98.4°) allows for the alloying of the FA+ cation into perovskite cavities. For the organic components, the FA+ and i-BA+ cations are ordered and the NH3+ groups in them are antiparallelly aligned toward the a-axis utilizing the N–H⋯Br hydrogen bond interaction with the inorganic framework (Fig. S3†). Such an antiparallel configuration of neighboring dipoles results in an antipolar state (Ps = 0), which reveals the probable antiferroelectricity of 1.
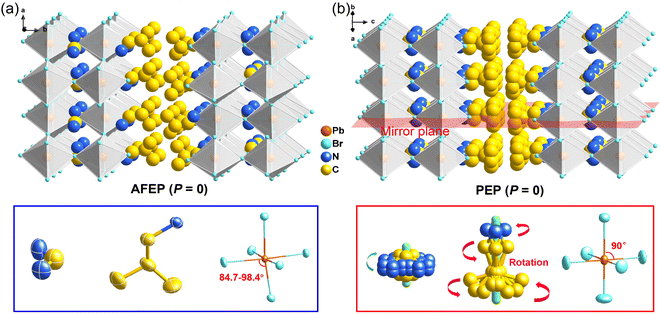 |
| Fig. 1 Crystal structure packing diagrams of 1 in the (a) LTP (left) at 200 K and (b) HTP (right) at 310 K. Upon heating, it is obvious that i-BA+ and FA+ cations become disordered with the dynamic reorientation, while the distorted PbBr6 octahedra return to be regular. | |
At the HTP, 1 crystallizes in the tetragonal centrosymmetric space group I4/mmm. In terms of polarization, the characteristic of the HTP is that both i-BA+ and FA+ cations are located on a crystallographic mirror plane with the completely disordered patterns, thus cancelling the net polarization of the crystal (Fig. 1b). The rotational motion of these organic cations is easily activated at around room temperature, indicating the quite small potential energy barrier of 1, which can be conducive to the generation of molecular dipoles and electric order controlled by applying a low external electric field. Meanwhile, the distorted inorganic PbBr6 octahedra translate to the highly symmetric configurations. This evolution is driven by both their rotational motion around their respective centers with the rotation axis parallel to the b direction and anti-parallel tilting in adjacent inorganic layers (Fig. 2). Therefore, it is the thermally induced order–disorder transition of organic cations and dynamic distortion of inorganic PbBr6 octahedra that synergistically result in the room-temperature antiferroelectric-to-paraelectric phase transition behavior of 1 under low electric fields.
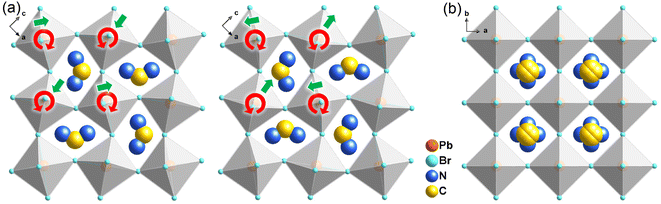 |
| Fig. 2 Schematic view of the rotational and tilted motions of PbBr6 octahedra between the (a) LTP and (b) HTP of 1. Red and green arrows represent the directions of rotation and tilting, respectively. | |
Phase transition properties.
To confirm the phase transition behavior of 1, the differential scanning calorimetry (DSC) measurement was first implemented. Fig. 3a shows the DSC curves that clearly exhibit one pair of reversible peak-type thermal anomalies at 303.2/301.3 K (Tc) in the heating/cooling processes, accompanied by a narrow thermal hysteresis of 1.9 K, indicating the feature of the second-order phase transition. As shown in Fig. 3b, conoscopic images have definitely proved the evolution from biaxial nematic phases (at 300 K) to the uniaxial nematic phases (at 310 K), which coincides with the structural phase transition of mmm-to-4/mmm. Further, we performed the dielectric measurements of 1 in the temperature range 270–330 K. Fig. 3c exhibits the variation of the real part (ε′) and imaginary part (ε′′) of the complex dielectric constant at selected frequencies. The ε′–T plots show peak-like anomalies with the maximum around 303 K and the ε′ values drop gradually on alternating the frequency from 105 to 107 Hz, suggesting that the dynamic motion of dipoles cannot keep up with the quick switching of the applied electrical field at higher frequencies. The quite small ε′′ values of dielectric loss (<0.04) demonstrate the low inherent thermal dissipation of 1, which can improve its working stability.43,44 A significant frequency dispersion of the dielectric loss is observed in the temperature region below Tc, that is, the increasing of Tc at higher frequency. In contrast to the ε′–T trace, the frequency dispersion becomes more prominent in the dielectric loss plot; this is the characteristic feature of dielectric relaxation behavior. Generally, this relaxation process of dielectric properties dependent on the applied frequency is closely related to the molecular dipole motions or ionic displacements. Considering the order–disorder structural changes of organic i-BA+ and FA+ cations during the phase transition, it is reasonable to believe that their dipolar motions result in the dielectric relaxation behavior, which would be greatly favorable for the low-loss energy storage of 1.45–47
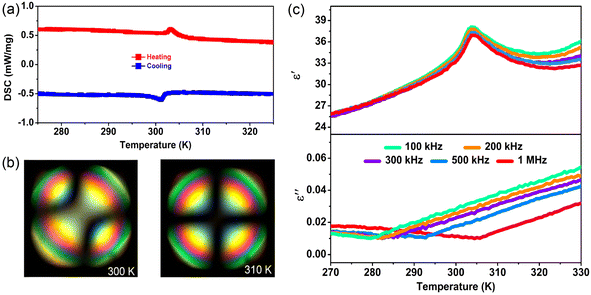 |
| Fig. 3 Phase transition properties of 1. (a) DSC curves of 1 measured in the heating and cooling runs. (b) Conoscopic images of the biaxial and uniaxial nematic phases at 300 and 310 K, respectively. (c) The real part (ε′) and imaginary part (ε′′) of the complex dielectric constant measured along the crystallographic c-axis direction at different frequencies upon heating. | |
Antiferroelectric properties.
Generally, the key feature of AFE materials is that the antiparallel arrangement of adjacent dipoles in the sublattices causes zero Ps (antipolar state) under the zero electric field. These molecular dipoles can be reversibly reversed and switched to be parallel under a sufficiently strong external field. This evolution process represents the electric field-triggered AFE-to-FE phase transition (phase switching) and is typically identified by the double P–E hysteresis loops. As shown in Fig. 4a, the polarization response of 1 to the electric field is linear at 305 K in the HTP, which is similar to that of ordinary dielectric materials. With the temperature just decreasing below Tc, the typical double P–E hysteresis loops become conspicuous, showing the assured proof of antiferroelectricity. The Pmax value is calculated to be ∼3.7 μC cm−2 under a low electric field of 41 kV cm−1 at room temperature, and the P–E loops change to be slightly thinner with the increasing of temperature. Such low electric fields guarantee the reliability of operation, thus, providing the possibility of high-level integration of miniaturized electronic devices. In addition, this Ps value is on par with those of some other molecular AFE compounds, such as 2-difluoromethylbenzimidazole (∼6 μC cm−2),13 (BA)2(EA)2Pb3I10 (∼5.6 μC cm−2),15 (3-pyrrolinium)CdBr3 (∼5.6 μC cm−2),16 2-trifluoromethylbenzimidazole (∼5.9 μC cm−2),18etc. From a structural viewpoint, the generation of polarization closely involves the dynamic ordering and antiparallel reorientations of FA+ and i-BA+ cations. The low Ps result of 1 may be caused by the inherent symmetry and orientation of the FA+ cations, being similar to those of the 2D hybrid perovskite ferroelectric (BA)2(FA)Pb2Br7 (∼3.8 μC cm−2).57Fig. 4b illustrates the representative current–field (J–E) traces of 1. Clearly, there are sharp peaks of current observed during the electric-induced phase transitions between the AFE and FE states. The current density exhibits a temperature-dependent behavior, which coincides well with that of the Ps–T variation. The double P–E hysteresis loops and J–E traces present the frequency stability over the frequency range of 40–100 Hz (Fig. S4†). Furthermore, we study the fatigue endurance of antiferroelectric materials. Fig. 4c shows that Ps of 1 remains unchanged after 2 × 105 switching operation cycles at low field and room temperature conditions. Such an excellent fatigue characteristic makes 1 a promising candidate toward the possible application in miniaturized energy storage devices. Besides, the forward switching field (EF, AFE-to-FE transition) and backward switching field (EA, FE-to-AFE transition) were also acquired from these J–E traces of 1. Fig. 5a presents the evolution of EF, EA, and the field hysteresis ΔE (ΔE = EF − EA), all of which decrease monotonically with the increasing temperature, and the ΔE is estimated to be 4.2 kV cm−1 at 302 K. As far as we know, this ΔE value for 1 is much smaller than that for other hybrid AFEs and inorganic AFE ceramics, such as (i-BA)2CsPb2Br7 (∼47 kV cm−1),14 Ag0.94La0.02NbO3 (∼75 kV cm−1),48 (Pb0.95Sr0.05)ZrO3 (∼190 kV cm−1),49etc. This unique advantage would effectively reduce the energy loss and probably cause remarkable energy storage efficiency (η).
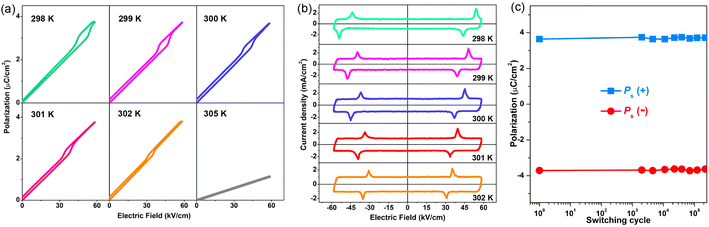 |
| Fig. 4 The AFE properties of 1. (a) The P–E hysteresis loops and (b) corresponding J–E curves. (c) The fatigue endurance of 1 after ∼2 × 105 switching cycles under low field and room temperature conditions. | |
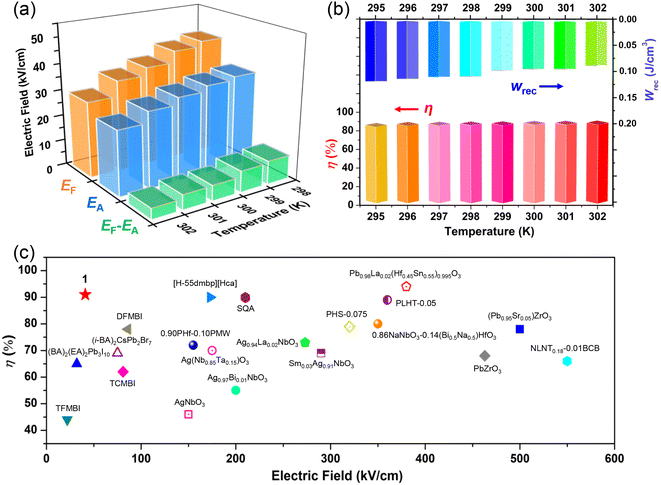 |
| Fig. 5 (a) EF, EA, and ΔE obtained at different temperatures. (b) Temperature dependence of energy density Wrec and storage efficiency η. (c) Energy storage efficiency (η) for various reported antiferroelectrics at room temperature. | |
Energy storage properties.
All the above studies confirm that 1 is a room-temperature molecule-based AFE material in the family of 2D hybrid perovskites. The energy storage efficiency (η) and recoverable energy density (Wrec) are two important figures-of-merit to appraise the energy storage performances for practical applications. Here, we have studied temperature dependence of η and Wrec of 1, as deduced from its double P–E loops with the following equations: η = Wrec/(Wrec + Wloss) and
, where E is the applied electric field, Pr and Pmax are the remnant polarization and maximum polarization, respectively, and Wloss represents energy loss (green areas in Fig. S5†). At room temperature, the η and Wrec values are estimated to be ∼89% and 0.13 J cm−3 from the double P–E hysteresis loop. On increasing the temperature, a satisfactory thermal stability has been confirmed, with the variations of both Wrec and η being less than 5%. It is fascinating that the temperature dependence of η shows a gradual increase up to ∼91% at 302 K, due to the decrease of Wloss caused by the shrinking of P–E loops (Fig. 5b). From the perspective of practical application, the frequency dependence of energy storage performances is also of great significance. With the frequency decreasing from 100 to 40 Hz (at 302 K, 41 kV cm−1), the Pmax value slightly decreases, while the ΔE increases (Fig. S4†). This indicates the energy dissipation of 1 remains at a low level, thus accounting for the stability of Wrec and η (Fig. S6†). As far as we know, this energy storage efficiency is even higher than that of the majority of inorganic AFE ceramics, such as Hf0.3Zr0.7O2 (η ∼ 50%),50 PHS-0.075 (η ∼ 79%),51 and PLHT-0.05 (η ∼ 89%) (Fig. 5c and Table S5†).52 The high energy storage efficiency signifies the low energy dissipation, which will less easily transform into thermal energy, thus drastically promoting its energy storage capacity. Such an ultrahigh energy efficiency at room temperature, together with the low critical fields, demonstrates the great potential of 1 for the application of energy storage with high practicability. In terms of improving the Wrec, the chemical design of organic cations, such as H/D substitution, plays an important role in increasing the Tc and the Ps of molecular ferroelectrics, which provides a hint for the realization of high-performance molecular antiferroelectric candidates.53 Besides, ion doping, including halogen anions or skeleton cations, may be an effective approach to increase the Wrec due to the introduction of relaxor characteristics.45
Origin of energy storage performance.
To better understand the spacer cation arrangement that controls the tendency toward ferroelectric or AFE ordering, we have taken the typical examples of (n-BA)2(FA)Pb2Br7 and (i-BA)2(FA)Pb2Br7 (1) for a detailed analysis. It is understood that the length and bulkiness of spacer cations play an important role in the formation of 2D perovskite frameworks, described by the distance between two adjacent inorganic sheets. As depicted in Fig. 6a, this plane distance is estimated to be ∼6.8 Å for 1, while the corresponding value for (n-BA)2(FA)Pb2Br7 containing the linear n-BA+ cation is ∼7.5 Å (Fig. S7a†). Hence, the reduction of space distance between two adjacent inorganic perovskite layers for 1 suggests the stronger confinement effects imposed by the rigid scaffold, which may probably influence the competition between their dipolar interactions and steric hindrance. That is, smaller distance between the adjacent parallel dipoles leads to more significant steric repulsions. As shown in Fig. S7b,† since the bulky tails of i-BA+ cations raise the steric hindrance, the average distance between the neighboring organic layers is about 3.85 Å, which becomes shorter by approximately 13% than that of n-BA+ (∼4.41 Å), revealing a stronger geometric constraint in 1. Consequently, the antipolar arrangement of structural moieties is also influenced by steric repulsions, which facilitate their stable AFE crystalline sublattices with the antiparallel array (Fig. S8†). Such findings demonstrate that our strategy of incorporating the branched-chain alkylamine i-BA into the interlayered space of 2D hybrid perovskites is viable for the generation of antiferroelectric order.
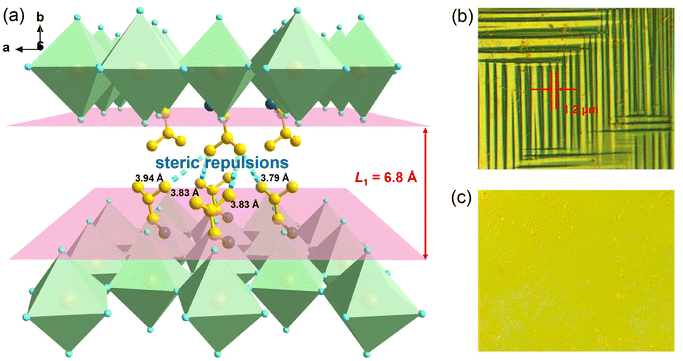 |
| Fig. 6 (a) The interlayered space and atomic distance between the adjacent layers of organic i-BA+ cations in 1. Time evolution of domain patterns observed at different temperatures: (b) 300 K and (c) 310 K. | |
Further, we employed polarized light microscopy techniques to investigate the domain structure of 1, in order to better understand the microscopic origin of its excellent energy storage performance. Under perpendicular polarized light, the domains of different orientations possess different birefringence properties, thus exhibiting dark and bright patterns. As depicted in Fig. 6b and c, the crystalline sheets of 1 exhibit two types of periodic stripe domain walls with an average width ≈1.2 μm in the LTP, which are almost perpendicular to each other. In general, such domains of micrometer scale decrease the polarization hysteresis between the AFE–FE and FE–AFE phase transitions owing to the fast response of microscale domains to the applied electric field, induced by the weak interatomic interactions between the microdomain clusters.46,54–56 Consequently, a slim hysteresis loop with high energy storage efficiency can be acquired by regulating the domain size to the micrometer scale in AFEs. As the temperature increases to the HTP, the domain structures slowly disappear, corresponding to its paraelectric state. Subsequently, as the temperature cools down to the LTP, the crystalline sheet transforms back into the ferroelastic phase with the reconstructed domain structures. Such a temperature dependence of the domain patterns also coincides well with the AFE-to-paraelectric phase transition of 1. Furthermore, it is known that the formation of solid solutions with relaxor compounds is an efficiency-enhancement strategy for antiferroelectric ceramics with relaxor characteristics, as demonstrated in some inorganic antiferroelectric counterparts. The lattice distortion and reduction in the grain size are also observed for relaxor compound additions, which will lead to changes in the domain structure and size. It is proposed that nanosized domains characteristic of relaxor-antiferroelectrics can be achieved in 2D hybrid perovskite materials through constructing solid solutions using halogen doping methods.46,53
Conclusions
In summary, we have successfully designed a new 2D hybrid perovskite-type molecular AFE, (i-BA)2(FA)Pb2Br7, which demonstrates an ultrahigh energy storage efficiency under low electric fields at room temperature. Structurally, the dynamic ordering and antiparallel reorientation of organic dipoles induce its AFE-type phase transition at 303 K along with a large Ps of ∼3.7 μC cm−2. It is noteworthy that the antipolar sublattices of 1 have been stabilized through raising steric hindrance of organic i-BA+ spacer cations. Most strikingly, the characteristic double hysteresis loops of 1 allow a high energy storage efficiency ∼91% at a quite low Ecr ∼41 kV cm−1. This Ecr value is much lower than those of state-of-the-art AFE oxides, revealing its great potential for the miniaturization of energy-storage devices. This work sheds light on exploration of new AFE candidates toward practical applications.
Author contributions
Y. L. prepared the samples, measured the antiferroelectric properties, and wrote the manuscript. H. J. X., X. T. L., and S. G. H. determined the structures. W. Q. G., Y. M., Q. S. F., and X. X. H. provided suggestions for research. Z. H. S. and J. H. L. designed and directed the studies. All authors contributed to writing and reviewing the manuscript.
Conflicts of interest
The authors declare no conflict of interest.
Acknowledgements
This work was supported by NSFC (22125110, 21875251, 21833010, 22205233, 22193042, 22122507, 21921001, and U21A2069), the Key Research Program of Frontier Sciences of the Chinese Academy of Sciences (ZDBS-LY-SLH024), the Natural Science Foundation of Fujian Province (2021J01523 and 2020J01112), Fujian Science & Technology Innovation Laboratory for Optoelectronic Information of China (2021ZR126), the Youth Innovation Promotion of CAS (2019301, Y202069, and 2020307), the National Postdoctoral Program for Innovative Talents (BX2021315), the National Key Research and Development Program of China (2019YFA0210402), and China Postdoctoral Science Fund (2022TQ0337).
Notes and references
- K. J. Griffith, K. M. Wiaderek, G. Cibin, L. E. Marbella and C. P. Grey, Nature, 2018, 559, 556–563 CrossRef CAS PubMed.
- M. F. El-Kady, V. Strong, S. Dubin and R. B. Kaner, Science, 2012, 335, 1326–1330 CrossRef CAS PubMed.
- E. Pomerantseva and Y. Gogotsi, Nat. Energy, 2017, 2, 17089 CrossRef CAS.
- C. Kittel, Phys. Rev., 1951, 82, 729–732 CrossRef CAS.
-
R. Blinc and B. Zeks, Soft Mode in Ferroelectrics and Antiferroelectrics, North-Holland, 1974 Search PubMed.
- Z. Liu, T. Lu, J. Ye, G. Wang, X. Dong, R. Withers and Y. Liu, Adv. Mater. Technol., 2018, 3, 1800111 CrossRef.
- P. Tolédano and M. Guennou, Phys. Rev. B, 2016, 94, 014107 CrossRef.
- X. Hao, J. Adv. Dielectr., 2013, 3, 1330001 CrossRef.
- J. Qi, N. Ma and Y. Yang, Adv. Mater. Interfaces, 2018, 5, 1701189 CrossRef.
- J. Wei, T. Yang and H. Wang, J. Eur. Ceram. Soc., 2019, 39, 624–630 CrossRef CAS.
- N. Ma and Y. Yang, Nano Energy, 2017, 40, 352–359 CrossRef CAS.
- S. Horiuchi and S. Ishibashi, J. Phys. Soc. Jpn., 2020, 89, 051009 CrossRef.
- S. Horiuchi, R. Kumai and S. Ishibashi, Chem. Sci., 2018, 9, 425–432 RSC.
- Z. Wu, X. Liu, C. Ji, L. Li, S. Wang, Y. Peng, K. Tao, Z. Sun, M. Hong and J. Luo, J. Am. Chem. Soc., 2019, 141, 3812–3816 CrossRef CAS PubMed.
- S. Han, X. Liu, Y. Liu, Z. Xu, Y. Li, M. Hong, J. Luo and Z. Sun, J. Am. Chem. Soc., 2019, 141, 12470–12474 CrossRef CAS PubMed.
- P.-F. Li, W.-Q. Liao, Y.-Y. Tang, H.-Y. Ye, Y. Zhang and R.-G. Xiong, J. Am. Chem. Soc., 2017, 139, 8752–8757 CrossRef CAS PubMed.
- S. Horiuchi and S. Ishibashi, Chem. Sci., 2021, 12, 14198–14206 RSC.
- S. Horiuchi, F. Kagawa, K. Hatahara, K. Kobayashi, R. Kumai, Y. Murakami and Y. Tokura, Nat. Commun., 2012, 3, 1308 CrossRef PubMed.
- T. Fukami, S. Akahoshi, K. Hukuda and T. Yagi, J. Phys. Soc. Jpn., 1989, 58, 3429–3430 CrossRef CAS.
- H. Xu, W. Guo, J. Wang, Y. Ma, S. Han, Y. Liu, L. Lu, X. Pan, J. Luo and Z. Sun, J. Am. Chem. Soc., 2021, 143, 14379–14385 CrossRef CAS PubMed.
- J. N. Wilson, J. M. Frost, S. K. Wallace and A. Walsh, APL Mater., 2019, 7, 010901 CrossRef.
- G. Grancini, A. R. S. Kandada, J. M. Frost, A. J. Barker, M. D. Bastiani, M. Gandini, S. Marras, G. Lanzani, A. Walsh and A. Petrozza, Nat. Photonics, 2015, 9, 695–701 CrossRef CAS PubMed.
- A. Walsh, D. O. Scanlon, S. Chen, X. G. Gong and S.-H. Wei, Angew. Chem., 2015, 127, 1811–1814 CrossRef.
- L. Dou, A. B. Wong, Y. Yu, M. Lai, N. Kornienko, S. W. Eaton, A. Fu, C. G. Bischak, J. Ma, T. Ding, N. S. Ginsberg, L.-W. Wang, A. P. Alivisatos and P. Yang, Science, 2015, 349, 1518–1521 CrossRef CAS PubMed.
- J. Byun, H. Cho, C. Wolf, M. Jang, A. Sadhanala, R. H. Friend, H. Yang and T.-W. Lee, Adv. Mater., 2016, 28, 7515–7520 CrossRef CAS PubMed.
- H. Tsai, C. Liu, E. Kinigstein, M. Li, S. Tretiak, M. Cotlet, X. Ma, X. Zhang and W. Nie, Adv. Sci., 2020, 7, 1903202 CrossRef CAS PubMed.
- G. Grancini, C. Roldán-Carmona, I. Zimmermann, E. Mosconi, X. Lee, D. Martineau, S. Narbey, F. Oswald, F. De Angelis, M. Graetzel and M. K. Nazeeruddin, Nat. Commun., 2017, 8, 15684 CrossRef CAS PubMed.
- T. Zhou, H. Lai, T. Liu, D. Lu, X. Wan, X. Zhang, Y. Liu and Y. Chen, Adv. Mater., 2019, 31, 1901242 CrossRef PubMed.
- C. Qin, A. S. D. Sandanayaka, C. Zhao, T. Matsushima, D. Zhang, T. Fujihara and C. Adachi, Nature, 2020, 585, 53–57 CrossRef CAS PubMed.
- L. Lei, D. Seyitliyev, S. Stuard, J. Mendes, Q. Dong, X. Fu, Y.-A. Chen, S. He, X. Yi, L. Zhu, C.-H. Chang, H. Ade, K. Gundogdu and F. So, Adv. Mater., 2020, 32, 1906571 CrossRef CAS PubMed.
- S. W. Eatona, M. Laia, N. A. Gibson, A. B. Wong, L. Dou, J. Ma, L.-W. Wang, S. R. Leone and P. Yang, Proc. Natl. Acad. Sci. U. S. A., 2016, 113, 8 CrossRef PubMed.
- L. Mao, C. C. Stoumpos and M. G. Kanatzidis, J. Am. Chem. Soc., 2019, 141, 1171–1190 CrossRef CAS PubMed.
- C. Katan, N. Mercier and J. Even, Chem. Rev., 2019, 119, 3140–3192 CrossRef CAS PubMed.
- Y. Liu, Z. Wu, X. Liu, S. Han, Y. Li, T. Yang, Y. Ma, M. Hong, J. Luo and Z. Sun, Adv. Opt. Mater., 2019, 7, 1901049 CrossRef CAS.
- Y. Liu, J. Wang, S. Han, X. Liu, M. Li, Z. Xu, W. Guo, M. Hong, J. Luo and Z. Sun, Chem.–Eur. J., 2020, 26, 3494–3498 CrossRef CAS PubMed.
- J. Hu, L. Yan and W. You, Adv. Mater., 2018, 30, 1802041 CrossRef PubMed.
- X.-G. Chen, X.-J. Song, Z.-X. Zhang, H.-Y. Zhang, Q. Pan, J. Yao, Y.-M. You and R.-G. Xiong, J. Am. Chem. Soc., 2020, 142, 10212–10218 CrossRef CAS PubMed.
- Y. Ma, J. Wang, W. Guo, S. Han, J. Xu, Y. Liu, L. Lu, Z. Xie, J. Luo and Z. Sun, Adv. Funct. Mater., 2021, 31, 2103012 CrossRef CAS.
- Y. Liu, X. Pan, X. Liu, S. Han, J. Wang, L. Lu, H. Xu, Z. Sun and J. Luo, Small, 2022, 18, 2106888 CrossRef CAS PubMed.
- K. Takaea and H. Tanaka, Proc. Natl. Acad. Sci. U. S. A., 2018, 115, 9917–9922 CrossRef PubMed.
- J. M. Luttinger and L. Tisza, Phys. Rev., 1946, 70, 954–964 CrossRef CAS.
- C. S. Zehe, J. A. Hill, N. P. Funnell, K. Kreger, K. P. van der Zwan, A. L. Goodwin, H.-W. Schmidt and J. Senker, Angew. Chem., Int. Ed., 2017, 56, 4432–4437 CrossRef CAS PubMed.
- V. L. Gurevich and A. K. Tagantsev, Adv. Phys., 1991, 40, 719–767 CrossRef CAS.
- Z. Yao, Z. Song, H. Hao, Z. Yu, M. Cao, S. Zhang, M. T. Lanagan and H. Liu, Adv. Mater., 2017, 29, 1601727 CrossRef PubMed.
- F. Li, S. Zhang, D. Damjanovic, L.-Q. Chen and T. R. Shrout, Adv. Funct. Mater., 2018, 28, 1801504 CrossRef.
- J. Li, F. Li, Z. Xu and S. Zhang, Adv. Mater., 2018, 30, 1802155 CrossRef PubMed.
- P. Mohapatra, Z. Fan, J. Cui and X. Tan, J. Eur. Ceram. Soc., 2019, 39, 4735–4742 CrossRef CAS.
- J. Gao, Y. Zhang, L. Zhao, K.-Y. Lee, Q. Liu, A. Studer, M. Hinterstein, S. Zhang and J.-F. Li, J. Mater. Chem. A, 2019, 7, 2225–2232 RSC.
- X. Hao, J. Zhai and X. Yao, J. Am. Ceram. Soc., 2009, 92, 1133–1135 CrossRef CAS.
- M. H. Park, H. J. Kim, Y. J. Kim, T. Moon, K. D. Kim and C. S. Hwang, Adv. Energy Mater., 2014, 4, 1400610 CrossRef.
- P.-Z. Ge, X.-G. Tang, K. Meng, X.-X. Huang, S.-F. Li, Q.-X. Liu and Y.-P. Jiang, Chem. Eng. J., 2022, 429, 132540 CrossRef CAS.
- P.-Z. Ge, X.-G. Tang, K. Meng, X.-X. Huang, Q.-X. Liu, Y.-P. Jiang, W.-P. Gong and T. Wang, Mater. Today Phys., 2022, 24, 100681 CrossRef CAS.
- H.-Y. Liu, H.-Y. Zhang, X.-G. Chen and R.-G. Xiong, J. Am. Chem. Soc., 2020, 142, 15205–15218 CrossRef CAS PubMed.
- H. Qi, R. Zuo, A. Xie, A. Tian, J. Fu, Y. Zhang and S. Zhang, Adv. Funct. Mater., 2019, 29, 1903877 CrossRef.
- Q. Zhang, H. Tong, J. Chen, Y. Lu, T. Yang, X. Yao and Y. He, Appl. Phys. Lett., 2016, 109, 262901 CrossRef.
- G. Wang, J. Li, X. Zhang, Z. Fan, F. Yang, A. Feteira, D. Zhou, D. C. Sinclair, T. Ma, X. Tan, D. Wang and I. M. Reaney, Energy Environ. Sci., 2019, 12, 582–588 RSC.
- L. Li, X. Shang, S. Wang, N. Dong, C. Ji, X. Chen, S. Zhao, J. Wang, Z. Sun, M. Hong and J. Luo, J. Am. Chem. Soc., 2018, 140, 6806–6809 CrossRef CAS PubMed.
|
This journal is © The Royal Society of Chemistry 2022 |