DOI:
10.1039/D2SC02637F
(Edge Article)
Chem. Sci., 2022,
13, 10773-10778
Molecular nanoribbon gels†
Received
11th May 2022
, Accepted 5th August 2022
First published on 8th August 2022
Abstract
Herein, we show that twisted molecular nanoribbons with as many as 322 atoms in the aromatic core are efficient gelators capable of self-assembling into ordered π-gels with morphologies and sol–gel transitions that vary with the length of the nanoribbon. In addition, the nanoribbon gels show a red fluorescence and also pseudoconductivity values in the same range as current state-of-the-art π-gels.
Introduction
π-Gels1 are the result of the spontaneous aggregation of π-conjugated systems into coiled fibrils. Notably, π-gels offer a combination of properties not found in other organic materials. For example, the spontaneous and reversible organization of π-gels can be used to prepare self-assembled materials for optoelectronic applications.1a,2 In addition, π-gels can be healed by taking advantage of the reversibility of the sol–gel transition. Also, given their fluidity and their soft nature, π-gels can be processed by liquid deposition methods and can be used to fabricate flexible devices.
Polycyclic aromatic hydrocarbons have attracted a lot of attention as gelators1d–f,2p since their extended π-surface favours π-stacking, which in turn, enables the formation of optimal channels for electronic and excitonic transport. The use of molecular nanographenes as gelators can potentially increase the performance of π-gels because of their larger π-surfaces. Even if there has been an increasing effort in the synthesis of giant nanographenes with >150 atoms in their aromatic core,3 current state-of-the-art nanographene gelators are constituted of C24 (ref. 4) and C68 (ref. 5) cores. This illustrates that incorporation of nanographenes in π-gels is a challenging task, mostly because of the large dimensions and monodisperse nature of nanographenes that favour the typical precipitation/crystallization of the gelator from the gel phase.1d,e Another aspect to consider is the planarity of the nanographene core. Although most nanographene gelators are planar,4 it has been recently shown that negatively curved nanographenes can also behave as an efficient gelators.5
In 2021, some of us reported the synthesis of a family of giant molecular nanoribbons (NRs) with C64N8S2, C180N16S2 and C296N24S2 cores (respectively, NR-13, NR-33 and NR-53, Fig. 1),3n constituted by pyrene, coronenebisimide, pyrazinoquinoxaline and quinoxalinothiadiazol units. These NRs combine a selection of solubilizing groups with a twisted aromatic framework that endows them with a high solubility. The NR backbone is twisted 34° at each coronene–pyrene junction as the result of steric congestion between the hydrogens at such cove regions (Fig. 2).
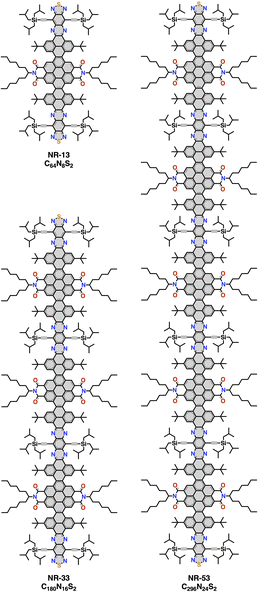 |
| Fig. 1 Chemical structures of NR-13, NR-33 and NR-53. The chemical formula corresponds only to the aromatic core atoms highlighted in grey. | |
 |
| Fig. 2 Different front and side views of a NR-53 model3n without solubilizing groups illustrating its twisted structure. | |
Herein, we show that despite their large dimensions and their twisted structure NR-13, NR-33 and NR-53 are efficient gelators that are able to self-assemble in 1-octanol at concentrations <3% to produce gels with morphologies that vary with the length of the NR. In addition, the NR π-gels show a red fluorescence and also pseudoconductivity values in the same range as current state-of-the-art π-gels.
Results and discussion
Gelation studies
The gelation and aggregation abilities of NR-13, NR-33 and NR-53 were studied in 1-butanol, 1-octanol and nitrobenzene. The criterion for the gel formation was the vial inverting method at room temperature by checking whether the sample flows. We found that the three NRs were suitable organogelators in 1-octanol, whereas in nitrobenzene only NR-13 and NR-33 were able to form gels. The three NRs produced opaque purple gels in 1-octanol upon cooling to room temperature in air. The gels exhibit reversible sol to gel phase transitions and are stable at room temperature for months. The minimum gelation concentration in 1-octanol for NR-13 is 3.0% (w/w), while for NR-33 and NR-53 is 1.3% (w/w). Below these concentrations, the NRs form aggregates that cannot retain the solvent.
Microscopy
Microscopy investigations reveal that the morphology of the gels depends directly on the length of the NR. The morphology of the dried gels (xerogels) was examined by scanning electron microscopy (SEM). The SEM images acquired from the xerogels of NR-13 (Fig. 3a and S1†) clearly showed rigid long fibrils, which exhibit lengths up to tens of μm and in some cases even longer. These fibrils show widths ranging from 0.40 to 2.5 μm, but they also vary in height (Fig. S1b†). The aggregates of NR-13 in 1-octanol at lower concentration (0.07% w/w), in which the gelation does not take place, were studied by transmission electron microscopy (TEM), showing shorter fibrils but in the same range of width (Fig. 3d). For NR-33, the SEM images of the xerogels showed shorter and thinner fibrils (Fig. 3b and S2†) than the ones of NR-13. These NR-33 fibrils are homogeneous in width (200–250 nm) and show lengths of several μm. The same trend was observed by TEM (0.1% w/w), with thin fibrils which possess a length of 1–2 μm and widths of hundreds of nanometers (Fig. 3e). In contrast to the xerogels of NR-13 and NR-33, the SEM images show that the xerogel of NR-53 is constituted by homogeneous spherical particles of 25–29 nm of diameter (Fig. 3c and S3†) that aggregate forming fibrils of several μm. In the case of NR-53 (0.1% w/w in 1-octanol), the TEM images revealed a different morphology than the corresponding xerogel. In fact, thin flexible fibrils (Fig. 3f) with widths up to 180 nm and lengths of several μm were observed.
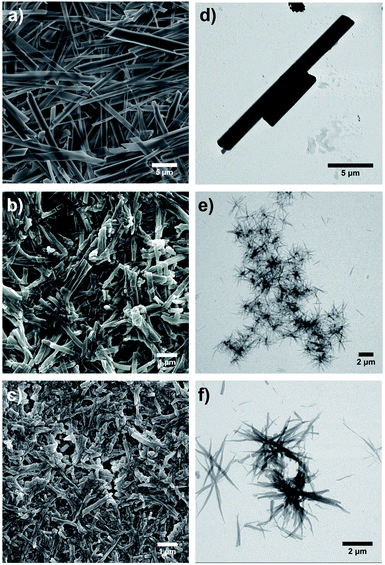 |
| Fig. 3 SEM images of the xerogels, in 1-octanol, of (a) NR-13 (3% w/w), (b) NR-33 (1.3% w/w), and (c) NR-53 (1.3% w/w). TEM images of the aggregates in 1-octanol of (d) NR-13 (0.07% w/w), (e) NR-33 (0.1% w/w), and (f) NR-53 (0.1% w/w). | |
X-ray diffraction
The crystallinity of the xerogels of NR-13, NR-33 and NR-53 was confirmed by powder X-ray diffraction (PXRD), which showed several intense diffraction peaks with a similar pattern (Fig. 4). The xerogel of NR-13 exhibits a pattern of sharp signals, indicating a high degree of crystallinity (Fig. 4a), which differs from the single crystal structure reported.3n The xerogels of the longer NR-33 and NR-53, show a similar pattern of broader peaks (Fig. 4b and c). In the case of NR-33 and NR-53 the d-spacings are consistent a lamellar packing. Indexing in both cases corresponds to those shown in Fig. 4 or to multiples of these. The broadening can be ascribed to the fact that the original gels of the larger nanoribbons were more viscous and retained more solvent.
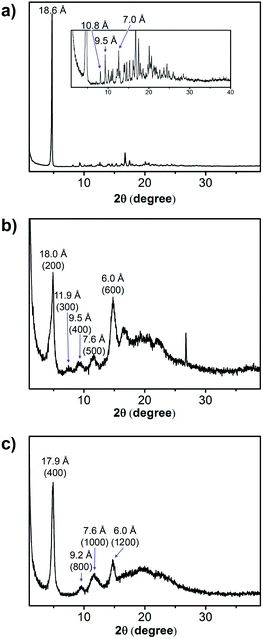 |
| Fig. 4 X-Ray powder diffraction pattern from the xerogels obtained from the 1-octanol gels of (a) NR-13 (3.0% w/w); (b) NR-33 (1.3% w/w); and (c) NR-53 (1.3% w/w). | |
Rheology
Rheological experiments were carried out in order to confirm the gel nature of the samples (Fig. 5). Small Amplitude Oscillatory Shear (SAOS) experiments at 20 °C showed G′ > G′′ values that are frequency-independent, which correspond to a percolated network structure. To investigate the thixotropic behaviour, we subjected the samples to shear rate ramps (increase-steady-decrease). The samples showed a marked pseudoplastic behaviour, but after shearing, the samples do not recover their initial gel-like structure as the viscosity remains constant and low upon shearing as illustrated for NR-53 (Fig. S4†). This effect, that can be ascribed to the orientation of the nanoribbons in the flow direction, was further investigated by large amplitude oscillatory shear (LAOS) experiments. The results (Fig. S5 and S6†) indicate that the supramolecular assembly loses its elastic property at high strain values, as G′′ > G′ was observed, indicating the rupture of the gel network. Similarly, continuous step–strain cycles at low (0.5%) and high (100%) strain showed that, once the strain is released, a weak structural rearrangement occurs, as the elastic property is restored again (G′ > G′′), although the moduli were not fully recovered and the sol–gel process was only partially reversed.
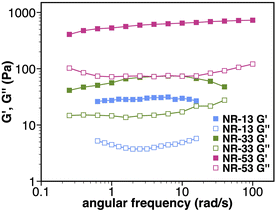 |
| Fig. 5 Rheological characterization. Frequency sweep test at T = 20 °C of NR-13 (3% w/w), NR-33 (1.3% w/w), and NR-53 (1.3% w/w). | |
The rheological behaviour correlates with the different morphologies of the gels. The thin, flexible fibrils of the NR-53 gel lead to the formation of a denser and more entangled nanofibrous structure with higher moduli than the stiffer fibers of NR-33 and NR-13 gels, which, as expected, result in a network with poorer connectivity. Therefore, the flexibility of the fibers increases the junction number density, improving the macroscopic viscoelastic response. This is consistent with previous observations on pyrene-based supramolecular gels, where the nanoscale flexibility of supramolecular packing was suggested to strongly influence the mechanical strength of the gel.6
Sol–gel transition
The sol–gel transitions (Tsol–gel) of the different gels were estimated by monitoring visually the changes in the fluidity of the samples with the temperature. The measurements illustrate how the Tsol–gel of the different gels increase with the increasing length of the NR (48, 61 and 77 °C, respectively for NR-13, NR-33 and NR-53). Even if there is a clear correlation between NR length and Tsol–gel, a linear correlation cannot be established because of the different minimum gelation concentration of the gel of NR-13.
Optoelectronic properties
Since the gels are opaque, the absorption spectra could not be recorded either in the gel or xerogel state (Fig. 6d–f). Nevertheless, the gels show a red fluorescent emission in all cases upon exposure to UV light. Therefore, the emission spectra of a diluted 1-octanol solution, the sol state, and the xerogel state were recorded and compared (Fig. 6a–c). In 1-octanol solutions, the emission spectra evidence a vibronically-resolved fluorescence band with maxima at 618, 618 and 620 nm respectively for NR-13, NR-33 and NR-53, similar to the fluorescence spectra reported in chloroform.3n In the sol state (Fig. S7†), the above-mentioned fluorescence emission coexists with a much more intense and red shifted emission band with maxima at 693, 687 and 675 nm, respectively for NR-13, NR-33 and NR-53, which indicate π-stacking in agreement with previous observations on other nanographene gels with extended cores.4,5 In the xerogels, also a red shifted featureless band with a similar broadness but with different maxima at 703, 721 and 679 nm, respectively for NR-13, NR-33 and NR-53, can be observed, being also consistent with π-stacking.4,5 Excitation spectra of the xerogels were recorded upon excitation at the fluorescence maxima, showing the same trends observed in the fluorescence measurements (Fig. S8†).
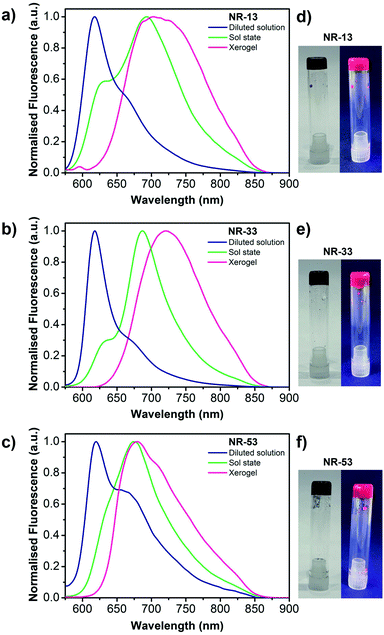 |
| Fig. 6 Fluorescence spectra of a diluted solution in 1-octanol, sol state and xerogel of (a) NR-13; (b) NR-33; and (c) NR-53. Gelation test in 1-octanol, under room light (left) and UV light (right), of: (d) NR-13 (3% w/w); (e) NR-33 (1.3% w/w); and (f) NR-53 (1.3% w/w). | |
Photoconductivity
The charge transport properties of the NR xerogels were assessed by flash-photolysis time-resolved microwave conductivity (FP-TRMC).7 FP-TRMC measures the pseudoconductivity (φΣμ, where φ is the product of the quantum yield, and Σμ is the sum of the charge carrier mobilities) directly from powder samples under an oscillating microwave electric field without the need of metal contacts. The φΣμ value is directly related to the minimum or inherent charge carrier mobility of the material. The measurements on the xerogels (Fig. 7) show very similar φΣμ maxima (φΣμmax) for NR-13 (0.25 × 10−4 cm2 V−1 s−1), NR-33 (0.14 × 10−4 cm2 V−1 s−1) and NR-53 (0.14 × 10−4 cm2 V−1 s−1). This φΣμmax values are in the same range as those of fullerenes,8 giant molecular nanoribbons,3e nanographenes,3i and undoped π-gels.2e,h,p The half lifetimes (τ1/2) extend beyond the ms in all cases. A likely rationale would be the formation of stable charge separated states that delay charge recombination, in agreement with previous electrochemical and theoretical studies3n that illustrate the generation of reversible radical-anions and the localization of two quasidegenerate LUMOs on each of the pyrazinobenzothiadiazole ends of the NRs.
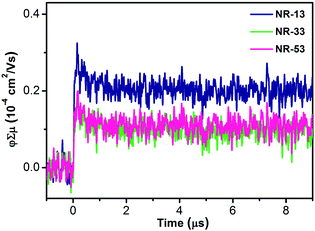 |
| Fig. 7 Conductivity transients of the xerogels of NR-13, NR-33 and NR-53 upon excitation at 355 nm, 9.1 × 1015 photons per cm2 per pulse. | |
Conclusions
To conclude we have shown that despite their twisted structure and their dimensions, giant molecular NRs can act as optimal gelators that produce ordered π-gels with morphologies that vary with the length of the NRs. Fluorescence spectroscopy evidences the existence of π–π stacking in the gels and also their red emitting properties. FP-TRMC shows that the NR gels display φΣμ values in the same range as state-of-the-art giant nanographenes3e,i and π-gels.2e,h,p Overall, this work opens up new possibilities for the organization of molecular nanoribbons into functional materials and their implementation into organic optoelectronic devices by low-cost and large-area liquid deposition methods.
Author contributions
M. M.-Ab. carried out the gelation experiments, the optical characterization and prepared the samples for SEM and TEM microscopy and for PXRD. R. K. D. carried out the synthesis of the nanoribbons. M. F. and R. A. carried out and interpret the rheology measurements. M. M.-Ar. established the sol–gel transitions. A. S. carried out the FP-TRMC measurements. A. M.-A. conceived and conceptualized the project. M. M.-Ab. and A. M.-A. co-wrote the manuscript. All authors further contributed to the discussion of the experimental work and the final version of the manuscript.
Conflicts of interest
The authors declare no conflict of interest.
Acknowledgements
This work was carried out with support from the Basque Science Foundation for Science (Ikerbasque), POLYMAT, the University of the Basque Country, Diputación de Guipúzcoa, Gobierno Vasco (PIBA_2022_1_0031 and BERC programme) and Gobierno de España (Projects PID2021-124484OB-I00 and CEX2020-001067-M financed by MCIN/AEI/10.13039/501100011033). Project (PCI2022-132921) funded by the Agencia Estatal de Investigación through the PCI 2022 and M-ERA.NET 2021 calls. Technical and human support provided by SGIker of UPV/EHU and European funding (ERDF and ESF) is acknowledged. This project has received funding from the European Research Council (ERC) under the European Union's Horizon 2020 research and innovation programme (Grant Agreement No. 722951). This project has received funding from the European Union's Horizon 2020 research and innovation programme under grant agreement No. 899895. This work was funded by the Εuropean Union under the Horizon Europe grant 101046231.
Notes and references
-
(a) Y. F. Yao, L. Zhang, E. Orgiu and P. Samori, Adv. Mater., 2019, 31, 1900599 CrossRef;
(b) F. Lu, E. A. Neal and T. Nakanishi, Acc. Chem. Res., 2019, 52, 1834–1843 CrossRef CAS PubMed;
(c) C. D. Jones and J. W. Steed, Chem. Soc. Rev., 2016, 45, 6546–6596 RSC;
(d) S. Ghosh, V. K. Praveen and A. Ajayaghosh, Annu. Rev. Mater. Res., 2016, 46, 235–262 CrossRef CAS;
(e) S. S. Babu, V. K. Praveen and A. Ajayaghosh, Chem. Rev., 2014, 114, 1973–2129 CrossRef CAS PubMed;
(f) S. S. Babu, S. Prasanthkumar and A. Ajayaghosh, Angew. Chem., Int. Ed., 2012, 51, 1766–1776 CrossRef CAS PubMed.
-
(a) J.-P. Hong, M.-C. Um, S.-R. Nam, J.-I. Hong and S. Lee, Chem. Commun., 2009, 310–312 RSC;
(b) Y.-S. Guan, Y. Qin, Y. Sun, J. Chen, W. Xu and D. Zhu, Chem. Commun., 2016, 52, 4648–4651 RSC;
(c) A. A. Sagade, K. V. Rao, U. Mogera, S. J. George, A. Datta and G. U. Kulkarni, Adv. Mater., 2013, 25, 559–564 CrossRef CAS PubMed;
(d) Y.-S. Guan, Y. Qin, Y. Sun, C. Wang, W. Xu and D. Zhu, Chem. Commun., 2015, 51, 12182–12184 RSC;
(e) S. Prasanthkumar, A. Saeki, S. Seki and A. Ajayaghosh, J. Am. Chem. Soc., 2010, 132, 8866–8867 CrossRef CAS PubMed;
(f) M. J. Hollamby, M. Karny, P. H. H. Bomans, N. A. J. M. Sommerdjik, A. Saeki, S. Seki, H. Minamikawa, I. Grillo, B. R. Pauw, P. Brown, J. Eastoe, H. Möhwald and T. Nakanishi, Nat. Chem., 2014, 6, 690 CrossRef CAS;
(g) J. López-Andarias, M. J. Rodriguez, C. Atienza, J. L. López, T. Mikie, S. Casado, S. Seki, J. L. Carrascosa and N. Martín, J. Am. Chem. Soc., 2015, 137, 893–897 CrossRef PubMed;
(h) V. S. Nair, R. D. Mukhopadhyay, A. Saeki, S. Seki and A. Ajayaghosh, Sci. Adv., 2016, 2, e1600142 CrossRef PubMed;
(i) C. Martín, K. Kennes, M. Van der Auweraer, J. Hofkens, G. de Miguel and E. M. García-Frutos, Adv. Funct. Mater., 2017, 27, 1702176 CrossRef;
(j) E. R. Draper, J. J. Walsh, T. O. McDonald, M. A. Zwijnenburg, P. J. Cameron, A. J. Cowan and D. J. Adams, J. Mater. Chem. C, 2014, 2, 5570–5575 RSC;
(k) W.-W. Tsai, I. D. Tevis, A. S. Tayi, H. Cui and S. I. Stupp, J. Phys. Chem. B, 2010, 114, 14778–14786 CrossRef CAS PubMed;
(l) D. A. Stone, A. S. Tayi, J. E. Goldberger, L. C. Palmer and S. I. Stupp, Chem. Commun., 2011, 47, 5702–5704 RSC;
(m) J. Xu, Y. Wang, H. Shan, Y. Lin, Q. Chen, V. A. L. Roy and Z. Xu, ACS Appl. Mater. Interfaces, 2016, 8, 18991–18997 CrossRef CAS;
(n) L. Zhang, S. Li, M. A. Squillaci, X. Zhong, Y. Yao, E. Orgiu and P. Samorì, J. Am. Chem. Soc., 2017, 139, 14406–14411 CrossRef CAS PubMed;
(o) K. Besar, H. A. M. Ardoña, J. D. Tovar and H. E. Katz, ACS Nano, 2015, 9, 12401–12409 CrossRef CAS PubMed;
(p) M. Martinez-Abadia, G. Antonicelli, A. Saeki and A. Mateo-Alonso, Angew. Chem., Int. Ed., 2018, 57, 8209–8213 CrossRef CAS PubMed.
-
(a) X. Guo, Z. Yuan, Y. Zhu, Z. Li, R. Huang, Z. Xia, W. Zhang, Y. Li and J. Wang, Angew. Chem., Int. Ed., 2019, 58, 16966–16972 CrossRef CAS PubMed;
(b) Y. Zhu, Z. Xia, Z. Cai, Z. Yuan, N. Jiang, T. Li, Y. Wang, X. Guo, Z. Li, S. Ma, D. Zhong, Y. Li and J. Wang, J. Am. Chem. Soc., 2018, 140, 4222–4226 CrossRef CAS PubMed;
(c) S. Ma, J. Gu, C. Lin, Z. Luo, Y. Zhu and J. Wang, J. Am. Chem. Soc., 2020, 142, 16887–16893 CrossRef CAS PubMed;
(d) C. M. Cruz, I. R. Márquez, S. Castro-Fernández, J. M. Cuerva, E. Maçôas and A. G. Campaña, Angew. Chem., Int. Ed., 2019, 58, 8068–8072 CrossRef CAS PubMed;
(e) D. Cortizo-Lacalle, J. P. Mora-Fuentes, K. Strutyński, A. Saeki, M. Melle-Franco and A. Mateo-Alonso, Angew. Chem., Int. Ed., 2018, 57, 703–708 CrossRef CAS PubMed;
(f) X. Yan, X. Cui and L.-s. Li, J. Am. Chem. Soc., 2010, 132, 5944–5945 CrossRef CAS PubMed;
(g) Y. Chen, C. Lin, Z. Luo, Z. Yin, H. Shi, Y. Zhu and J. Wang, Angew. Chem., Int. Ed., 2021, 60, 7796–7801 CrossRef CAS;
(h) Y. Wang, Z. Yin, Y. Zhu, J. Gu, Y. Li and J. Wang, Angew. Chem., Int. Ed., 2019, 58, 587–591 CrossRef CAS PubMed;
(i) J. P. Mora-Fuentes, A. Riaño, D. Cortizo-Lacalle, A. Saeki, M. Melle-Franco and A. Mateo-Alonso, Angew. Chem., Int. Ed., 2019, 58, 552–556 CrossRef CAS PubMed;
(j) U. Beser, M. Kastler, A. Maghsoumi, M. Wagner, C. Castiglioni, M. Tommasini, A. Narita, X. Feng and K. Müllen, J. Am. Chem. Soc., 2016, 138, 4322–4325 CrossRef CAS PubMed;
(k) C. D. Simpson, J. D. Brand, A. J. Berresheim, L. Przybilla, H. J. Räder and K. Müllen, Chem.–Eur. J., 2002, 8, 1424–1429 CrossRef CAS PubMed;
(l) Y.-Z. Tan, B. Yang, K. Parvez, A. Narita, S. Osella, D. Beljonne, X. Feng and K. Müllen, Nat. Commun., 2013, 4, 2646 CrossRef PubMed;
(m) Y. Zhu, X. Guo, Y. Li and J. Wang, J. Am. Chem. Soc., 2019, 141, 5511–5517 CrossRef CAS PubMed;
(n) R. K. Dubey, M. Melle-Franco and A. Mateo-Alonso, J. Am. Chem. Soc., 2021, 143, 6593–6600 CrossRef CAS PubMed;
(o) F. Hernández-Culebras, M. Melle-Franco and A. Mateo-Alonso, Angew. Chem., Int. Ed., 2022, e202205018 Search PubMed.
-
(a) S. Ito, P. T. Herwig, T. Böhme, J. P. Rabe, W. Rettig and K. Müllen, J. Am. Chem. Soc., 2000, 122, 7698–7706 CrossRef CAS;
(b) L. Zhi, J. Wu and K. Müllen, Org. Lett., 2005, 7, 5761–5764 CrossRef CAS PubMed;
(c) H.-S. Kim, J.-H. Lee, T.-H. Kim, S. Okabe, M. Shibayama and S.-M. Choi, J. Phys. Chem. B, 2011, 115, 7314–7320 CrossRef CAS PubMed;
(d) X. Dou, W. Pisula, J. Wu, G. J. Bodwell and K. Müllen, Chem.–Eur. J., 2008, 14, 240–249 CrossRef CAS PubMed;
(e) J. P. Hill, W. Jin, A. Kosaka, T. Fukushima, H. Ichihara, T. Shimomura, K. Ito, T. Hashizume, N. Ishii and T. Aida, Science, 2004, 304, 1481–1483 CrossRef CAS;
(f) W. Jin, T. Fukushima, M. Niki, A. Kosaka, N. Ishii and T. Aida, Proc. Natl. Acad. Sci. U.S.A., 2005, 102, 10801–10806 CrossRef CAS PubMed;
(g) W. Jin, Y. Yamamoto, T. Fukushima, N. Ishii, J. Kim, K. Kato, M. Takata and T. Aida, J. Am. Chem. Soc., 2008, 130, 9434–9440 CrossRef CAS PubMed;
(h) K. V. Rao, K. Jayaramulu, T. K. Maji and S. J. George, Angew. Chem., Int. Ed., 2010, 49, 4218–4222 CrossRef CAS PubMed;
(i) K. V. Rao, K. K. R. Datta, M. Eswaramoorthy and S. J. George, Angew. Chem., Int. Ed., 2011, 50, 1179–1184 CrossRef CAS PubMed;
(j) A. Jain, K. V. Rao, C. Kulkarni, A. George and S. J. George, Chem. Commun., 2012, 48, 1467–1469 RSC.
- K. Kato, K. Takaba, S. Maki-Yonekura, N. Mitoma, Y. Nakanishi, T. Nishihara, T. Hatakeyama, T. Kawada, Y. Hijikata, J. Pirillo, L. T. Scott, K. Yonekura, Y. Segawa and K. Itami, J. Am. Chem. Soc., 2021, 143, 5465–5469 CrossRef CAS PubMed.
- K. Y. Kim, M. Ok, J. Kim, S. H. Jung, M. L. Seo and J. H. Jung, Gels, 2020, 6, 16 CrossRef CAS PubMed.
- A. Saeki, Y. Koizumi, T. Aida and S. Seki, Acc. Chem. Res., 2012, 45, 1193–1202 CrossRef CAS PubMed.
- S. Reboredo, R. M. Girón, S. Filippone, T. Mikie, T. Sakurai, S. Seki and N. Martín, Chem.–Eur. J., 2016, 22, 13627–13631 CrossRef CAS PubMed.
|
This journal is © The Royal Society of Chemistry 2022 |