DOI:
10.1039/D2SC02365B
(Edge Article)
Chem. Sci., 2022,
13, 9305-9309
Three-dimensional microporous and mesoporous covalent organic frameworks based on cubic building units†
Received
27th April 2022
, Accepted 15th July 2022
First published on 15th July 2022
Abstract
Covalent organic frameworks (COFs) have attracted extensive interest due to their unique structures and various applications. However, structural diversities are still limited, which greatly restricts the development of COF materials. Herein, we report two unusual cubic (8-connected) building units and their derived 3D imine-linked COFs with bcu nets, JUC-588 and JUC-589. Owing to these unique building blocks with different sizes, the obtained COFs can be tuned to be microporous or mesoporous structures with high surface areas (2728 m2 g−1 for JUC-588 and 2482 m2 g−1 for JUC-589) and promising thermal and chemical stabilities. Furthermore, the high selectivity of CO2/N2 and CO2/CH4, excellent H2 uptakes, and efficient dye adsorption are observed. This research thus provides a general strategy for constructing stable 3D COF architectures with adjustable pores via improving the valency of rigid building blocks.
Introduction
Constructed from organic building blocks and dynamic covalent linkages, covalent organic frameworks (COFs)1–5 have been widely studied in gas adsorption,6–8 heterogeneous catalysis,9–15 energy storage,16–20 and many other fields.21–26 Highly designable structures, light element compositions, large surface areas and promising stabilities endow these materials with prominent performances and potential applications.27–29 Interestingly, nano-morphologies of COFs, such as thin films and nanospheres, have also been explored and showed promising properties.30–35 Different from the common two-dimensional (2D) species, three-dimensional (3D) COFs are still scarce due to the limited building blocks and the serious crystallization problem. To date, only about a dozen of 3D topologies are available in the COF library, while most of them are based on 3- or 4-connected knots.36 Recently, several high valency knots have been designed successfully.37 For example, Cooper et al. reported a 3D cage-based COF with a twofold interpenetrated acs net via 6-connected organic cages.38 Our group developed triptycene-based building blocks and synthesized derived frameworks based on stp, ceq, and hea topologies.39–41 Notably, Yaghi and co-workers presented the pioneering idea to increase the diversity of COFs through higher valency nodes, and a series of BP-COFs were reported in view of 8-connected units and polycubane structures.42 Nevertheless, these frameworks were only constructed on the basis of the rare [B4P4O12] cube, which is defective in stability and functionalization. Therefore, it is highly desired to develop a more general strategy for incorporating high valency in stable COFs, such as imine-linked COFs as one of the most popular members, which is necessary for the extension of structural diversity and potential applications of COFs. However, this avenue is still not quite explored.43,44
In this work, we report two 3D microporous and mesoporous imine-linked COFs with bcu nets, termed JUC-588 and JUC-589, based on two novel cubic building blocks with different sizes. Both COFs show highly crystalline structures, large surface areas (2728 m2 g−1 for JUC-588 and 2482 m2 g−1 for JUC-589), and excellent thermal and chemical stabilities. In addition, the high selectivity of CO2/N2 (20 for JUC-588 and 14 for JUC-589) and CO2/CH4 (12 for JUC-588 and 10 for JUC-589), promising uptake of H2 (245 cm3 g−1 or 2.19 wt% for JUC-588 and 211 cm3 g−1 or 1.89 wt% for JUC-589 at 77 K and 1 bar), and adsorption of dye molecules for both COFs are studied. Therefore, this study provides a facile strategy for constructing 3D stable COFs with variable pores by enhancing the nodes of building blocks.
Results and discussion
To obtain highly connected knots, we designed two unique building units with eight aldehyde terminal groups, 3,3′,5,5′-tetra(3′′,5′′-diformylphenyl)-2,2′,6,6′-tetramethoxy-4,4′-dimethyl biphenyl (TDFTD) and 2,3,5,6-tetrakis(([3,5-diformylphenyl]-5-yl)-9H-carbazol-9-yl)-1,4-benzenedicarbonitrile (TDFCB, Scheme 1a and b). Due to steric hindrance, both linkers can act as cubic (8-connected) building blocks, which has been proven by single crystals of their analogues.45,46 Furthermore, because of these building units with distinct sizes, the pores of the obtained frameworks can be regulated. Through the condensation of eight-functional monomers and a linear building unit, 1,4-phenylenediamine (PDA, Scheme 1c), two 3D imine-linked frameworks with microporous or mesoporous channels were synthesized (Scheme 1d and e), which tends to be a bcu net based on reticular chemistry (Scheme 1f).47
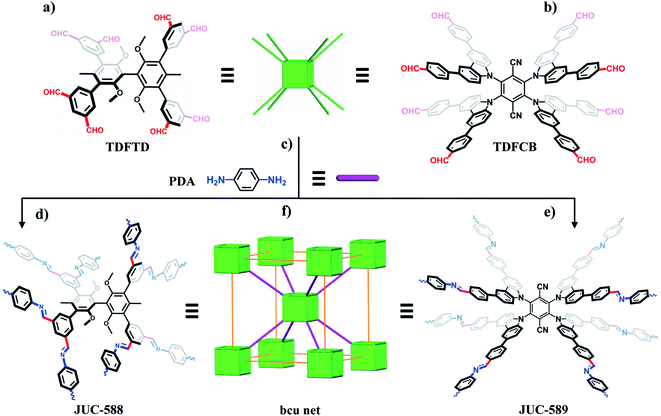 |
| Scheme 1 A strategy for preparing 3D COFs based on cubic building blocksa. aMolecular structures of TDFTD (a) and TDFCB (b) with cubic (8-connected) knots. Structure of a linear building block, PDA (c). Extended frameworks of JUC-588 (d) and JUC-589 (e). (f) A bcu net for JUC-588 and JUC-589. | |
Typically, the syntheses were carried out by suspending PDA and TDFTD or TDFCB in a mixed solution of dioxane and mesitylene in the presence of 3 M acetic acid aqueous solution, followed by heating at 120 °C for 3 days. Morphologies observed by scanning electron microscopy (SEM) and transmission electron microscopy (TEM) demonstrated aggregates of nanoparticles (Fig. S1–S4, ESI†). The formation of imine moieties was verified by Fourier transform infrared (FT-IR) spectra with new vibration peaks at 1630 cm−1 for JUC-588 and 1626 cm−1 for JUC-589 (Fig. S5, ESI†). Solid-state 13C cross-polarization magic-angle-spinning (CP/MAS) NMR spectroscopy was carried out and illustrated chemical shifts at 160 ppm for JUC-588 and 157 ppm for JUC-589, which further proved the formation of imine bonds (Fig. S6 and S7, ESI†). High thermal stability up to 400 °C for both COFs was confirmed by thermogravimetric analysis (TGA) under a nitrogen atmosphere (Fig. S8 and S9, ESI†). Meanwhile, promising chemical stabilities were evidenced by powder X-ray diffraction (PXRD) patterns after immersing in various solutions for 72 h, such as in acidic (1 M HCl), basic (1 M NaOH), and common organic solvents including dimethylformamide, dichloromethane, tetrahydrofuran, and n-hexane (Fig. S10 and S11, ESI†).
For the structure definition of JUC-588 and JUC-589, PXRDs were employed in conjunction with structural simulations (Fig. 1). Model structures were obtained through geometrical energy minimization using the Materials Studio software package on the basis of the bcu net.48 JUC-588 adopted the space group P42/nmc (No. 137) with unit cell parameters of a = b = 23.8190 Å, c = 23.9058 Å, and α = β = γ = 90°, while JUC-589 utilized Immm (No. 71) with a = 35.7099 Å, b = 9.7315 Å, c = 50.7873 Å, and α = β = γ = 90° (Tables S5 and S6, ESI†). The calculated PXRDs of the model crystal structures fitted well with the experimental patterns (Fig. S12 and S13, ESI†). Furthermore, we carried out full profile pattern matching (Pawley) refinement. Peaks at 5.25, 7.39, 8.27, 10.48, 11.7, 14.85, and 15.78 correspond to the (101), (002), (102), (202), (103), (004), and (303) facets of JUC-588, and 3.02, 6.06, 6.96, and 9.09° correspond to the (101), (202), (004), and (303) facets of JUC-589, respectively. The refinement results illustrated good agreement factors (Rp = 2.86% and Rwp = 3.76% for JUC-588; Rp = 1.12% and Rwp = 1.53% for JUC-589). Considering these results, both COFs were proposed to have the expected 3D frameworks with the bcu net, microporous JUC-588 with a pore size of 1.5 nm and rhombic mesoporous JUC-589 with a pore size of 2.1 nm × 2.4 nm, respectively (Fig. 2).
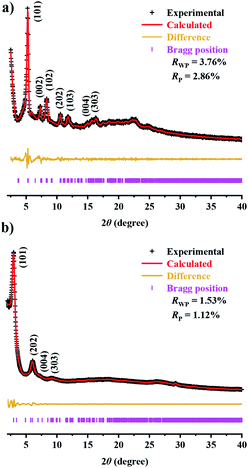 |
| Fig. 1 PXRD patterns of JUC-588 (a) and JUC-589 (b). | |
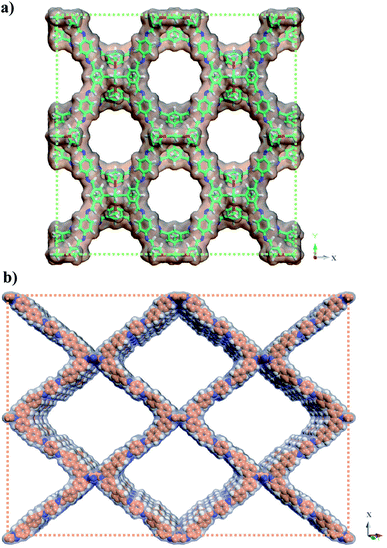 |
| Fig. 2 Structural representations of microporous JUC-588 (a) and mesoporous JUC-589 (b). | |
Subsequently, N2 adsorption and desorption measurements were carried out at 77 K to determine the permanent channel structures and specific surface areas. As shown in Fig. 3a, JUC-588 demonstrated a sharp increase at low pressure (P/P0 < 0.05), which illustrated the microporous structure with a type I isotherm. Different from JUC-588, the nitrogen adsorption of JUC-589 with a type IV isotherm exhibited an extra uptake step between P/P0 = 0.1 and 0.2, proving the mesoporous nature of this framework (Fig. 3b). Based on the Brunauer–Emmett–Teller (BET) model, specific surface areas were calculated to be 2728 m2 g−1 for JUC-588 and 2482 m2 g−1 for JUC-589 respectively, which is slightly lower than that (3300 m2 g−1) of JUC-564 with the largest pore size (43 Å) among 3D COFs and record-breaking low density (0.108 g cm−3) among crystalline materials reported to date (Fig. S14, ESI†).39 Pore-size distributions were determined by nonlocal density functional theory (NLDFT), in which JUC-588 showed a narrow distribution centered at 1.7 nm (Fig. 3c), while JUC-589 demonstrated the presence of dominant mesopores of about 2.1 nm and 2.3 nm (Fig. 3d). These results were in good agreement with those of the proposed models (1.5 nm for JUC-588 and 2.1 nm × 2.4 nm for JUC-589). Remarkably, JUC-589 represents the first example of mesoporous COFs based on an 8-connected node and shows a higher BET surface area and pore size compared to those of similar materials reported, such as BP-COF-1 (BET: 519 m2 g−1 and pore size: 0.6 nm),42 and NUST-5 (BET: 680 m2 g−1 and pore size: 1.5 nm),43 and NKCOF-23 (BET: 1900 m2 g−1 and pore size: 1.7 nm).44
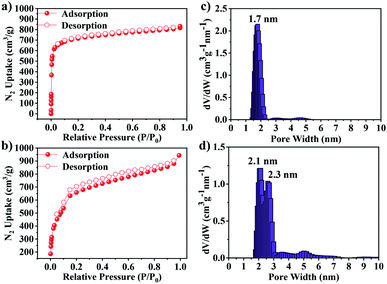 |
| Fig. 3 N2 adsorption–desorption isotherms at 77 K for JUC-588 (a) and JUC-589 (b). Pore size distribution for JUC-588 (c) and JUC-589 (d). | |
For exploring the potential applications of these novel COFs in the fields of energy or the environment, we tested the selectivity of CO2/N2 and CO2/CH4, storage of H2, and dye adsorption. The adsorption isotherms of CO2, N2 and CH4 were recorded at 273 K and 1 bar, which can be comparable with those from reported porous materials (Tables S2–S4†). As shown in Fig. 4a and b, the sorption amounts of CO2 were as high as 67 cm3 g−1 for JUC-588 and 40 cm3 g−1 for JUC-589 respectively, which are much higher than those of other gases, such as CH4 (16 cm3 g−1 for JUC-588 and 12 cm3 g−1 for JUC-589) and N2 (6 cm3 g−1 for JUC-588 and 5 cm3 g−1 for JUC-589). The ideal adsorption selectivity was calculated from the ratio of the initial slopes based on the Henry region of the isotherms (Fig. S15 and S16, ESI†), and high selectivity of CO2/N2 (20 for JUC-588 and 14 for JUC-589) or CO2/CH4 (12 for JUC-588 and 10 for JUC-589) was obtained (Table S1†). Furthermore, the H2 uptakes of both COFs were explored at different temperatures under 1 atm (Fig. 4c). The H2 storage capacity of JUC-588 was 245 cm3 g−1 (or 2.19 wt%) at 77 K and 138 cm3 g−1 (or 1.23 wt%) at 87 K respectively, which is lower than that of JUC-596 with functional triptycene groups with high fractional free volume and numerous available sites (305 cm3 g−1 or 2.72 wt% at 77 K);41 however, it exceeded that of JUC-589 (211 cm3 g−1 or 1.89 wt% at 77 K and 129 cm3 g−1 or 1.15 wt% at 87 K) and most porous materials under approximate test conditions, such as PAF-1 (179 cm3 g−1 or 1.60 wt%),49 MOF-177 (139 cm3 g−1 or 1.25 wt%),50 and CTC-COF (125 cm3 g−1 or 1.12 wt%).51 Based on the above results, the isosteric heats of adsorption (Qst) for H2 were calculated to be 4.12 kJ mol−1 for JUC-588 and 6.54 kJ mol−1 for JUC-589, respectively (Fig. S17 and S18, ESI†). In addition, mesoporous JUC-589 could effectively adsorb a dye molecule, Coomassie brilliant blue R250 (R250) with dimensions of about 2.1 nm × 1.8 nm × 0.6 nm, while JUC-588 could barely take up the R250 molecule due to its microporous channels, which further proves the existence of mesopores in JUC-589 (Fig. 4d, S19 and S20, ESI†). The maximum uptake capacity of R250 for JUC-589 is about 185.7 mg g−1, which is comparable with those from reported porous materials, such as PCN-124-stu(Cu) (78.7 mg g−1)52 and MIL-100(Fe, Al) (100.0 mg g−1).53
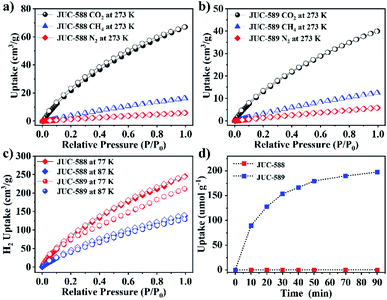 |
| Fig. 4 Adsorption of N2, CH4, and CO2 for JUC-588 (a) and JUC-589 (b) measured at 273 K. (c) Uptake of H2 for JUC-588 and JUC-589 measured at 77 K and 87 K. (d) Adsorption of a dye molecule for JUC-588 and JUC-589. | |
Conclusions
In summary, we designed two new monomers with eight aldehyde functional groups, TDFTD or TDFCB, and further assembled their derived 3D imine COFs with rare bcu nets, named microporous JUC-588 and mesoporous JUC-589. Both materials showed high crystallinity, large surface areas (up to 2728 m2 g−1 for JUC-588), and good thermal and chemical stabilities. Furthermore, the selectivity of CO2/N2 (20 for JUC-588 and 14 for JUC-589) and CO2/CH4 (12 for JUC-588 and 10 for JUC-589), the uptake of H2 (245 cm3 g−1 for JUC-588 and 211 cm3 g−1 for JUC-589 at 77 K and 1 bar), and the adsorption of dye were studied, which indicates the promising potential of these COFs constructed with high valency in the fields of energy and the environment. Therefore, this work develops a general strategy for enriching the structural diversity of COF materials and promotes their potential applications. Furthermore, the nano-morphologies of 3D COFs would be a great topic of research, which will greatly promote their performance in catalysis and separation, and related study is underway.
Data availability
The authors declare that the data supporting the findings of this study are available within the article and its ESI† or from the corresponding author upon reasonable request.
Author contributions
Q. F., X. Y. and H. L. were responsible for the overall design, direction and supervision of the project. L. L. performed the experimental work. X. G., H. Z., Z. Z. and Y. L. took SEM images and helped with the TGA and PXRD tests. L. Z. was in charge of other physical measurements. All authors discussed the results and contributed to the writing of the manuscript.
Conflicts of interest
There are no conflicts to declare.
Acknowledgements
This work was supported by the National Natural Science Foundation of China (22025504, 21621001, 21390394, and 22105082), “111” project (BP0719036 and B17020), China Postdoctoral Science Foundation (2020TQ0118 and 2020M681034), and program for JLU Science and Technology Innovative Research Team.
Notes and references
- A. P. Côté, A. I. Benin, N. W. Ockwig, M. O'Keeffe, A. J. Matzger and O. M. Yaghi, Science, 2005, 310, 1166 CrossRef PubMed.
- J. W. Colson, A. R. Woll, A. Mukherjee, M. P. Levendorf, E. L. Spitler, V. B. Shields, M. G. Spencer, J. Park and W. R. Dichtel, Science, 2011, 332, 228 CrossRef CAS PubMed.
- X. Feng, X. S. Ding and D. L. Jiang, Chem. Soc. Rev., 2012, 41, 6010–6022 RSC.
- Y. Yusran, X. Y. Guan, H. Li, Q. R. Fang and S. L. Qiu, Natl. Sci. Rev., 2020, 7, 170 CrossRef CAS PubMed.
- K. Y. Geng, T. He, R. Y. Liu, S. Dalapati, K. T. Tan, Z. P. Li, S. S. Tao, Y. F. Gong, Q. H. Jiang and D. L. Jiang, Chem. Rev., 2020, 120, 8814 CrossRef CAS PubMed.
- P. Kuhn, M. Antonietti and A. Thomas, Angew. Chem., Int. Ed., 2008, 47, 3450 CrossRef CAS PubMed.
- S. S. Han, H. Furukawa, O. M. Yaghi and W. A. Goddard, J. Am. Chem. Soc., 2008, 130, 11580 CrossRef CAS PubMed.
- Q. R. Fang, Z. B. Zhuang, S. Gu, R. B. Kaspar, J. Zheng, J. H. Wang, S. L. Qiu and Y. S. Yan, Nat. Commun., 2014, 5, 4503 CrossRef PubMed.
- S. Y. Ding, J. Gao, Q. Wang, Y. Zhang, W. G. Song, C. Y. Su and W. Wang, J. Am. Chem. Soc., 2011, 133, 19816 CrossRef CAS PubMed.
- V. S. Vyas, F. Haase, L. Stegbauer, G. Savasci, F. Podjaski, C. Ochsenfeld and B. V. Lotsch, Nat. Commun., 2015, 6, 8508 CrossRef CAS PubMed.
- Q. Sun, B. Aguila, J. Perman, N. Nguyen and S. Q. Ma, J. Am. Chem. Soc., 2016, 138, 15790 CrossRef CAS PubMed.
- X. R. Wang, X. Han, J. Zhang, X. W. Wu, Y. Liu and Y. Cui, J. Am. Chem. Soc., 2016, 138, 12332 CrossRef CAS PubMed.
- S. C. Yan, X. Y. Guan, H. Li, D. H. Li, M. Xue, Y. S. Yan, V. Valtchev, S. L. Qiu and Q. R. Fang, J. Am. Chem. Soc., 2019, 141, 2920 CrossRef CAS PubMed.
- S. Bi, P. Thiruvengadam, S. C. Wei, W. B. Zhang, F. Zhang, L. S. Gao, J. S. Xu, D. Q. Wu, J. S. Chen and F. Zhang, J. Am. Chem. Soc., 2020, 142, 11893 CrossRef CAS PubMed.
- F. Q. Chen, X. Y. Guan, H. Li, J. H. Ding, L. K. Zhu, B. Tang, V. Valtchev, Y. S. Yan, S. L. Qiu and Q. R. Fang, Angew. Chem., Int. Ed., 2021, 60, 22230 CrossRef CAS PubMed.
- S. Wan, J. Guo, J. Kim, H. Ihee and D. L. Jiang, Angew. Chem., Int. Ed., 2008, 47, 8826 CrossRef CAS PubMed.
- M. Dogru, M. Handloser, F. Auras, T. Kunz, D. Medina, A. Hartschuh, P. Knochel and T. Bein, Angew. Chem., Int. Ed., 2013, 52, 2920 CrossRef CAS PubMed.
- G. H. V. Bertrand, V. K. Michaelis, T. C. Ong, R. G. Griffin and M. Dinca, Proc. Natl. Acad. Sci. U. S. A., 2013, 110, 4923 CrossRef CAS PubMed.
- H. Li, J. H. Chang, S. S. Li, X. Y. Guan, D. H. Li, C. Y. Li, L. X. Tang, M. Xue, Y. S. Yan, V. Valtchev, S. L. Qiu and Q. R. Fang, J. Am. Chem. Soc., 2019, 141, 13324 CrossRef CAS PubMed.
- Y. Du, H. S. Yang, J. M. Whiteley, S. Wan, Y. H. Jin, S. H. Lee and W. Zhang, Angew. Chem., Int. Ed., 2016, 55, 1737 CrossRef CAS PubMed.
- S. Chandra, T. Kundu, S. Kandambeth, R. BabaRao, Y. Marathe, S. M. Kunjir and R. Banerjee, J. Am. Chem. Soc., 2014, 136, 6570 CrossRef CAS PubMed.
- S. Wang, Q. Y. Wang, P. P. Shao, Y. Z. Han, X. Gao, L. Ma, S. Yuan, X. J. Ma, J. W. Zhou, X. Feng and B. Wang, J. Am. Chem. Soc., 2017, 139, 4258 CrossRef CAS PubMed.
- X. Y. Guan, H. Li, Y. C. Ma, M. Xue, Q. R. Fang, Y. S. Yan, V. Valtchev and S. L. Qiu, Nat. Chem., 2019, 11, 587 CrossRef CAS PubMed.
- J. P. Yu, L. Y. Yuan, S. Wang, J. H. Lan, L. R. Zheng, C. Xu, J. Chen, L. Wang, Z. W. Huang, W. Q. Tao, Z. R. Liu, Z. F. Chai, J. K. Gibson and W. Q. Shi, CCS Chem., 2019, 1, 286 CAS.
- R. R. Liang, F. Z. Cui, R. H. A, Q. Y. Qi and X. Zhao, CCS Chem., 2020, 2, 139 CrossRef CAS.
- J. H. Chang, H. Li, J. Zhao, X. Y. Guan, C. M. Li, G. T. Yu, V. Valtchev, Y. S. Yan, S. L. Qiu and Q. R. Fang, Chem. Sci., 2021, 12, 8452 RSC.
- S. Y. Ding and W. Wang, Chem. Soc. Rev., 2013, 42, 548 RSC.
- D. Rodriguez-San-Miguel and F. Zamora, Chem. Soc. Rev., 2019, 48, 4375 RSC.
- S. Kandambeth, K. Dey and R. Banerjee, J. Am. Chem. Soc., 2019, 141, 1807 CrossRef CAS PubMed.
- S. Mohata, K. Dey, S. Bhunia, N. Thomas, E. B. Gowd, T. G. Ajithkumar, C. M. Reddy and R. Banerjee, J. Am. Chem. Soc., 2022, 144, 400 CrossRef CAS PubMed.
- A. K. Mahato, S. Bag, H. S. Sasmal, K. Dey, I. Giri, M. Linares-Morear, C. Carbonell, P. Falcaro, E. B. Gowd, R. K. Vijayaraghavan and R. Banerjee, J. Am. Chem. Soc., 2021, 143, 20916 CrossRef PubMed.
- K. Dey, S. Mohata and R. Banerjee, ACS Nano, 2021, 15, 12723 CrossRef CAS.
- H. S. Sasmal, S. Bag, B. Chandra, P. Majumder, H. Kuiry, S. Karak, S. S. Gupta and R. Banerjee, J. Am. Chem. Soc., 2021, 143, 8426 CrossRef CAS PubMed.
- K. Dey, S. Bhunia, H. S. Sasmal, C. M. Reddy and R. Banerjee, J. Am. Chem. Soc., 2021, 143, 955 CrossRef CAS PubMed.
- Z. S. Xiong, B. B. Sun, H. B. Zou, R. W. Wang, Q. R. Fang, Z. T. Zhang and S. L. Qiu, J. Am. Chem. Soc., 2022, 144, 6583 CrossRef CAS PubMed.
- X. Y. Guan, F. Q. Chen, Q. R. Fang and S. L. Qiu, Chem. Soc. Rev., 2020, 49, 1357 RSC.
- O. Yahiaoui, A. N. Fitch, F. Hoffman, M. Froba, A. Thomas and J. Roeser, J. Am. Chem. Soc., 2018, 140, 5330 CrossRef CAS PubMed.
- Q. Zhu, X. Wang, R. Clowes, P. Cui, L. J. Chen, M. A. Little and A. I. Cooper, J. Am. Chem. Soc., 2020, 142, 16842 CrossRef CAS PubMed.
- H. Li, J. H. Ding, X. Y. Guan, F. Q. Chen, C. Y. Li, L. K. Zhu, M. Xue, D. Q. Yuan, V. Valtchev, Y. S. Yan, S. L. Qiu and Q. R. Fang, J. Am. Chem. Soc., 2020, 142, 13334 CrossRef CAS PubMed.
- H. Li, F. Q. Chen, X. Y. Guan, J. L. Li, C. Y. Li, B. Tang, V. Valtchev, Y. S. Yan, S. L. Qiu and Q. R. Fang, J. Am. Chem. Soc., 2021, 143, 2654 CrossRef CAS PubMed.
- C. Y. Yu, H. Li, Y. J. Wang, J. Q. Suo, X. Y. Guan, R. Wang, V. Valtchev, Y. S. Yan, S. L. Qiu and Q. R. Fang, Angew. Chem., Int. Ed., 2022, 61, e202117101 CAS.
- C. Gropp, T. Q. Ma, N. Hanikel and O. M. Yaghi, Science, 2020, 370, 424 CrossRef PubMed.
- Z. Shan, M. M. Wu, D. Y. Zhu, X. W. Wu, K. Zhang, R. Verduzco and G. Zhang, J. Am. Chem. Soc., 2022, 144, 5728 CrossRef CAS PubMed.
- F. Z. Jin, E. Lin, T. H. Wang, S. B. Geng, T. Wang, W. S. Liu, F. H. Xiong, Z. F. Wang, Y. Chen, P. Cheng and Z. J. Zhang, J. Am. Chem. Soc., 2022, 144, 5643 CrossRef CAS PubMed.
- W. G. Lu, Z. W. Wei, D. Q. Yuan, J. Tian, S. Fordham and H. C. Zhou, Chem. Mater., 2014, 26, 4589 CrossRef CAS.
- M. K. Etherington, N. A. Kukhta, H. F. Higginbotham, A. Danos, A. N. Bismillah, D. R. Graves, P. R. McGonigal, N. Haase, A. Morherr, A. S. Batsanov, C. Pflumm, V. Bhalla, M. R. Bryce and A. P. Monkman, J. Phys. Chem. C, 2019, 123, 11109 CrossRef CAS PubMed.
- M. Li, D. Li, M. O'Keeffe and O. M. Yaghi, Chem. Rev., 2014, 114, 1343 CrossRef CAS PubMed.
-
Materials Studio ver. 7.0, Accelrys Inc., San Diego, CA Search PubMed.
- T. Ben, H. Ren, S. Q. Ma, D. P. Cao, J. H. Lan, X. F. Jing, W. C. Wang, J. Xu, F. Deng, J. M. Simmons, S. L. Qiu and G. S. Zhu, Angew. Chem., Int. Ed., 2009, 48, 9457 CrossRef CAS PubMed.
- H. K. Chae, D. Y. Siberio-Perez, J. Kim, Y. Go, M. Eddaoudi, A. J. Matzger, M. O'Keeffe and O. M. Yaghi, Nature, 2004, 427, 523 CrossRef CAS PubMed.
- J. R. Zhen, S. Y. Ding, W. Wang, J. M. Liu, J. L. Sun, Z. T. Huang and Q. Y. Zheng, Chin. J. Chem., 2016, 34, 783 CrossRef CAS.
- W. G. Jin, W. Chen, P. H. Xu, X. W. Lin, X. C. Huang, G. H. Chen, F. S. Lu and X. M. Chen, Chem.–Eur. J., 2017, 23, 13058 CrossRef CAS PubMed.
- H. Lv, H. Zhao, T. Cao, L. Qian, Y. Wang and G. Zhao, J. Mol. Catal. A: Chem., 2015, 400, 81 CrossRef CAS.
Footnote |
† Electronic supplementary information (ESI) available: Materials and characterization, SEM images, TEM images, FT-IR spectra, solid-state 13C NMR spectra, TGA curves, PXRD patterns, gas adsorption, dye adsorption, and unit cell parameters. See https://doi.org/10.1039/d2sc02365b |
|
This journal is © The Royal Society of Chemistry 2022 |
Click here to see how this site uses Cookies. View our privacy policy here.