DOI:
10.1039/D2SC02277J
(Edge Article)
Chem. Sci., 2022,
13, 7256-7263
Three-component carboacylation of alkenes via cooperative nickelaphotoredox catalysis†
Received
22nd April 2022
, Accepted 27th May 2022
First published on 30th May 2022
Abstract
Various commercially available acyl chlorides, aldehydes, and alkanes were exploited for versatile three-component 1,2-carboacylations of alkenes to forge two vicinal C–C bonds through the cooperative action of nickel and sodium decatungstate catalysis. A wealth of ketones with high levels of structural complexity was rapidly obtained via direct functionalization of C(sp2)/C(sp3)–H bonds in a modular manner. Furthermore, a regioselective late-stage modification of natural products showcased the practical utility of the strategy, generally featuring high resource economy and ample substrate scope.
Introduction
Alkenes have found widespread applications for the rapid construction of diverse bioactive molecules and functionalized polymers.1 In this context, alkene difunctionalization has undergone impressive progress during the last decade.2,3 Recently, cooperative nickelaphotoredox catalysis has attracted increasing attention by installing two functional groups onto C
C double bonds to achieve the 1,2-difunctionalization of alkenes under mild reaction conditions, which was elegantly explored by Nevado,4 Molander,5 Aggarwal,6 Rueping,7 Martin,8 Studer,9 Koh10 among others.11,12 For instance, the groups of Kong13 and Molander5a disclosed two elegant approaches for the carboarylation of alkenes through the activation of aliphatic C–H bonds via cooperative nickelaphotoredox catalysis. Despite the indisputable advances, direct 1,2-carboacylations of alkenes for the preparation of ketones via nickelaphotoredox catalysis is underdeveloped.
Alkene 1,2-difunctionalizations by means of the direct 1,2-carboacylation can access carbonyl compounds, which are prestigious structural motifs and represent important pharmacophores existing in many drug molecules such as ibuprofen, ketoprofen, and naproxen.14 Most of the traditional methods are based on preformed organometallic reagents, which are typically not compatible with electrophilic sensitive functional groups (Scheme 1A).15 Moreover, the multistep synthesis significantly decreases the efficiency and resource economy of these syntheses.16 Recently, efficient strategies have surfaced to achieve direct 1,2-carboacylations of alkenes,17,18 and especially, N-heterocyclic carbene (NHC)-catalyzed radical-based 1,2-carboacylations of alkenes have witnessed major momentum (Scheme 1B).19,20 However, key synthesis challenges remain: (a) pre-activated alkyl and acyl substrates are required, which translate into the low atomic economy; (b) limited radical precursors including alkyl halides, redox-active esters and cyclobutanone oxime esters served as alkyl resources; (c) preformed complex acyl reagents are required. To overcome these major limitations, it is hence highly desirable to develop new, alternative strategies that provide efficient access to substituted carbonyl compounds. In this context, acyl chlorides and aldehydes are inexpensive, abundant and easily available commodity chemicals and can serve as suitable acyl sources for the direct transformation of alkenes.21,22 Their transformation in one single-step operation is appealing but challenging. In sharp contrast, we, herein, present a photochemical nickel-catalyzed approach for the preparation of functionalized carbonyl compounds, using acyl chlorides as acyl reagent to incorporate simultaneously and with excellent selectivity two different carbon fragments onto alkenes (Scheme 1C). This method exhibits broad substrate scope, it is compatible with carbonyl radicals from various aldehydes and with diverse tertiary/secondary/primary alkyl radicals, indeed contrasting with previous reports.
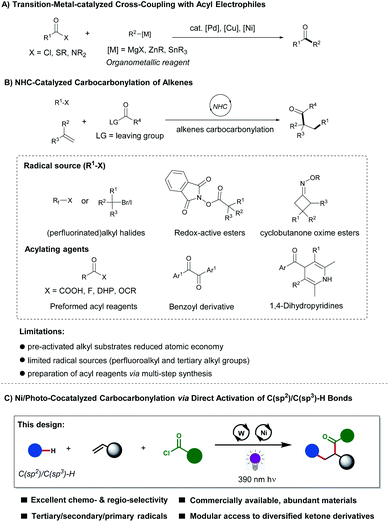 |
| Scheme 1 Synthetic approaches to carbonyl compounds. | |
Results and discussion
To identify the ideal reaction conditions for the envisioned carboacylation of alkenes reaction, we chose three commercial reagents, namely valeraldehyde (1), ethyl acrylate (2) and 3-methyl-benzoyl chloride (3), as the substrates (Table 1). After a careful investigation of all reaction parameters (more details see the ESI†), we arrived at the optimized conditions, involving 2 mol% sodium decatungstate (NaDT) and 10 mol% Ni(dtbbpy)Br2, along with K2HPO4 as the base under blue LED (10 W) irradiation in acetone (0.2 M) at 30 °C for 24 h, furnishing the dicarbonylation product 4 in 71% isolated yield (Table 1, entry 1). Comparable photocatalyst tetrabutylammonium decatungstate (TBADT) resulted in a lower yield (entry 2). This result shows that ion pairing could be an important factor to modulate the reactivity in ionic photoredox catalysts.23 Differently substituted 2,2′-bipyridines, such as ligands L1, L2 or L4, led to dramatic erosions in yield (entry 3). In addition, employing phenanthroline (L5) or bisoxazole (L6) as ligand gave no conversion, showing that the backbone of the ligand had a great impact on the reactivity for the catalyst (entry 4). Replacing K2HPO4 with other bases, like K3PO4 or Na2HPO4, was found to be less efficient to the product yield (entries 5 and 6). Only small traces or even no products could be observed when the reaction was carried out using other solvents (entries 7–9). Reducing the amount of aldehyde from 5 equiv. to 4 equiv. had a little impact on the reaction outcome (entry 10). In addition, reducing alkene to 1 equiv. led to dramatic erosions in yield (entry 11). Control experiments confirmed that the alkene carbocarbonylations did not occur without the nickel catalyst, the photocatalyst or light (entry 12).
Table 1 Optimization of reaction conditionsa
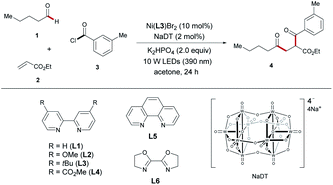
|
Entry |
Variation from “standard conditions” |
Yieldb/[%] |
Reaction conditions: 1 (1 mmol), 2 (0.4 mmol), 3 (0.2 mmol), Ni(L3)Br2 (10 mol%), NaDT (2 mol%), K2HPO4 (0.4 mmol) in acetone (0.2 M) at 30 °C under irradiation of LEDs (10 W, λ = 390 nm) for 24 hours.
GC yield with n-dodecane as internal standard.
Isolated yield.
|
1 |
None |
76 (71)c |
2 |
TBADT instead of NaDT |
59 |
3 |
L1, L2 or L4 instead of L3 |
26, 36, 15 |
4 |
L5 or L6 instead of L3 |
0 |
5 |
K3PO4 instead of K2HPO4 |
42 |
6 |
Na2HPO4 instead of K2HPO4 |
27 |
7 |
MeCN instead of acetone |
11 |
8 |
DCM instead of acetone |
Trace |
9 |
DMSO instead of acetone |
0 |
10 |
4 equivalents of valeraldehyde were used |
70 |
11 |
1 equivalent of ethyl acrylate were used |
59 |
12 |
Absence of NaDT, Ni or light |
0 |
After having established the optimized reaction conditions, we next assessed the generality of acyl chlorides 3 in this cooperative catalysis (Table 2). A wide range of acyl chlorides 3 reacted successfully, affording the 1,4-dicarbonyl compounds (4–23) in moderate yields. The cooperative catalysis was shown to be amenable for both electron-donating substituents such as methyl, methoxy and thiomethyl, as well as electron-withdrawing substituents, including trifluoromethyl, ester and cyano groups (4–15). Acyl chlorides with halogen groups in the meta or para position, including F, Cl, Br and chloromethyl, as well as various heteroaroyl chlorides, were perfectly compatible, providing an opportunity for further late-stage transformations. Notably, dialkyl carbamoyl chloride like dimethylcarbamoyl chloride were tolerated as well (24). Under otherwise identical reaction conditions, aliphatic acyl chlorides failed thus far to deliver the three-component products.
Table 2 Scope of three-component dicarbonylation of alkenes a

|
Reaction conditions: 1 (1 mmol), 2 (0.4 mmol), 3 (0.2 mmol), Ni(dtbbpy)Br2 (10 mol%), NaDT (2 mol%), K2HPO4 (0.4 mmol) in acetone (0.2 M) at 30 °C under irradiation of LEDs (10 W, λ = 390 nm) for 24 hours. Isolated yields. d.r., diastereomeric ratio.
6.0 equivalents of aldehyde 1 and 4.0 equivalents of alkene 2 were used.
|
|
Thereafter, we turned our attention to the reactivity of aldehydes 1 (Table 2). A variety of aliphatic aldehydes with different groups were hence probed, showing good reactivity to afford the desired products 25–30. Interestingly, (hetero)aromatic aldehydes were also successfully transformed into the corresponding products 31–34. Subsequently, we explored the generality of this dual metallaphotoredox for different alkene 2 types as reaction partners with aldehydes and acyl chlorides. A broad range of acrylates with different groups was examined. The different electronic and steric configurations of the ester group of alkenes also have shown good tolerance in this photocatalysis (35–39). Furthermore, it was demonstrated that our strategy was not only applicable to acrylates. Indeed, it also enabled difunctionalization of other electron-deficient olefins, including methyl vinyl ketone (40), acrylonitrile (41), N-methylmaleimide (42) and phenyl vinyl sulfone (43). In order to further demonstrate the potential of the dual catalysis, the complex acrylate derived from the natural hormone estrone was subjected to the protocol (44). The reaction proceeded efficiently, affording the desired 1,4-diketone in 58% yield with excellent chemo- and regioselectivity, indicating its potential for late-stage functionalization of complex molecules. Under slightly modified conditions, this strategy was also applicable for dicarbonylation of styrenes bearing electron-withdrawing groups such as 4-(trifluoromethyl)styrene, giving 45 in 28% yield. But styrenes substrates containing electron-donating groups such as 4-methylstyrene and unactivated alkene like hex-1-ene were not tolerated.
Having established the 1,2-dicarbonylation, the suitability of this strategy to introduce simultaneously an alkyl group and a carbonyl group onto a double bond was studied, by employing hydrocarbons and acyl chlorides as the radical precursor and the acyl source, respectively (Table 3). Gratifyingly, when three-component carbonylation of alkenes was conducted with 1,3-benzodioxole, ethyl acrylate and 4-methylbenzoyl chloride under slightly modified conditions, the desired product 46 was obtained in 69% yield. Afterward, oxygen-containing substrates were incorporated onto C–C double bond of alkenes, in moderate to good yields and with excellent regioselectivity under the optimized conditions (47–49). It is particularly noteworthy that our photochemical method was not limited to tertiary (3°) alkyl radicals. Indeed, the less stable secondary (2°) and primary (1°) alkyl radicals were likewise compatible with this dual catalysis. Based on these results, we wondered whether our protocol can be extended to unactivated C–H precursors other than ether motifs. Importantly, a wide range of alkanes with various groups were successfully employed as radical precursors, thus resulting in the corresponding ketone compounds in satisfactory yields with excellent regioselectivities (50–54). For instance, 2,3-dimethylbutane was selectively functionalized on the tertiary C–H bond (52). Cyclopentanone was exclusively functionalized at the β position in 57% yield (53). In addition, the reaction conducted with cyclopentanecarbonitrile with strong coordination ability formed the desired product 54.
Table 3 Scope of three-component carboacylation of alkenesa

|
General reaction conditions: 1 (2 mmol), 2 (0.4 mmol), 3 (0.2 mmol), Ni(dtbbpy)Br2 (10 mol%), NaDT (2 mol%), K2HPO4 (0.4 mmol) in acetone (0.2 M) at 30 °C under irradiation of LEDs (10 W, λ = 390 nm) for 24 hours. Yields of isolated products. d.r., diastereomeric ratio; r.r., regioisomeric ratio.
|
|
Powerful transformations of carbonyl compounds were also conducted, which demonstrates the synthetic utility of dual photocatalysis. For example, 1,4-dicarbonyl compounds were preferentially transformed by cyclizations to various heteroarenes such as furan, pyrrole and thiophene derivatives 55–57, in excellent yields of 82–92% (Scheme 2). Pyrrole 57 has a similar skeleton with atorvastatin, which is a drug widely applied to treat hyperlipidemia.
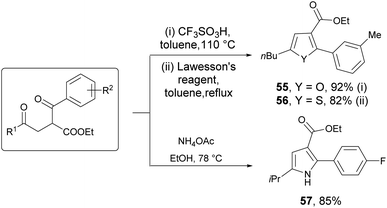 |
| Scheme 2 Synthetic applications. | |
In order to highlight the translational impact of this cooperative catalysis for complex organic molecules, we next exploited natural product scaffolds. We performed late-stage functionalization (Scheme 3). Ambroxide, which is an important chemical in the fragrance industries due to its pleasant smell and fixative properties, was efficiently functionalized in the α-oxy position in 51% yields (58). In addition, natural product sclareolide, which contains six different methylene sites and two tertiary C–H bonds, was preferentially functionalized on the C ring, generating the corresponding C(sp3)–H functionalized product (59). Likewise, medically relevant coupling partners such as camphene, was also successfully transformed to the desired product (60). From the point of view of atomic economy, the use of a large excess of complex substrates will limit the application of this strategy in natural products. Under slightly modified conditions, we also successfully reduce the amounts of complex substrates from 5 equiv. to 1 equiv. to achieve late-stage functionalization in moderate yields through increasing the equivalents of alkenes and acyl chlorides.
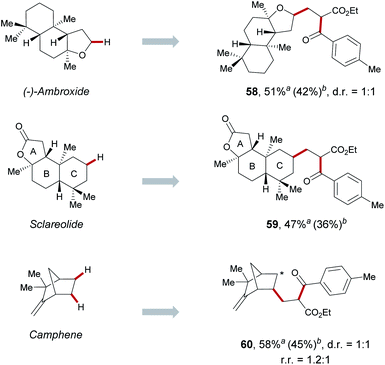 |
| Scheme 3 Late-stage functionalization of complex molecules. a Reaction conditions: 1 (1 mmol), 2 (0.4 mmol), 3 (0.2 mmol), Ni(dtbbpy)Br2 (10 mol%), NaDT (2 mol%), K2HPO4 (0.4 mmol) in acetone (0.2 M) at 30 °C under irradiation of LEDs (10 W, λ = 390 nm) for 24 hours. b1 (0.2 mmol), 2 (0.6 mmol), 3 (0.6 mmol), Ni(dtbbpy)Br2 (10 mol%), NaDT (2 mol%), K2HPO4 (0.4 mmol) in acetone (0.2 M) at 30 °C under irradiation of LEDs (10 W, λ = 390 nm) for 24 hours. | |
To gain insight into the catalysis mode of action, several mechanistic experiments were performed (Scheme 4). The addition of TEMPO (1.0 equiv.) strongly inhibited the three-component coupling reaction, suggesting that this transformation involved a radical mechanism. Thus, a small amount of the TEMPO-adduct 2,2,6,6-tetramethylpiperidin-1-yl pentanoate could be observed by GC-MS (Scheme 4a). Then, a reaction of Ni(COD)2 with benzoyl chloride in THF at room temperature afforded nickel(II) complex 61. We have not observed the corresponding 1,4-diketone 7 when a stoichiometric reaction of nickel(II) complex 61 was performed with valeraldehyde (1) and ethyl acrylate (2) (Scheme 4b). This finding demonstrates that oxidative addition takes place after radical capture of the nickel complex. Furthermore, when the reaction was carried out with 10 mol% of nickel-complex 61 to replace Ni(dtbbpy)Br2 as the catalyst under conditions of carboacylation, the expected product 7 was observed in 65% yield (Scheme 4c).
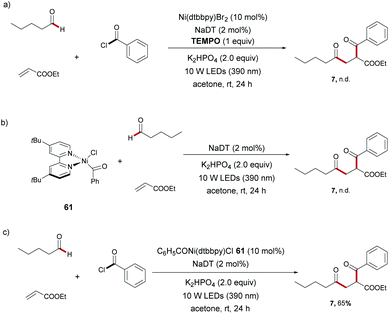 |
| Scheme 4 Mechanistic experiments. | |
Based on our experimental results and previous findings,5a,10 we propose a plausible mechanism for the photoredox/nickel-catalyzed carbocarbonylation, as outlined in Scheme 5. Upon visible light irradiation, NaDT is excited to generate photoexcited decatungstate A, which is capable of abstracting a hydrogen atom from a non-activated C–H bond of aldehydes/hydrocarbon resulting in carbon-centered radical intermediate D and [W]5−H+ (B). The latter can be reduced to afford [W]6−2H+ (C) in the presence of [W]4−, while C is able to reduce Ni(I) catalyst to the corresponding Ni(0) species via successive single-electron transfer. The addition of D to alkenes gives an alkyl radical E, which can be captured by Ni(0) to form alkyl-Ni(I) intermediate F. Then oxidative addition of acyl chloride to alkyl-Ni(I) intermediate generates a nickel(III) species G, which performs reductive elimination to give the corresponding carbonyl compounds and nickel(I) species H.
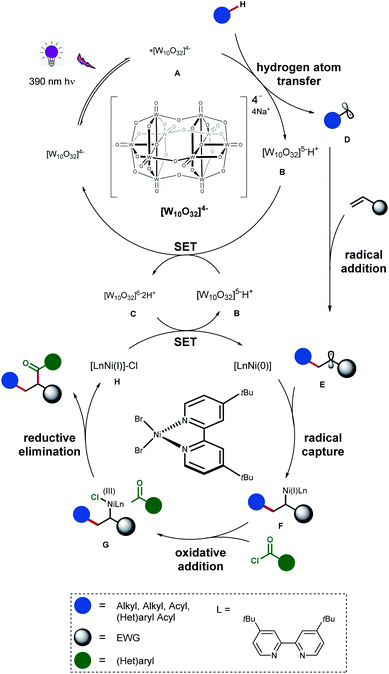 |
| Scheme 5 Proposed mechanism. | |
Conclusions
In conclusion, we have successfully achieved three-component intermolecular carbocarbonylation of alkenes by the synergy of nickel-photoredox catalysts. This robust strategy provided facile access to various complex ketones with excellent regioselectivities starting from a diverse class of inexpensive commercial chemicals. With the use of aldehydes and unactivated hydrocarbons, this methodology showed a broad scope and high functional group tolerance. Our strategy is expected to find broad applications in medical chemists as well as pharmaceutical and agricultural industries because it enables the facile construction of carbonyl compounds with high levels of complexity.
Data availability
All experimental data, procedures for data analysis and pertinent data sets are provided in the ESI.†
Author contributions
D. W. and L. A. conceived the project. D. W. conducted the experiments. D. W. and L. A. wrote the manuscript. All authors discussed the results.
Conflicts of interest
There are no conflicts to declare.
Acknowledgements
Generous support by the DFG (Gottfried-Wilhelm-Leibniz award to L. A.) and the Alexander von Humboldt Foundation (fellowship to D. W.) is gratefully acknowledged.
Notes and references
-
(a) J. Derosa, O. Apolinar, T. Kang, V. T. Tran and K. M. Engle, Chem. Sci., 2020, 11, 4287–4296 RSC;
(b) Z. Zhang, T. Roisnel, P. H. Dixneuf and J.-F. Soulé, Angew. Chem., Int. Ed., 2020, 58, 14110–14114 (
Angew. Chem.
, 2019
, 131
, 14248–14252
) CrossRef PubMed;
(c) R. K. Dhungana, S. Kc, P. Basnet and R. Giri, Chem. Rec., 2018, 18, 1314–1340 CrossRef CAS PubMed;
(d) J. S. Zhang, L. Liu, T. Chen and L. B. Han, Chem.–Asian J., 2018, 13, 2277–2291 CrossRef CAS PubMed.
-
(a) H. Tu, F. Wang, L. Huo, Y. Li, S. Zhu, X. Zhao, H. Li, F. Qing and L. Chu, J. Am. Chem. Soc., 2020, 142, 9604–9611 CrossRef CAS PubMed;
(b) L. Song, D.-M. Fu, L. Chen, Y.-X. Jiang, J.-H. Ye, L. Zhu, Y. Lan, Q. Fu and D.-G. Yu, Angew. Chem., Int. Ed., 2020, 59, 21121–21128 (
Angew. Chem.
, 2020
, 132
, 14248–14252
) CrossRef CAS PubMed;
(c) X. Wu and C. Zhu, Acc. Chem. Res., 2020, 53, 1620–1636 CrossRef CAS;
(d) Z. Li, G. Fang, Q. Gu and X. Liu, Chem. Soc. Rev., 2020, 49, 32–48 RSC;
(e) P. Fan, Y. Lan, C. Zhang and C. Wang, J. Am. Chem. Soc., 2020, 142, 2180–2186 CrossRef CAS;
(f) H. Jiang and A. Studer, Chem. Soc. Rev., 2020, 49, 1790–1811 RSC;
(g) Z. Li, M. Wang and Z. Shi, Angew. Chem., Int. Ed., 2020, 60, 186–190 (
Angew. Chem.
, 2020
, 133
, 188–192
) CrossRef;
(h) X. Wang, X. Lu, S. He and Y. Fu, Chem. Sci., 2020, 11, 7950–7956 RSC;
(i) D. Anthony, Q. Lin, J. Baudet and T. Diao, Angew. Chem., Int. Ed., 2019, 58, 3198–3202 (
Angew. Chem.
, 2019
, 131
, 3230–3234
) CrossRef CAS PubMed;
(j) D. Wang, B. Dong, Y. Wang, J. Qian, J. Zhu, Y. Zhao and Z. Shi, Nat. Commun., 2019, 10, 3539–3548 CrossRef PubMed;
(k) Q. Fu, Z.-Y. Bo, J.-H. Ye, T. Ju, H. Huang, L.-L. Liao and D.-G. Yu, Nat. Commun., 2019, 10, 3592–3600 CrossRef PubMed;
(l) M. Zhang, J. Xie and C. Zhu, Nat. Commun., 2018, 9, 3517–3526 CrossRef PubMed;
(m) F. Wang, P. Chen and G. Liu, Acc. Chem. Res., 2018, 51, 2036–2046 CrossRef CAS PubMed;
(n) M. Yan, J. C. Lo, J. T. Edwards and P. S. Baran, J. Am. Chem. Soc., 2016, 138, 12692–12714 CrossRef CAS PubMed.
-
(a) T. Xiong and Q. Zhang, Chem. Soc. Rev., 2021, 50, 8857–8873 RSC;
(b) S. Sharma, J. Singh and A. Sharmaa, Adv. Synth. Catal., 2021, 363, 3146–3169 CrossRef CAS;
(c) L. Pitzer, J. L. Schwarz and F. Glorius, Chem. Sci., 2019, 10, 8285–8291 RSC;
(d) Y. Jin and C. Wang, Chem. Sci., 2019, 10, 1780–1785 RSC;
(e) Z. Zhang, L. Gong, X.-Y. Zhou, S.-S. Yan, J. Li and D.-G. Yu, Acta Chim. Sin., 2019, 77, 783–793 CrossRef CAS;
(f) T. Koike and M. Akita, Chem, 2018, 4, 409–437 CrossRef CAS;
(g) G. S. Sauer and S. Lin, ACS Catal., 2018, 8, 5175–5187 CrossRef CAS;
(h) K. Wang, Z. Ding, Z. Zhou and W. Kong, J. Am. Chem. Soc., 2018, 140, 12364–12368 CrossRef CAS PubMed.
-
(a) X. Wei, W. Shu, A. García-Domínguez, E. Merino and C. Nevado, J. Am. Chem. Soc., 2020, 142, 13515–13522 CrossRef CAS PubMed;
(b) W. Shu, A. García-Domínguez, M. T. Quirls, R. Mondal, D. J. Cordenas and C. Nevado, J. Am. Chem. Soc., 2019, 141, 13812–13821 CrossRef CAS PubMed;
(c) A. García-Domínguez, R. Mondal and C. Nevado, Angew. Chem., Int. Ed., 2019, 58, 12286–12290 (
Angew. Chem.
, 2019
, 131
, 12414–12418
) CrossRef;
(d) A. García-Domínguez, Z. Li and C. Nevado, J. Am. Chem. Soc., 2017, 139, 6835–6838 CrossRef PubMed;
(e) E. Merino and C. Nevado, Chem. Soc. Rev., 2014, 43, 6598–6608 RSC.
-
(a) M. W. Campbell, M. Yuan, V. C. Polites, O. Gutierrez and G. A. Molander, J. Am. Chem. Soc., 2021, 143, 3901–3910 CrossRef CAS PubMed;
(b) A. Lipp, S. O. Badir and G. A. Molander, Angew. Chem., Int. Ed., 2021, 60, 1714–1726 (
Angew. Chem.
, 2021
, 133
, 1738–1750
) CrossRef CAS PubMed;
(c) S. O. Badir and G. A. Molander, Chem, 2020, 6, 1327–1339 CrossRef CAS PubMed;
(d) M. W. Campbell, J. S. Compton, C. B. Kelly and G. A. Molander, J. Am. Chem. Soc., 2019, 141, 20069–20078 CrossRef CAS PubMed.
- R. S. Mega, V. K. Duong, A. Noble and V. K. Aggarwal, Angew. Chem., Int. Ed., 2020, 59, 4375–4379 (
Angew. Chem.
, 2020
, 132
, 4405–4409
) CrossRef CAS PubMed.
-
(a) B. Maity, C. Zhu, H. Yue, L. Huang, M. Harb, Y. Minenkov, M. Rueping and L. Cavallo, J. Am. Chem. Soc., 2020, 142, 16942–16952 CrossRef CAS PubMed;
(b) L. Huang, T. Ji and M. Rueping, J. Am. Chem. Soc., 2020, 142, 3532–3539 CrossRef CAS PubMed;
(c) A. Dewanji, P. E. Krach and M. Rueping, Angew. Chem., Int. Ed., 2019, 58, 3566–3570 (
Angew. Chem.
, 2019
, 131
, 3604–3608
) CrossRef CAS PubMed;
(d) L. Huang and M. Rueping, Angew. Chem., Int. Ed., 2018, 57, 10333–10337 (
Angew. Chem.
, 2018
, 130
, 10490–10494
) CrossRef CAS;
(e) H. Yue, C. Zhu and M. Rueping, Angew. Chem., Int. Ed., 2017, 57, 1371–1375 (
Angew. Chem.
, 2017
, 130
, 1385–1389
) CrossRef PubMed.
- S. Sun, Y. Duan, R. S. Mega, R. J. Somerville and R. Martin, Angew. Chem., Int. Ed., 2020, 59, 4370–4374 (
Angew. Chem.
, 2020
, 132
, 4400–4404
) CrossRef CAS PubMed.
- H. Jiang, X. Yu, C. G. Daniliuc and A. Studer, Angew. Chem., Int. Ed., 2021, 60, 14399–14404 (
Angew. Chem.
, 2021
, 133
, 14520–14525
) CrossRef CAS PubMed.
- T. Yang, X. Chen, W. Rao and M. Koh, Chem, 2020, 6, 738–751 CAS.
- For reported elegant examples see:
(a) C. A. Vasilopoulos, S. W. Krska and S. S. Stahl, Science, 2021, 372, 398–403 CrossRef PubMed;
(b) L. Zhang, S. Chen, H. He, W. Li, C. Zhu and J. Xie, Chem. Commun., 2021, 57, 9064–9067 RSC;
(c) B. Maity, C. Zhu, M. Rueping and L. Cavallo, ACS Catal., 2021, 11, 13973–13982 CrossRef CAS;
(d) C. Zhu, H. Yue, L. Chu and M. Rueping, Chem. Sci., 2020, 11, 4051–4064 RSC;
(e) P. Fan, Y. Lan, C. Zhang and C. Wang, J. Am. Chem. Soc., 2020, 142, 2180–2186 CrossRef CAS PubMed;
(f) D. X. Shu, L. Huan, Q. Huang and H. Huo, J. Am. Chem. Soc., 2020, 45, 19058–19064 CrossRef PubMed;
(g) L. Guo, H. Tu, S. Zhu and L. Chu, Org. Lett., 2019, 21, 4771–4776 CrossRef CAS PubMed.
- For selected reviews, see:
(a) A. Y. Chan, I. B. Perry, N. B. Bissonnette, B. F. Buksh, G. A. Edwards, L. I. Frye, O. L. Garry, M. N. Lavagnino, B. X. Li, Y. Liang, E. Mao, A. Millet, J. V. Oakley, N. L. Reed, H. A. Sakai, C. P. Seath and D. W. C. MacMillan, Chem. Rev., 2022, 122, 1485–1542 CrossRef CAS PubMed;
(b) B. N. Holmberg-Douglas and D. A. Nicewicz, Chem. Rev., 2022, 122, 1925–2016 CrossRef PubMed;
(c) L. Yi, T. Ji, K.-Q. Chen, X.-Y. Chen and M. Rueping, CCS Chem., 2022, 4, 9–30 CrossRef CAS;
(d) F.-D. Lu, G.-F. He, L.-Q. Lu and W.-J. Xiao, Green Chem., 2021, 23, 5379–5393 RSC;
(e) F.-D. Lu, J. Chen, X. Jiang, J.-R. Chen, L.-Q. Lu and W.-J. Xiao, Chem. Soc. Rev., 2021, 50, 12808–12827 RSC;
(f) S. K. Kariofillis and A. G. Doyle, Acc. Chem. Res., 2021, 54, 988–1000 CrossRef CAS PubMed;
(g) C. Zhu, H. Yue, L. Chu and M. Rueping, Chem. Sci., 2020, 11, 4051–4064 RSC;
(h) H. Cao, X. Tang, H. Tang, Y. Yuan and J. Wu, Chem. Catal., 2021, 1, 523–598 CrossRef;
(i) L. Guillemard and J. Wencel-Delord, Beilstein J. Org. Chem., 2020, 16, 1754–1804 CrossRef CAS PubMed;
(j) W.-J. Zhou, Y.-H. Zhang, Y.-Y. Gui, L. Sun and D.-G. Yu, Synthesis, 2018, 50, 3359–3378 CrossRef CAS;
(k) J. Twilton, C. Le, P. Zhang, M. H. Shaw, R. W. Evans and D. W. C. MacMillan, Nat. Rev. Chem., 2017, 1, 52–69 CrossRef CAS;
(l) K. Skubi, T. V. Blum and T. P. Yoon, Chem. Rev., 2016, 116, 10035–10074 CrossRef CAS PubMed;
(m) Y.-Y. Gui, L. Sun, Z.-P. Lua and D.-G. Yu, Org. Chem. Front., 2016, 3, 522–526 RSC.
- S. Xu, H. Chen, Z. Zhou and W. Kong, Angew. Chem., Int. Ed., 2021, 60, 7405–7411 (
Angew. Chem.
, 2021
, 133
, 7481–7487
) CrossRef CAS PubMed.
-
(a) R. L. Sutar, S. Sen, O. Eivgi, G. Segalovich, I. Schapiro, O. Reany and N. G. Lemcoff, Chem. Sci., 2018, 9, 1368–1374 RSC;
(b) J. Li, M. Kong, B. Qiao, R. Lee, X. Zhao and Z. Jiang, Nat. Commun., 2018, 9, 2445–2453 CrossRef PubMed;
(c) J. T. Edwards, R. R. Merchant, K. S. McClymont, K. W. Knouse, T. Qin, L. R. Malins, B. Vokits, S. A. Shaw, D.-H. Bao, F.-L. Wei, T. Zhou, M. D. Eastgate and P. S. Baran, Nature, 2017, 545, 213–219 CrossRef CAS PubMed;
(d) L. Lin, X. Bai, X. Ye, X. Zhao, C.-H. Tan and Z. Jiang, Angew. Chem., Int. Ed., 2017, 56, 13842–13846 (
Angew. Chem.
, 2017
, 129
, 14030–14034
) CrossRef CAS PubMed;
(e) H. Renata, Q. H. Zhou, G. Dunstl, J. Felding, R. R. Merchant, C. H. Yeh and P. S. Baran, J. Am. Chem. Soc., 2015, 137, 1330–1340 CrossRef CAS PubMed;
(f) A. Démolis, N. Essayem and F. Rataboul, ACS Sustainable Chem. Eng., 2014, 2, 1338–1352 CrossRef;
(g) F. Alois and N. Takashi, J. Am. Chem. Soc., 2007, 129, 1906–1907 CrossRef.
-
(a) Y. Yamamoto, H. Maekawa, S. Goda and I. Nishiguchi, Org. Lett., 2003, 5, 2755–2758 CrossRef CAS PubMed;
(b) Y. Liu and Y. Zhang, Tetrahedron, 2003, 59, 8429–8437 CrossRef CAS;
(c) N.-S. Li, S. Yu and G. W. Kabalka, Organometallics, 1998, 17, 3815–3817 CrossRef CAS.
- T. H. Meyer, L. H. Finger, P. Gandeepan and L. Ackermann, Trends Chem., 2019, 1, 63–76 CrossRef CAS.
-
(a) Y. Matsuki, N. Ohnishi, Y. Kakeno, S. Takemoto, T. Ishii, K. Nagao and H. Ohmiya, Nat. Commun., 2021, 12, 3848–3855 CrossRef CAS PubMed;
(b) Q. Zhang, Y. Huang, L. W. Zhan, W. Y. Tang, J. Hou and B. D. Li, Org. Lett., 2020, 22, 7460–7464 CrossRef CAS PubMed;
(c) H. Zhang, Q. Xiao, X.-K. Qi, X.-W. Gao, Q.-X. Tong and J.-J. Zhong, Chem. Commun., 2020, 56, 12530–12533 RSC;
(d) T. Morack, C. Mck-Lichtenfeld and R. Gilmour, Angew. Chem., Int. Ed., 2019, 58, 1208–1212 (
Angew. Chem.
, 2019
, 131
, 1221–1225
) CrossRef CAS PubMed;
(e) G. Goti, B. Bieszczad, A. Vega-Peñaloza and P. Melchiorre, Angew. Chem., Int. Ed., 2019, 58, 1213–1217 (
Angew. Chem.
, 2019
, 131
, 1226–1230
) CrossRef CAS PubMed;
(f) C. M. Wang, D. Song, P. J. Xia, J. Wang, H. Y. Xiang and H. Yang, Chem.–Asian J., 2018, 13, 271–274 CrossRef CAS PubMed;
(g) M. D. Vu, M. Das and X. W. Liu, Chem.–Eur.
J., 2017, 23, 15899–15902 CrossRef CAS PubMed;
(h) J. A. Walker, K. L. Vickerman, J. N. Humke and L. M. Stanley, J. Am. Chem. Soc., 2017, 139, 10228–10231 CrossRef CAS PubMed;
(i) M. M. D. Wilde and M. Gravel, Angew. Chem., Int. Ed., 2013, 52, 12651–12654 CrossRef CAS PubMed;
(j) Q. Liu, S. Perreault and T. Rovis, J. Am. Chem. Soc., 2008, 130, 14066–14067 CrossRef CAS PubMed;
(k) M. Ischay and T. P. Yoon, Eur. J. Org. Chem., 2012, 3359–3372 CrossRef CAS;
(l) M. C. Myers, A. R. Bharadwaj, B. C. Milgram and K. A. Scheidt, J. Am. Chem. Soc., 2005, 127, 14675–14680 CrossRef CAS PubMed;
(m) A. E. Mattson, A. R. Bharadwaj and K. A. Scheidt, J. Am. Chem. Soc., 2004, 126, 2314–2315 CrossRef CAS PubMed.
-
(a) S. Dong, G. Wu, X. Yuan, C. Zou and J. Ye, Org. Chem. Front., 2017, 4, 2230–2234 RSC;
(b) G. Bergonzini, C. Cassani, H. Lorimer-Olsson, J. Horberg and C.-J. Wallentin, Chem.–Eur. J., 2016, 22, 3292–3295 CrossRef CAS PubMed;
(c) G.-Z. Wang, R. Shang, W.-M. Cheng and Y. Fu, Org. Lett., 2015, 17, 4830–4833 CrossRef CAS PubMed;
(d) G. Bergonzini, C. Cassani and C.-J. Wallentin, Angew. Chem., Int. Ed., 2015, 54, 14066–14069 CrossRef CAS PubMed;
(e) I. Ryu, A. Tani, T. Fukuyama, D. Ravelli, M. Fagnoni and A. Albini, Angew. Chem., Int. Ed., 2011, 50, 1869–1872 (
Angew. Chem.
, 2011
, 123
, 1909–1912
) CrossRef CAS PubMed;
(f) M. T. Wentzel, V. J. Reddy, T. K. Hyster and C. J. Douglas, Angew. Chem., Int. Ed., 2009, 48, 6121–6123 CrossRef.
- For reported elegant examples see:
(a) S. Jin, X. Sui, G. C. Haug, V. D. Nguyen, H. T. Dang, H. D. Arman and O. V. Larionov, ACS Catal., 2022, 12, 285–294 CrossRef CAS;
(b) Y.-Y. Cheng, J.-X. Yu, T. Lei, H.-Y. Hou, B. Chen, C.-H. Tung and L.-Z. Wu, Angew. Chem., Int. Ed., 2021, 60, 26822–26828 (
Angew. Chem.
, 2021
, 133
, 27026–27032
) CrossRef CAS;
(c) K. Liu and A. Studer, J. Am. Chem. Soc., 2021, 143, 4903–4909 CrossRef CAS;
(d) Z. Li, M. Huang, X. Zhang, J. Chen and Y. Huang, ACS Catal., 2021, 11, 10123–10130 CrossRef CAS;
(e) M. Kusakabe, K. Nagao and H. Ohmiya, Org. Lett., 2021, 23, 7242–7247 CrossRef CAS PubMed;
(f) Y. Gao, Y. Quan, Z. Li, L. Gao, Z. Zhang, X. Zou, R. Yan, Y. Qu and K. Guo, Org. Lett., 2021, 23, 183–189 CrossRef CAS PubMed;
(g) C. Zhu, J. Dong, X. Liu, L. Gao, Y. Zhao, J. Xie, S. Li and C. Zhu, Angew. Chem., Int. Ed., 2020, 59, 12817–12821 (
Angew. Chem.
, 2020
, 132
, 12917–12921
) CrossRef CAS PubMed;
(h) L. Chen, S. Jin, J. Gao, T. Liu, Y. Shao, J. Feng, K. Wang, T. Lu and D. Du, Org.
Lett., 2021, 23, 394–399 CrossRef CAS PubMed;
(i) Q.-Y. Meng, N. Dçben and A. Studer, Angew. Chem., Int. Ed., 2020, 59, 19956–19960 (
Angew. Chem.
, 2020
, 132
, 20129–20134
) CrossRef CAS PubMed;
(j) J.-L. Li, Y.-Q. Liu, W.-L. Zou, R. Zeng, X. Zhang, Y. Liu, B. Han, Y. He, H.-J. Leng and Q.-Z. Li, Angew. Chem., Int. Ed., 2020, 59, 1863–1870 (
Angew. Chem.
, 2020
, 132
, 1879–1886
) CrossRef CAS PubMed;
(k) Y. Yuan, F.-P. Wu, J.-X. Xu and X.-F. Wu, Angew. Chem., Int. Ed., 2020, 59, 17055–17061 (
Angew. Chem.
, 2020
, 132
, 17203–17209
) CrossRef CAS PubMed;
(l) H.-B. Yang, Z.-H. Wang, J.-M. Li and C. Wu, Chem. Commun., 2020, 56, 3801–3804 RSC;
(m) L. Wang and C. Wang, Org. Lett., 2020, 22, 8829–8835 CrossRef CAS PubMed;
(n) X. Zhao, B. Li and W. Xia, Org. Lett., 2020, 22, 1056–1061 CrossRef CAS PubMed;
(o) B. Zhang, Q. Peng, D. Guo and J. Wang, Org. Lett., 2020, 22, 443–447 CrossRef CAS PubMed;
(p) T. Ishii, K. Ota, K. Nagao and H. Ohmiya, J. Am. Chem. Soc., 2019, 141, 14073–14077 CrossRef CAS PubMed;
(q) T. Ishii, Y. Kakeno, K. Nagao and H. Ohmiya, J. Am. Chem. Soc., 2019, 141, 3854–3858 CrossRef CAS PubMed;
(r) H. Wang, Y. Wang, X. Chen, C. Mou, S. Yu, H. Chai, Z. Jin and Y. R. Chi, Org. Lett., 2019, 21, 7440–7444 CrossRef CAS PubMed;
(s) X. Zhao, H.-Y. Tu, L. Guo, S. Zhu, F.-L. Qing and L. Chu, Nat. Commun., 2018, 9, 3488–3492 CrossRef;
(t) Z.-Y. Song, K.-Q. Chen, X.-Y. Chen and S. Ye, J. Org. Chem., 2018, 83, 2966–2970 CrossRef CAS PubMed.
- For reported elegant examples see:
(a) X. Wu, Y. Zhang, Y. Wang, J. Ke, M. Jeret, R. N. Reddi, S. Yang, B.-A. Song and Y. R. Chi, Angew. Chem., Int. Ed., 2017, 56, 2942–2946 (
Angew. Chem.
, 2017
, 129
, 2988–2992
) CrossRef CAS;
(b) Y. Huang, K. B. Smith and M. Brown, Angew. Chem., Int. Ed., 2017, 56, 13314–13318 (
Angew. Chem.
, 2017
, 129
, 13499–13503
) CrossRef CAS PubMed;
(c) Y. Wang, Y. Du, X. Huang, X. Wu, Y. Zhang, S. Yang and Y. R. Chi, Org. Lett., 2017, 19, 632–635 CrossRef CAS PubMed;
(d) X.-Y. Chen, K.-Q. Chen, D.-Q. Sun and S. Ye, Chem. Sci., 2017, 8, 1936–1941 RSC;
(e) W. Yang, W. Hu, X. Dong, X. Li and J. Sun, Angew. Chem., Int. Ed., 2016, 55, 15783–15786 (
Angew. Chem.
, 2016
, 128
, 16015–16018
) CrossRef CAS PubMed;
(f) B.-S. Li, Y. Wang, R. S. J. Proctor, Y. Zhang, R. D. Webster, S. Yang, B. Song and Y. R. Chi, Nat. Commun., 2016, 7, 12933–12940 CrossRef PubMed;
(g) N. A. White and T. Rovis, J. Am. Chem. Soc., 2015, 137, 10112–10115 CrossRef CAS PubMed;
(h) Y. Zhang, Y. Du, Z. Huang, J. Xu, X. Wu, Y. Wang, M. Wang, S. Yang, R. D. Webster and Y. R. Chi, J. Am. Chem. Soc., 2015, 137, 2416–2419 CrossRef CAS PubMed;
(i) N. A. White and T. Rovis, J. Am. Chem. Soc., 2014, 136, 14674–14677 CrossRef CAS PubMed;
(j) Y. Du, Y. Wang, X. Li, Y. Shao, G. Li, R. D. Webster and Y. R. Chi, Org. Lett., 2014, 16, 5678–5681 CrossRef CAS PubMed;
(k) K. Takaki, A. Ohno, M. Hino, T. Shitaoka, K. Komeyama and H. Yoshida, Chem. Commun., 2014, 50, 12285–12288 RSC;
(l) J. Guin, S. D. Sarkar, S. Grimme and A. Studer, Angew. Chem., Int. Ed., 2008, 47, 8727–8730 (
Angew. Chem.
, 2008
, 120
, 8855–8858
) CrossRef CAS.
-
(a) D. Mazzarella, A. Pulcinella, L. Bovy, R. Broersma and T. Noël, Angew. Chem., Int. Ed., 2021, 60, 21277–21282 (
Angew. Chem.
, 2020
, 133
, 21447–21452
) CrossRef CAS PubMed;
(b) G. Laudadio, Y. Deng, K. van der Wal, D. Ravelli, M. Nuño, M. Fagnoni, D. Guthrie, Y. Sun and T. Noël, Science, 2020, 369, 92–96 CrossRef CAS PubMed;
(c) L. K. G. Ackerman, J. I. Martinez Alvarado and A. G. Doyle, J. Am. Chem. Soc., 2018, 140, 14059–14063 CrossRef CAS PubMed.
-
(a) A. Ghosh, K. F. Johnson, K. L. Vickerman, J. A. Walker and L. M. Stanley, Org. Chem. Front., 2016, 3, 639–644 RSC;
(b) S. K. Murphy and V. M. Dong, Chem. Commun., 2014, 50, 13645–13649 RSC;
(c) M. C. Willis, Chem. Rev., 2010, 110, 725–748 CrossRef CAS PubMed;
(d) M. Christmann, Angew. Chem., Int. Ed., 2005, 44, 2632–2634 CrossRef CAS PubMed.
- J. D. Earley, A. Zieleniewska, H. H. Ripberger, N. Y. Shin, M. S. Lazorski, Z. J. Mast, H. J. Sayre, J. K. McCusker, G. D. Scholes, R. R. Knowles, O. G. Reid and G. Rumbles, Nat. Chem., 2022 DOI:10.1038/s41557-022-00911-6.
|
This journal is © The Royal Society of Chemistry 2022 |
Click here to see how this site uses Cookies. View our privacy policy here.