DOI:
10.1039/D2SC02205B
(Edge Article)
Chem. Sci., 2022,
13, 7347-7354
RhodiumIII-catalyzed remote difunctionalization of arenes assisted by a relay directing group†
Received
19th April 2022
, Accepted 28th May 2022
First published on 30th May 2022
Abstract
Rhodium-catalyzed diverse tandem twofold C–H bond activation reactions of para-olefin-tethered arenes have been realized, with unsaturated reagents such as internal alkynes, dioxazolones, and isocyanates being the coupling partner as well as a relay directing group which triggers cyclization of the para-olefin group under oxidative or redox-neutral conditions. The reaction proceeded via initial ortho-C–H activation assisted by a built-in directing group in the arene, and the ortho-incorporation of the unsaturated coupling partner simultaneously generated a relay directing group that allows sequential C–H activation at the meta-position and subsequent cyclization of the para-olefins. The overall reaction represents C–C or N–C difunctionalization of the arene with the generation of diverse 2,3-dihydrobenzofuran platforms. The catalytic system proceeded with good efficiency, simple reaction conditions, and broad substrate scope. The diverse transformations of the products demonstrated the synthetic utility of this tandem reaction.
Introduction
Over the last few decades, transition metal catalyzed C–H bond functionalization has been established as a highly effective, step-economy, and easy-to-operate strategy in modern organic chemistry for the construction of various value-added compounds from structurally simple chemicals.1,2 So far, heteroatom-containing chelator moieties have proved competent to this strategy, with ortho-C–H functionalization being the most dominant scenario (Scheme 1a).1 In contrast, the activation of meta-C–H bonds is commonly overruled by proximity-driven ortho-selectivity.3 In 2012, a brilliant solution to this selectivity was introduced by the Yu3 group (Scheme 1b), achieving meta-selective functionalization via the use of a nitrile-containing template as a directing group. This strategy has been subsequently extended to other directing templates and metal catalysts by the groups of Tan, Maiti, Yu and Li.4 In these systems, the linear nitrile-based template preferentially activates the meta-C–H bond, and the weak coordinating nature of the directing template also assists the formation of a macrocyclic organometallic intermediate. Another elegant solution to this selectivity is to use a transient directing group strategy (Scheme 1c), which involves the initial ortho-C–H activation, and subsequently second C–H activation occurs at the meta-C–H bond. In 2015, by using NBE as a transient mediator, the Yu5 group gloriously realized meta-selective alkylation of phenyl acetamide and it was subsequently extended to diverse classes of substrates by the groups of Yu, Dong, Ferreira, Zhou, Zhao and Shi (Scheme 1c).6 In these systems the transient directing group NBE is not incorporated into the final products. Notably, a number of approaches are available for selective sequential ortho- and meta-dual C–H functionalization of arenes (Scheme 1d). In 2008, Miura and coworkers developed a Rh(III)-catalyzed [2 + 2 + 2] aromatic homologation reaction between arenes and alkynes via chelation-assisted dual C–H activation, where the alkyne insertion-derived alkenyl intermediate directs the 2nd C–H activation toward the meta-position.7 In 2013, our group reported the [3 + 2] oxidative annulation of arenes with azabicyclic olefins, where this bicyclic olefin behaved similarly to NBE but it was incorporated into the final product, leading to 1,2-carboamination of the arenes.8 In 2017, dioxazolone was successfully used for multiple amidation of a amide through ruthenium catalysis by the group of Chang.9 The discovery of dual C–H functionalization has opened a new avenue for the construction of various polycyclic scaffolds.10–12 To the best of our knowledge, this attractive strategy is limited to C–H activation-coupling with multiple incorporation of a single unsaturated reagent. In contrast, applications of this strategy to the arenes bearing a tethered coupling reagent at the para-position of the directing group via the metal-catalyzed relay directing group strategy remain unexplored (Scheme 1e). This attractive strategy will allow the realization of molecular complexity via arene difunctionalization with simultaneous incorporation of different functional groups.
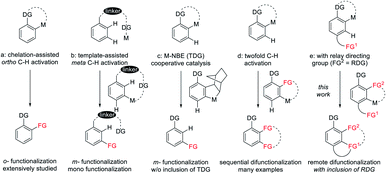 |
| Scheme 1 Metal-catalyzed selective C–H activation strategies. | |
2,3-Dihydrobenzofuran skeletons present a commonly encountered building block of many natural pharmaceuticals and biologically active compounds.13 Nevertheless, the development of a new avenue for the construction of this structural motif remains highly challenging.14 Recently, the intramolecular cyclization of arenes with alkene units tethered at the ortho-position has been well-established for the construction of dihydrobenzofurans by transition metal catalysis (Scheme 2a).15 Later, the tethered olefin-containing arenes were extended to meta-substituted by Yao and coworkers.16 The same strategy was extended to alkyne-tethered arenes by the groups of Luan and García-López.17 Intriguingly, analogous intramolecular annulations have been realized by using meta-olefin-tethered arenes via chelation-assisted C–H activation (Scheme 2b).18 However, this reaction system is limited to the arenes with an olefin unit tethered at the meta- or ortho-position. Herein, we report RhIII-catalyzed C–H activation, insertion of an unsaturated coupling partner (relay directing group), second C–H activation, and intramolecular alkene insertion cascade, leading to efficient synthesis of 2,3-dihydrobenzofuran compounds with a quaternary carbon center (Scheme 2c).
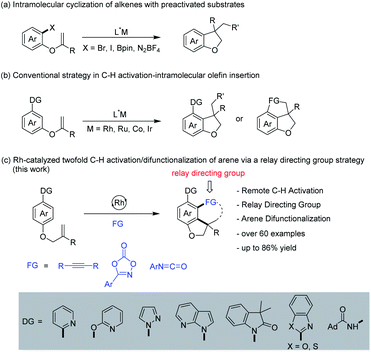 |
| Scheme 2 Metal-catalyzed synthesis of 2,3-dihydrobenzofuran. | |
Results and discussion
2-(4-((2-Methylallyl)oxy)phenyl)pyridine (1a) was designed as a model substrate for coupling with diphenylacetylene (2a) to achieve our proof-of-concept studies under oxidative conditions. As shown in Table 1, the desired carboannulation product 3 was obtained with moderate to good yields in various solvents (entries 1–6), where t-AmOH proved to be the optimal solvent. Decreased yields of product 3 was detected when replacing the AgOAc oxidant with other Ag(I) salts such as AgF, Ag2CO3, and Ag2O (entries 7–9). Commonly used acid and base additives such as Zn(OAc)2, CsOAc, CsOPiv, NaOAc, and HOAc were also screened (entries 10–14). Among them, CsOPiv provided the best result (entry 12, 76%). In addition, under a nitrogen atmosphere, this carboannulation reaction also proceed to give the product in 75% yield (entry 15). The introduction of the AgSbF6 additive (20 mol%) turned out to be detrimental to the reaction efficiency (entry 16 vs. 12).
Table 1 Optimization studiesa
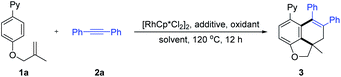
|
Entry |
Additive |
Oxidant |
Solvent |
Yieldb (%) |
Reaction conditions: 1a (0.05 mmol), 2a (0.065 mmol), [RhCp*Cl2]2 (5 mol%), additive (0.5 equiv.), oxidant (2.3 equiv.), and solvent (1.0 mL), at 120 °C under air for 12 h.
Isolated yield.
Not detected.
Under N2.
AgSbF6 (20 mol%) was used.
|
1 |
PivOH |
AgOAc |
THF |
48 |
2 |
PivOH |
AgOAc |
1,4-Dioxane |
26 |
3 |
PivOH |
AgOAc |
DCE |
Trace |
4 |
PivOH |
AgOAc |
MeOH |
NDc |
5 |
PivOH |
AgOAc |
PhMe |
41 |
6 |
PivOH |
AgOAc |
t-AmOH |
68 |
7 |
PivOH |
AgF |
t-AmOH |
40 |
8 |
PivOH |
Ag2CO3 |
t-AmOH |
30 |
9 |
PivOH |
Ag2O |
t-AmOH |
15 |
10 |
Zn(OAc)2 |
AgOAc |
t-AmOH |
35 |
11 |
CsOAc |
AgOAc |
t-AmOH |
69 |
12 |
CsOPiv |
AgOAc |
t-AmOH |
76 |
13 |
NaOAc |
AgOAc |
t-AmOH |
70 |
14 |
HOAc |
AgOAc |
t-AmOH |
65 |
15d |
PivOH |
AgOAc |
t-AmOH |
75 |
16e |
PivOH |
AgOAc |
t-AmOH |
40 |
With the optimized reaction conditions in hand (Table 1, entry 12), we then explored the scope and limitation of alkynes (Scheme 3). The reaction occurred smoothly for symmetrical diarylalkynes to afford the expected products 3–9 in good yields (63–82%). The introduction of alkyl, methoxy, and halogen substituents into the para-position of the phenyl ring exerted little effect on the reaction efficiency. The presence of 4-CF3 and 4-CO2Et at the para-position were also tolerated (10 and 11). In contrast, 4-CN substituted symmetrical diarylalkyne (2j) only reacted with low efficiency (12) possibly due to the coordinating effect. Comparable efficiency was realized for various substitutions at the meta-position of the aryl ring (13–18). In addition, when disubstituted (2q) and 2-naphthyl-substituted alkynes (2r) were employed, the corresponding carboannulation products 19 and 20 were also obtained with good yields. Several heterocycle-substituted alkynes can also deliver the desired products 21 and 22 in 52% and 43% yields. The extension of the alkynes to unsymmetrical ones also proved to be successful, affording the products 23, 27, and 28 in moderate yields with excellent regioselectivities. The reaction efficiency is sensitive to the length of alkyl chains in the unsymmetrical alkynes (24–26). The regioselectivity of product 25 had been determined by NOESY analysis (see the ESI† for details). However, no product was detected when phenylacetylene, dialkyl alkyne or substituted 1,3-enyne was used.
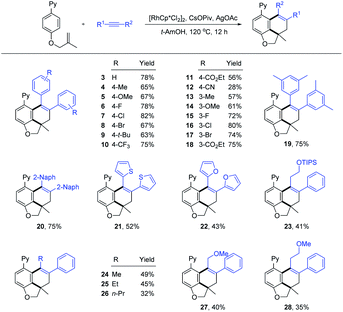 |
| Scheme 3 Substrate scope of alkynesa. a Reaction conditions: 1a (0.20 mmol), alkyne (0.26 mmol), [RhCp*Cl2]2 (5 mol%), CsOPiv (0.10 mmol, 0.5 equiv.), AgOAc (0.46 mmol, 2.3 equiv.), and t-AmOH (2 mL), at 120 °C under air for 12 h, and isolated yield. | |
Next, the carboannulation reactions of 2a with different olefin-tethered arenes were carried out (Scheme 4). It was found that pyridine rings bearing 5-Me and 5-OMe groups reacted smoothly to deliver the corresponding products (29 and 30) in good yields (72% and 68%). However, the introduction of halogen or CF3 substituents into the 5-position of the pyridine ring met with difficulty under the original conditions. To our delight, the coupling reaction proceeded with moderate yields by using DCE as the solvent instead of t-AmOH (31–33). Substrates with Me and OMe attached on the 4-position of the pyridine ring did not significantly affect the reaction efficiency (34 and 35). The 4-fluoro or 4-chloro substituted pyridine ring exhibited poor efficiency with 36% and 39% yield (36 and 37), respectively. By replacing the olefin unit from the methyl group, the desired products (38–40) could also be obtained in good yields. Furthermore, when 1-substituted isoquinoline-directed arene 1n was checked, inseparable diastereoisomers 41 were achieved in a 1.1
:
1 ratio with 49% yield. Employing the 3-substituted isoquinoline as the directing group led to the formation of product 42 (49% yield). In addition to forming benzo-dihydrobenzofurans, other intriguing benzo-heterocycles were all accessed by altering the tethering group attached to the alkene (43 and 44, 67% and 51%, respectively). However, the terminal alkene and C-tethered alkene substituted substrates exhibited poor efficiency with 21% and 19% yield (45 and 46). When arenes with nitrogen atoms were used as a linker or 1,2-disubstituted alkene, no desired annulation products were detected (see the ESI†).
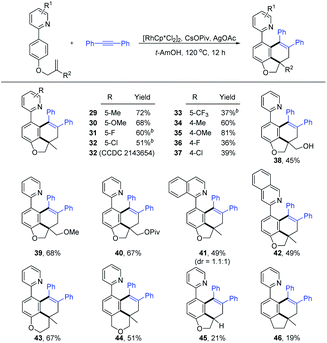 |
| Scheme 4 Substrate scope of arenesa. a Reaction conditions: arene (0.20 mmol), 2a (0.26 mmol), [RhCp*Cl2]2 (5 mol%), CsOPiv (0.10 mmol, 0.5 equiv.), AgOAc (0.46 mmol, 2.3 equiv.), and t-AmOH (2 mL), at 120 °C under air for 12 h, and isolated yield. b DCE (2 mL) was used. | |
To further highlight the generality of our tandem reactions, a detailed study on the reactivity of various directing groups was conducted, which showed wide compatibility of coordinating functionality (Scheme 5). For example, using pyridyloxyl as the auxiliary group, the C–H activation/intramolecular remote difunctionalization of arenes delivered the desired coupling product 47 in 76% yield. In addition, the directing group has been successfully extended to pyrazole, 7-azaindole, oxindole, benzooxazole, benzothiazole and amide group (48–53 and 32–85% yield). In the case of couplings using a sterically bulky heteroaryl directing group, the reaction created an additional chiral axis but with poor diastereoselectivity (49 and 50). Subsequently, by using Cramer's second generation chiral (R)-Rh catalyst,2i,19 a preliminary investigation of the enantioselective version of the annulation of arene 1t with alkyne 2a was conducted (see the ESI† for details). The desired product 47 was obtained with 20% yield and 34% ee. These preliminary results provided possibilities for further studies on asymmetric tandem reactions.
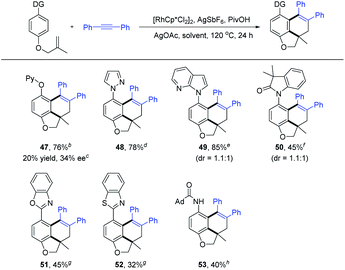 |
| Scheme 5 Substrate scope of directing groupsa. a Reaction conditions: arene (0.20 mmol), 2a (0.24 mmol), [RhCp*Cl2]2 (5 mol%), AgSbF6 (20 mol%), PivOH (1.0 eq.), AgOAc (2.3 eq.), 120 °C, air, 24 h, and isolated yield. b TFE (2.0 mL) was used and 100 °C. c (R)-Rh (2.5 mol%), AgSbF6 (10 mol%), PivOH (2.0 eq.) and MeOH (2.0 mL) were used, 80 °C, and 48 h. d NaOAc (1.0 eq.) and 1,4-dioxane (2.0 mL) were used and 80 °C. e MeOH (2.0 mL) was used. f AgF (2.3 eq.) and THF (2.0 mL) were used. g NaOAc (1.0 eq.) and THF (2.0 mL) were used. ht-AmOH (2.0 mL) was used. | |
Dioxazolones have been successfully employed as amidating reagents or directing groups in C–H activation.9,20 We envisaged an iterative C–H functionalization strategy, where the installed amide group from dioxazolone serves as the next coordinating group (relay directing group) for the subsequent C–H functionalization event. We found that the C–H amidation/intramolecular cyclization proceeded smoothly to afford the expected product 54 by treating 1a with 3-phenyl-1,4,2-dioxazol-5-one (2zb) in HFIP in the presence of [Cp*RhCl2]2, AgSbF6 and PivOH at 110 °C (see Table S9 in the ESI† for more details). Next, we explored the substrate scope and limitation of this iterative C–H functionalization (Scheme 6). The dioxazolones bearing 4-Me, 4-OMe, 4-t-Bu, 4-F, 4-Cl and 4-CF3 groups on the phenyl ring all reacted smoothly to deliver the carboannulation products (55–60) in 40–66% yields. When naphtho- and furo-substituted dioxazolones were employed, the corresponding products 61 and 62 were obtained with 44% and 40% yields, respectively. In contrast, Me or t-Bu substituted dioxazolone failed to undergo the carboannulation coupling.
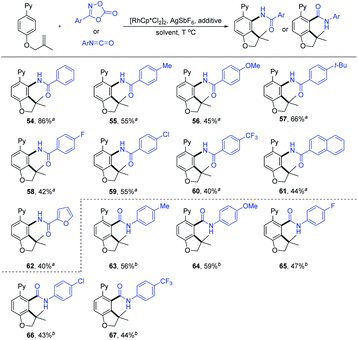 |
| Scheme 6 Substrate scope of unsaturated reagents. Reaction conditions: a1a (0.20 mmol), 2 (0.26 mmol), [RhCp*Cl2]2 (5 mol%), AgSbF6 (20 mol%), PivOH (0.40 mmol, 2.0 equiv.), and HFIP (2 mL), at 110 °C under air for 36 h, and isolated yield. b1a (0.20 mmol), 2 (0.24 mmol), [RhCp*Cl2]2 (5 mol%), AgSbF6 (20 mol%), AgOAc (0.20 mmol, 1.0 equiv.), and DCM (2 mL), at 75 °C under N2 for 24 h, and isolated yield. | |
To better define the scope of the relay directing group, isocyanates21 were explored as the coupling reagent that is expected to generate an intrinsic amide directing group (Scheme 6). The coupling of 1a with isocyanatobenzene bearing a para-Me group under modified conditions afforded product 63 in 56% yield (see Table S10 in the ESI†). The scope of the isocyanates was also briefly examined, and 4-OMe, 4-F, 4-Cl and 4-CF3 groups on the phenyl ring were all compatible, delivering the carboannulation products (64–67) in 43–59% yields. However, no product was detected when N-Et or -Ts substituted isocyanate was used, suggesting the influence of the electronic effect of the isocyanate reagent.
To explore the practicability of this tandem reaction (Scheme 7), a gram-scale reaction was performed under a reduced catalyst loading (2.5 mol%), affording the product 3 in 79% yield. To show the synthetic utility of the present protocol, the synthetic transformations of compound 3 were conducted. Rh(III)-catalyzed coupling of 3 with a variety of unsaturated coupling partners afforded various C–H functionalization products in 56–71% yields (68–71) with the assistance of the initial directing group. The rhodium-catalyzed oxidative C–H annulation reaction of 3 with 2a gave the expected quaternary ammonium salt 72 in 97% yield, which may find wild application as numerous biologically active compounds or functional molecules.22
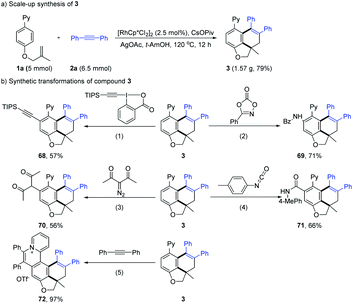 |
| Scheme 7 Synthetic transformations of compounds 3. Reaction conditions: (1) 3, 1-(triisopropylsilyl)ethynyl-1,2-benziodoxol-3(1H)-one (TIPS-EBX), [RhCp*Cl2]2, AgSbF6, MeOH, 60 °C, and 24 h. (2) 3, 3-phenyl-1,4,2-dioxazol-5-one (2zb), [RhCp*Cl2]2, AgSbF6, DCE, 80 °C, and 24 h. (3) 3, 3-diazopentane-2,4-dione, [RhCp*Cl2]2, AgSbF6, KOAc, DCE, 80 °C, N2, and 24 h. (4) 3, 1-isocyanato-4-methylbenzene, [RhCp*Cl2]2, AgSbF6, DCM, 80 °C, N2, and 12 h. (5) 3, 1,2-diphenylethyne, [RhCp*Cl2]2, AgSbF6, AgOTf, AgOAc, MeOH, 120 °C, N2, and 24 h. | |
To elucidate the mechanism of the tandem reaction, we carried out a set of control experiments (Scheme 8). H-D exchange studies of 1a were conducted using t-AmOD as the medium in the absence of 2a, where 1a-d2 was recovered in 96% yield. 1H NMR analysis indicated slight deuteration (20% D) at the ortho-positions of the 2-phenyl unit. In addition, another H/D exchange experiment in the presence of 2a was also carried out. The product 3 was detected to be less than 5% deuteration, suggesting the reversibility of C–H activation of substrate 1a. A five-membered rhodacyclic species A (CCDC 2158590†) was prepared from 1a and [Cp*RhCl2]2. By using rhodacyclic complex A as a catalyst, the coupling of 1a with 2a gave the desired product in 77% yield. The stoichiometric reaction of complex A with 2a also proceeded smoothly under oxidative or oxidant-free conditions. Finally, the treatment of alkenylation product 73 (see the ESI† for more details) under standard conditions did not afford the corresponding product 48, indicating that alkenylation product 73 was not a reaction intermediate in the tandem reaction system. Thus, the alkyne-insertion-derived rhodium alkenyl intermediate is suggested as a plausible intermediate.
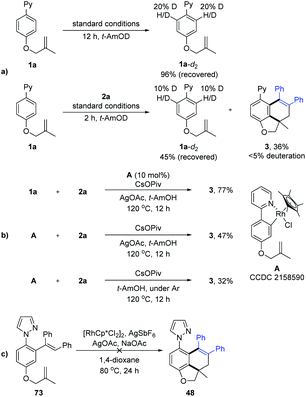 |
| Scheme 8 Preliminary mechanistic studies. | |
On the basis of our above studies and previously related reports,7,10,18 a plausible mechanism is proposed to account for the present catalytic tandem reaction (Scheme 9). The reaction catalytic cycle starts from coordination of the directing group of 1a to the Rh(III) catalyst species. The ortho-C–H bond activation would generate a five-membered rhodacyclic species A, which then reacts with alkyne 2a to give a seven-membered intermediate Bvia alkyne insertion. Intermediate B would produce intermediate Cvia dissociation of the N–Rh bond. Subsequent rollover C–H activation affords intermediate Dvia meta-C–H bond activation. Then, the insertion of an alkene and subsequent C–C reductive elimination would afford the corresponding product 3 and a Rh(I) species. Finally, the oxidation of the Rh(I) species by the stoichiometric Ag(I) oxidant regenerates the active Rh(III) catalyst for the next catalytic cycle. In the case of coupling using dioxazolone or isocyanate, the 1st C–H functionalization generates an amide species that functions as a relay directing group that enables subsequent olefin insertion–hydroarylation under redox-neutral conditions.
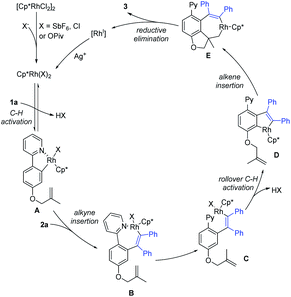 |
| Scheme 9 Plausible mechanism. | |
Conclusions
In summary, we have developed an efficient and straightforward protocol for the synthesis of 2,3-benzofuran compounds with a quaternary carbon center under oxidative or redox-neutral conditions. The reaction proceeded via initial ortho-C–H activation assisted by a built-in directing group in the arene, and the ortho-incorporation of the unsaturated coupling partner simultaneously generated a relay directing group that triggers the 2nd C–H activation at the meta-position followed by subsequent cyclization of the para-olefins. In addition, the diverse transformations of the products demonstrated the synthetic utility of this tandem reaction. Further development of the asymmetrical version of related tandem reactions of metal-catalyzed multiple C–H activation is underway in our laboratory.
Data availability
Further details of the experimental procedure, 1H, 13C and 19F NMR, HPLC spectra, and X-ray crystallographic data for 32 and A are available in the ESI.†
Author contributions
X. L. conceived the idea and guided the project. L. S. performed the experiments and analyzed the results. Y. Z. repeated data. X. L., J. C. and B. L. supervised the project. X. L., L. S. and B. L. co-wrote the manuscript.
Conflicts of interest
The authors declare no competing financial interest.
Acknowledgements
We acknowledge the financial support for this work from the National Key R&D Program of China (2021YFC0864600), the NSFC (No. 21801066, U1804283 and 82130103), and the Central Plains Scholars and Scientists Studio Fund (2018002).
Notes and references
- For selected reviews of transition-metal-catalyzed C–H functionalization, see:
(a) L. McMurray, F. O'Hara and M. J. Gaunt, Chem. Soc. Rev., 2011, 40, 1885–1898 RSC;
(b) S. H. Cho, J. Y. Kim, J. Kwak and S. Chang, Chem. Soc. Rev., 2011, 40, 5068–5083 RSC;
(c) L. Ackermann, Chem. Rev., 2011, 111, 1315–1345 CrossRef CAS PubMed;
(d) B.-J. Li and Z.-J. Shi, Chem. Soc. Rev., 2012, 41, 5588–5598 RSC;
(e) P. B. Arockiam, C. Bruneau and P. H. Dixneuf, Chem. Rev., 2012, 112, 5879–5918 CrossRef CAS PubMed;
(f) Z. Shi, D. C. Koester, M. Boultadakis-Arapinis and F. Glorius, J. Am. Chem. Soc., 2013, 135, 12204–12207 CrossRef CAS PubMed;
(g) Z. Chen, B. Wang, J. Zhang, W. Yu, Z. Liu and Y. Zhang, Org. Chem. Front., 2015, 2, 1107–1295 RSC;
(h) G. Song and X. Li, Acc. Chem. Res., 2015, 48, 1007–1020 CrossRef CAS PubMed;
(i) T. Gensch, M. N. Hopkinson, F. Glorius and J. Wencel-Delord, Chem. Soc. Rev., 2016, 45, 2900–2936 RSC;
(j) G. Cera and L. Ackermann, Top. Curr. Chem., 2016, 374, 191 Search PubMed;
(k) Y. Yang, J. Lan and J. You, Chem. Rev., 2017, 117, 8787–8863 CrossRef CAS PubMed;
(l) J. R. Hummel, J. A. Boerth and J. A. Ellman, Chem. Rev., 2017, 117, 9163–9227 CrossRef CAS PubMed;
(m) C. Sambiagio, D. Schönbauer, R. Blieck, T. Dao-Huy, G. Pototschnig, P. Schaaf, T. Wiesinger, M. F. Zia, J. Wencel-Delord, T. Besset, B. U. W. Maes and M. Schnürch, Chem. Soc. Rev., 2018, 47, 6603–6743 RSC;
(n) P. Gandeepan, T. Müller, D. Zell, G. Cera, S. Warratz and L. Ackermann, Chem. Rev., 2019, 119, 2192–2452 CrossRef CAS PubMed;
(o) U. Dutta, S. Maiti, T. Bhattacharya and D. Maiti, Science, 2021, 372 DOI:10.1126/science.abd5992;
(p) X. Yu, Z.-Z. Zhang, J.-L. Niu and B.-F. Shi, Org. Chem. Front., 2022, 9, 1458–1484 RSC.
- For selected examples, see:
(a) K. Ueura, T. Satoh and M. Miura, Org. Lett., 2007, 9, 1407–1409 CrossRef CAS PubMed;
(b) K. Ueura, T. Satoh and M. Miura, J. Org. Chem., 2007, 72, 5362–5367 CrossRef CAS PubMed;
(c) S. Rakshit, C. Grohmann, T. Besset and F. Glorius, J. Am. Chem. Soc., 2011, 133, 2350–2353 CrossRef CAS PubMed;
(d) N. Guimond, S. I. Gorelsky and K. Fagnou, J. Am. Chem. Soc., 2011, 133, 6449–6457 CrossRef CAS PubMed;
(e) T. K. Hyster, L. Knörr, T. R. Ward and T. Rovis, Science, 2012, 338, 500–503 CrossRef CAS PubMed;
(f) T. K. Hyster, D. M. Dalton and T. Rovis, Chem. Sci., 2015, 6, 254–258 RSC;
(g) N. Semakul, K. E. Jackson, R. S. Paton and T. Rovis, Chem. Sci., 2017, 8, 1015–1020 RSC;
(h) T. Piou, F. Romanov-Michailidis, M. Romanova-Michaelides, K. E. Jackson, N. Semakul, T. D. Taggart, B. S. Newell, C. D. Rithner, R. S. Paton and T. Rovis, J. Am. Chem. Soc., 2017, 139, 1296–1310 CrossRef CAS PubMed;
(i) B. Ye and N. Cramer, Science, 2012, 338, 504–506 CrossRef CAS PubMed;
(j) M. D. Wodrich, B. Ye, J. F. Gonthier, C. Corminboeuf and N. Cramer, Chem.–Eur. J., 2014, 20, 15409–15418 CrossRef CAS PubMed;
(k) D. Wang, F. Wang, G. Song and X. Li, Angew. Chem., Int. Ed., 2012, 51, 12348–12352 CrossRef CAS PubMed;
(l) Z. Qi, M. Wang and X. Li, Chem. Commun., 2014, 50, 9776–9778 RSC;
(m) T. T. Nguyen, L. Grigorjeva and O. Daugulis, Angew. Chem., Int. Ed., 2018, 57, 1688–1691 CrossRef CAS PubMed.
- D. Leow, G. Li, T.-S. Mei and J.-Q. Yu, Nature, 2012, 486, 518–522 CrossRef CAS PubMed.
-
(a) S. Lee, H. Lee and K. L. Tan, J. Am. Chem. Soc., 2013, 135, 18778–18781 CrossRef CAS PubMed;
(b) R.-Y. Tang, G. Li and J.-Q. Yu, Nature, 2014, 507, 215–220 CrossRef CAS PubMed;
(c) G. Yang, P. Lindovska, D. Zhu, J. Kim, P. Wang, R.-Y. Tang, M. Movassaghi and J.-Q. Yu, J. Am. Chem. Soc., 2014, 136, 10807–10813 CrossRef CAS PubMed;
(d) M. Bera, A. Modak, T. Patra, A. Maji and D. Maiti, Org. Lett., 2014, 16, 5760–5763 CrossRef CAS PubMed;
(e) M. Bera, A. Maji, S. K. Sahoo and D. Maiti, Angew. Chem., Int. Ed., 2015, 54, 8515–8519 CrossRef CAS PubMed;
(f) L. Chu, M. Shang, K. Tanaka, Q. Chen, N. Pissarnitski, E. Streckfuss and J.-Q. Yu, ACS Cent. Sci., 2015, 1, 394–399 CrossRef CAS PubMed;
(g) T. Patra, R. Watile, S. Agasti, T. Naveen and D. Maiti, Chem. Commun., 2016, 52, 2027–2030 RSC;
(h) S. Li, L. Cai, H. Ji, L. Yang and G. Li, Nat. Commun., 2016, 7, 10443–10450 CrossRef PubMed;
(i) A. Maji, B. Bhaskararao, S. Singha, R. B. Sunoj and D. Maiti, Chem. Sci., 2016, 7, 3147–3153 RSC;
(j) M. Bera, S. K. Sahoo and D. Maiti, ACS Catal., 2016, 6, 3575–3579 CrossRef CAS;
(k) H.-J. Xu, Y. Lu, M. E. Farmer, H.-W. Wang, D. Zhao, Y.-S. Kang, W.-Y. Sun and J.-Q. Yu, J. Am. Chem. Soc., 2017, 139, 2200–2203 CrossRef CAS PubMed;
(l) S. Bag, R. Jayarajan, R. Mondal and D. Maiti, Angew. Chem., Int. Ed., 2017, 56, 3182–3186 CrossRef CAS PubMed;
(m) M. Bera, S. Agasti, R. Chowdhury, R. Mondal, D. Pal and D. Maiti, Angew. Chem., Int. Ed., 2017, 56, 5272–5276 CrossRef CAS PubMed;
(n) U. Dutta, A. Modak, B. Bhaskararao, M. Bera, S. Bag, A. Mondal, D. W. Lupton, R. B. Sunoj and D. Maiti, ACS Catal., 2017, 7, 3162–3168 CrossRef CAS;
(o) L. Fang, T. G. Saint-Denis, B. L. H. Taylor, S. Ahlquist, K. Hong, S. S. Liu, L. L. Han, K. N. Houk and J.-Q. Yu, J. Am. Chem. Soc., 2017, 139, 10702–10714 CrossRef CAS PubMed;
(p) H.-J. Xu, Y.-S. Kang, H. Shi, P. Zhang, Y.-K. Chen, B. Zhang, Z.-Q. Liu, J. Zhao, W.-Y. Sun, J.-Q. Yu and Y. Lu, J. Am. Chem. Soc., 2019, 141, 76–79 CrossRef CAS PubMed;
(q) T. K. Achar, X. Zhang, R. Mondal, M. S. Shanavas, S. Maiti, S. Maity, N. Pal, R. S. Paton and D. Maiti, Angew. Chem., Int. Ed., 2019, 58, 10353–10360 CrossRef CAS PubMed;
(r) S. Li, H. Wang, Y. Weng and G. Li, Angew. Chem., Int. Ed., 2019, 58, 18502–18507 CrossRef CAS PubMed;
(s) S. Porey, X. Zhang, S. Bhowmick, V. K. Singh, S. Guin, R. S. Paton and D. Maiti, J. Am. Chem. Soc., 2020, 142, 3762–3774 CrossRef CAS PubMed;
(t) S. Bag, K. S., A. Mondal, R. Jayarajan, U. Dutta, S. Porey, R. B. Sunoj and D. Maiti, J. Am. Chem. Soc., 2020, 142, 12453–12466 CrossRef CAS PubMed;
(u) S. Bag, S. Jana, S. Pradhan, S. Bhowmick, N. Goswami, S. K. Sinha and D. Maiti, Nat. Commun., 2021, 12, 1393 CrossRef CAS PubMed.
- X.-C. Wang, W. Gong, L.-Z. Fang, R.-Y. Zhu, S. Li, K. M. Engle and J.-Q. Yu, Nature, 2015, 519, 334–338 CrossRef CAS PubMed.
-
(a) Z. Dong, J. Wang and G. Dong, J. Am. Chem. Soc., 2015, 137, 5887–5890 CrossRef CAS PubMed;
(b) P. Wang, M. E. Farmer, X. Huo, P. Jain, P.-X. Shen, M. Ishoey, J. E. Bradner, S. R. Wisniewski, M. D. Eastgate and J.-Q. Yu, J. Am. Chem. Soc., 2016, 138, 9269–9276 CrossRef CAS PubMed;
(c) J. Han, L. Zhang, Y. Zhu, Y. Zheng, X. Chen, Z.-B. Huang, D.-Q. Shi and Y. Zhao, Chem. Commun., 2016, 52, 6903–6906 RSC;
(d) Q. Li and E. M. Ferreira, Chem.–Eur. J., 2017, 23, 11519–11523 CrossRef CAS PubMed;
(e) Q. Ding, S. Ye, G. Cheng, P. Wang, M. E. Farmer and J.-Q. Yu, J. Am. Chem. Soc., 2017, 139, 417–425 CrossRef CAS PubMed;
(f) G.-C. Li, P. Wang, M. E. Farmer and J.-Q. Yu, Angew. Chem., Int. Ed., 2017, 56, 6874–6877 CrossRef CAS PubMed;
(g) G. Cheng, P. Wang and J.-Q. Yu, Angew. Chem., Int. Ed., 2017, 56, 8183–8186 CrossRef CAS PubMed;
(h) M. E. Farmer, P. Wang, H. Shi and J.-Q. Yu, ACS Catal., 2018, 8, 7362–7367 CrossRef CAS PubMed;
(i) H. Shi, A. N. Herron, Y. Shao, Q. Shao and J.-Q. Yu, Nature, 2018, 558, 581–585 CrossRef CAS PubMed;
(j) J. Wang and G. Dong, Chem. Rev., 2019, 119, 7478–7528 CrossRef CAS PubMed;
(k) Z. Wu, N. Fatuzzo and G. Dong, J. Am. Chem. Soc., 2020, 142, 2715–2720 CrossRef CAS PubMed;
(l) R. Li and G. Dong, J. Am. Chem. Soc., 2020, 142, 17859–17875 CrossRef CAS PubMed.
- N. Umeda, H. Tsurugi, T. Satoh and M. Miura, Angew. Chem., Int. Ed., 2008, 47, 4019–4022 CrossRef CAS PubMed.
- Z. Qi and X. Li, Angew. Chem., Int. Ed., 2013, 52, 8995–9000 CrossRef CAS PubMed.
- J. Park, J. Lee and S. Chang, Angew. Chem., Int. Ed., 2017, 56, 4256–4260 CrossRef CAS PubMed.
-
(a) S. Mochida, M. Shimizu, K. Hirano, T. Satoh and M. Miura, Chem.–Asian J., 2010, 5, 847–851 CrossRef CAS PubMed;
(b) J. Wu, X. Cui, X. Mi, Y. Li and Y. Wu, Chem. Commun., 2010, 46, 6771–6773 RSC;
(c) N. Umeda, K. Hirano, T. Satoh, N. Shibata, H. Sato and M. Miura, J. Org. Chem., 2011, 76, 13–24 CrossRef CAS PubMed;
(d) G. Song, X. Gong and X. Li, J. Org. Chem., 2011, 76, 7583–7589 CrossRef CAS PubMed;
(e) Z. Shi, C. Tang and N. Jiao, Adv. Synth. Catal., 2012, 354, 2695–2700 CrossRef CAS;
(f) Z.-C. Qian, J. Zhou, B. Li and B.-F. Shi, Synlett, 2014, 25, 1036–1040 CrossRef CAS;
(g) J. Zheng and S.-L. You, Chem. Commun., 2014, 50, 8204–8207 RSC;
(h) J. Jia, J. Shi, J. Zhou, X. Liu, Y. Song, H. E. Xu and W. Yi, Chem. Commun., 2015, 51, 2925–2928 RSC;
(i) L. Shi, X. Zhong, H. She, Z. Lei and F. Li, Chem. Commun., 2015, 51, 7136–7139 RSC;
(j) S.-S. Li, C.-Q. Wang, H. Lin, X.-M. Zhang and L. Dong, Org. Lett., 2015, 17, 3018–3021 CrossRef CAS PubMed;
(k) Á. M. Martínez, J. Echavarren, I. Alonso, N. Rodríguez, R. G. Arrayás and J. C. Carretero, Chem. Sci., 2015, 6, 5802–5814 RSC;
(l) L. C. M. Castro, A. Obata, Y. Aihara and N. Chatani, Chem.–Eur. J., 2016, 22, 1362–1367 CrossRef PubMed;
(m) K. Fukuzumi, Y. Unoh, Y. Nishii, T. Satoh, K. Hirano and M. Miura, J. Org. Chem., 2016, 81, 2474–2481 CrossRef CAS PubMed;
(n) P. Annamalai, W.-Y. Chen, S. Raju, K.-C. Hsu, N. S. Upadhyay, C.-H. Cheng and S.-C. Chuang, Adv. Synth. Catal., 2016, 358, 3642–3648 CrossRef CAS;
(o) X. Zhang, X. Yu, D. Ji, Y. Yamamoto, A. I. Almansour, N. Arumugam, R. S. Kumar and M. Bao, Org. Lett., 2016, 18, 4246–4249 CrossRef CAS PubMed;
(p) Z. He and Y. Huang, ACS Catal., 2016, 6, 7814–7823 CrossRef CAS;
(q) L. Wang, Y. Yu, M. Yang, C. Kuai, D. Cai, J. Yu and X. Cui, Adv. Synth. Catal., 2017, 359, 3818–3825 CrossRef CAS;
(r) A. Biswas, D. Giri, D. Das, A. De, S. K. Patra and R. Samanta, J. Org. Chem., 2017, 82, 10989–10996 CrossRef CAS PubMed;
(s) K. R. Bettadapur, R. Kapanaiah, V. Lanke and K. R. Prabhu, J. Org. Chem., 2018, 83, 1810–1818 CrossRef CAS PubMed;
(t) X. Xu, H. Zhao, J. Xu, C. Chen, Y. Pan, Z. Luo, Z. Zhang, H. Li and L. Xu, Org. Lett., 2018, 20, 3843–3847 CrossRef CAS PubMed;
(u) Q. Li, Y. Wang, B. Li and B. Wang, Org. Lett., 2018, 20, 7884–7887 CrossRef CAS PubMed;
(v) H. Li, X. Yan, J. Zhang, W. Guo, J. Jiang and J. Wang, Angew. Chem., Int. Ed., 2019, 58, 6732–6736 CrossRef CAS PubMed;
(w) E. Tomita, K. Yamada, Y. Shibata, K. Tanaka, M. Kojima, T. Yoshino and S. Matsunaga, Angew. Chem., Int. Ed., 2020, 59, 10474–10478 CrossRef CAS PubMed;
(x) X. Yan, J. Jiang and J. Wang, Angew. Chem., Int. Ed., 2022 DOI:10.1002/anie.202201522.
-
(a) R. Mi, G. Zheng, Z. Qi and X. Li, Angew. Chem., Int. Ed., 2019, 58, 17666–17670 CrossRef CAS PubMed;
(b) S. V. Kumar, S. Banerjee and T. Punniyamurthy, Org. Chem. Front., 2019, 6, 3885–3890 RSC;
(c) S. Banerjee, S. V. Kumar and T. Punniyamurthy, J. Org. Chem., 2020, 85, 2793–2805 CrossRef CAS PubMed.
- C.-J. Zhou, H. Gao, S.-L. Huang, S.-S. Zhang, J.-Q. Wu, B. Li, X. Jiang and H. Wang, ACS Catal., 2019, 9, 556–564 CrossRef CAS.
-
(a) K. C. Nicolaou, S. A. Snyder, N. Giuseppone, X. Huang, M. Bella, M. V. Reddy, P. B. Rao, A. E. Koumbis, P. Giannakakou and A. O'Brate, J. Am. Chem. Soc., 2004, 126, 10174–10182 CrossRef CAS PubMed;
(b) M. Naguib, P. Diaz, J. J. Xu, F. Astruc-Diaz, S. Craig, P. VivasMejia and D. L. Brown, Br. J. Pharmacol., 2008, 155, 1104–1116 CrossRef CAS PubMed;
(c) P. Diaz, S. S. Phatak, J. Xu, F. R. Fronczek, F. Astruc-Diaz, C. M. Thompson, C. N. Cavasotto and M. Naguib, ChemMedChem, 2009, 4, 1615–1629 CrossRef CAS PubMed;
(d) M. Naguib, J. J. Xu, P. Diaz, D. L. Brown, D. Cogdell, B. Bie, J. Hu, S. Craig and W. N. Hittelman, Anesth. Analg., 2012, 114, 1104–1120 CrossRef CAS PubMed;
(e) F. Astruc-Diaz, S. W. McDaniel, J. J. Xu, S. Parola, D. L. Brown, M. Naguib and P. Diaz, J. Pharm. Sci., 2013, 102, 352–364 CrossRef CAS PubMed.
-
(a) T. D. Sheppard, J. Chem. Res., 2011, 35, 377–385 CrossRef CAS;
(b) A. Peneau, C. Guillou and L. Chabaud, Eur. J. Org. Chem., 2018, 5777–5794 CrossRef CAS.
-
(a) S. G. Newman, J. K. Howell, N. Nicolaus and M. Lautens, J. Am. Chem. Soc., 2011, 133, 14916–14919 CrossRef CAS PubMed;
(b) H. Cong and G. C. Fu, J. Am. Chem. Soc., 2014, 136, 3788–3791 CrossRef CAS PubMed;
(c) W. You and M. K. Brown, J. Am. Chem. Soc., 2015, 137, 14578–14581 CrossRef CAS PubMed;
(d) Z. M. Zhang, B. Xu, Y. Qian, L. Wu, Y. Wu, L. Zhou, Y. Liu and J. Zhang, Angew. Chem., Int. Ed., 2018, 57, 10373–10377 CrossRef CAS PubMed;
(e) Z. M. Zhang, B. Xu, L. Wu, L. Zhou, D. Ji, Y. Liu, Z. Li and J. Zhang, J. Am. Chem. Soc., 2019, 141, 8110–8115 CrossRef CAS PubMed;
(f) R. C. Carmona, O. D. Köster and C. R. D. Correia, Angew. Chem., Int. Ed., 2018, 57, 12067–12070 CrossRef CAS PubMed;
(g) Z.-X. Tian, J.-B. Qiao, G.-L. Xu, X. Pang, L. Qi, W.-Y. Ma, Z.-Z. Zhao, J. Duan, Y.-F. Du, P. Su, X.-Y. Liu and X.-Z. Shu, J. Am. Chem. Soc., 2019, 141, 7637–7643 CrossRef CAS PubMed;
(h) Z.-M. Zhang, B. Xu, L. Wu, Y. Wu, Y. Qian, L. Zhou, Y. Liu and J. Zhang, Angew. Chem., Int. Ed., 2019, 58, 14653–14659 CrossRef CAS PubMed;
(i) H. Qi, D. Chi and S. Chen, Org. Lett., 2022, 24, 2910–2914 CrossRef CAS PubMed.
- S. Guo, P. Li, Z. Guan, L. Cai, S. Chen, A. Lin and H. Yao, Org. Lett., 2019, 21, 921–925 CrossRef CAS PubMed.
-
(a) Z. Zuo, J. Wang, J. Liu, Y. Wang and X. Luan, Angew. Chem., Int. Ed., 2020, 59, 653–657 CrossRef CAS PubMed;
(b) L. Fan, J. Hao, J. Yu, X. Ma, J. Liu and X. Luan, J. Am. Chem. Soc., 2020, 142, 6698–6707 CrossRef CAS PubMed;
(c) M. Pérez-Gómez, P. Herrera-Ramírez, D. Bautista, I. Saura-Llamas and J.-A. García-López, Organometallics, 2022, 41, 649–658 CrossRef PubMed;
(d) J. Wu, L. Li, M. Liu, L. Bai and X. Luan, Angew. Chem., Int. Ed., 2022, 61 DOI:10.1002/anie.202113820.
-
(a) R. K. Thalji, K. A. Ahrendt, R. G. Bergman and J. A. Ellman, J. Am. Chem. Soc., 2001, 123, 9692–9693 CrossRef CAS PubMed;
(b) Z. Ding and N. Yoshikai, Angew. Chem., Int. Ed., 2013, 52, 8574–8578 CrossRef CAS PubMed;
(c) T. A. Davis, T. K. Hyster and T. Rovis, Angew. Chem., Int. Ed., 2013, 52, 14181–14185 CrossRef CAS PubMed;
(d) B. Ye, P. A. Donets and N. Cramer, Angew. Chem., Int. Ed., 2014, 53, 507–511 CrossRef CAS PubMed;
(e) Z. Shi, M. Boultadakis-Arapinis, D. C. Koester and F. Glorius, Chem. Commun., 2014, 50, 2650–2652 RSC;
(f) T. A. Davis, C. Wang and T. Rovis, Synlett, 2015, 26, 1520–1524 CrossRef CAS PubMed;
(g) K. Ghosh, R. K. Rit, E. Ramesh and A. K. Sahoo, Angew. Chem., Int. Ed., 2016, 55, 7821–7825 CrossRef CAS PubMed;
(h) D. F. Fernández, M. Gulías, J. L. Mascareñas and F. López, Angew. Chem., Int. Ed., 2017, 56, 9541–9545 CrossRef PubMed;
(i) C. Chen, C. Shi, Y. Yang and B. Zhou, Chem. Sci., 2020, 11, 12124–12129 RSC;
(j) W. Yu, C. Chen, L. Feng, T. Xia, C. Shi, Y. Yang and B. Zhou, Org. Lett., 2022, 24, 1762–1767 CrossRef CAS PubMed.
- B. Ye and N. Cramer, J. Am. Chem. Soc., 2013, 135, 636–639 CrossRef CAS PubMed.
-
(a) Y. Park, K. T. Park, J. G. Kim and S. Chang, J. Am. Chem. Soc., 2015, 137, 4534–4542 CrossRef CAS PubMed;
(b) J. Park and S. Chang, Angew. Chem., Int. Ed., 2015, 54, 14103–14107 CrossRef CAS PubMed;
(c) H. Xiong, S. Xu, S. Sun and J. Cheng, Org. Chem. Front., 2018, 5, 2880–2884 RSC;
(d) S. Y. Hong, Y. Hwang, M. Lee and S. Chang, Acc. Chem. Res., 2021, 54, 2683–2700 CrossRef CAS PubMed.
-
(a) Y. Kuninobu, K. Kikuchi, Y. Tokunaga, Y. Nishina and K. Takai, Tetrahedron, 2008, 64, 5974–5981 CrossRef CAS;
(b) K. D. Hesp, R. G. Bergman and J. A. Ellman, J. Am. Chem. Soc., 2011, 133, 11430–11433 CrossRef CAS PubMed;
(c) K. Muralirajan, K. Parthasarathy and C.-H. Cheng, Org. Lett., 2012, 14, 4262–4265 CrossRef CAS PubMed;
(d) W. Hou, B. Zhou, Y. Yang, H. Feng and Y. Li, Org. Lett., 2013, 15, 1814–1817 CrossRef CAS PubMed;
(e) J. R. Hummel and J. A. Ellman, Org. Lett., 2015, 17, 2400–2403 CrossRef CAS PubMed.
-
(a) J. Stanslas, D. J. Hagan, M. J. Ellis, C. Turner, J. Carmichael, W. Ward, T. R. Hammonds and M. F. G. Stevens, J. Med. Chem., 2000, 43, 1563–1572 CrossRef CAS PubMed;
(b) H. Ihmels, K. Faulhaber, D. Vedaldi, F. Dall'Acqua and G. Viola, Photochem. Photobiol., 2005, 81, 1107–1115 CrossRef CAS PubMed;
(c) X. Cheng, D. Wang, L. Jiang and D. Yang, Chem. Biodiversity, 2008, 5, 1335–1344 CrossRef CAS PubMed;
(d) K. Bbadra and G. S. Kumar, Med. Res. Rev., 2011, 31, 821–862 CrossRef PubMed;
(e) K. Benner, H. Ihmels, S. Kölsh and P. M. Pithan, Org. Biomol. Chem., 2014, 12, 1725–1734 RSC;
(f) A. Granzhan, H. Ihmels and M. Tian, ARKIVOC, 2015, 494–523 Search PubMed;
(g) K. Xu, Y. Fu, Y. Zhou, F. Hennersdorf, P. Machata, I. Vincon, J. J. Weigand, A. A. Popov, R. Berger and X. Feng, Angew. Chem., Int. Ed., 2017, 56, 15876–15881 CrossRef CAS PubMed;
(h) Q. Li, Y. Li, T. Min, J. Gong, L. Du, D. L. Phillips, J. Liu, J. W. Y. Lam, H. H. Y. Sung, I. D. Williams, R. T. K. Kwok, C. L. Ho, K. Li, J. Wang and B. Z. Tang, Angew. Chem., Int. Ed., 2020, 59, 9470–9477 CrossRef CAS PubMed.
Footnote |
† Electronic supplementary information (ESI) available: Experimental procedures and characterization data of all compounds. CCDC 2143654 (32) and 2158590 (A). For ESI and crystallographic data in CIF or other electronic format see https://doi.org/10.1039/d2sc02205b |
|
This journal is © The Royal Society of Chemistry 2022 |
Click here to see how this site uses Cookies. View our privacy policy here.