DOI:
10.1039/D2SC01887J
(Edge Article)
Chem. Sci., 2022,
13, 6348-6354
Regioselective synthesis of spirocyclic pyrrolines via a palladium-catalyzed Narasaka–Heck/C–H activation/[4 + 2] annulation cascade reaction†
Received
1st April 2022
, Accepted 5th May 2022
First published on 6th May 2022
Abstract
A novel palladium-catalyzed spirocyclization through sequential Narasaka–Heck cyclization, C–H activation and [4 + 2] annulation has been developed. In this reaction, cheap and readily available 2-chlorobenzoic acid or ethyl phenylpropiolate was employed as the C2 insertion unit to react with γ,δ-unsaturated oxime ester. The key step in this transformation is the regioselective insertion of the C2 synthon into the spiro-palladacycle intermediate that is formed by the δ-C–H activation process, thereby efficiently assembling a series of spirocyclic pyrrolines with high regiocontrol. Furthermore, density functional theory (DFT) calculations and control experiments were performed to gain some insights into the reaction mechanism.
Introduction
In the past few decades, palladium-catalyzed inert C–H bond activation, as an extremely powerful tool for constructing useful compounds, including industrial materials, pharmaceuticals and natural products, has experienced tremendous developments due to its high atom- and step-economy.1–11 Many of the pioneering studies on palladium-catalyzed directing-group-assisted C–H activation have been widely investigated.12–18 Moreover, palladium-catalyzed intramolecular Heck-type cyclization/C–H activation cascade reactions, as a complementary strategy for the remote C–H bond activation, were also extensively reported.19,20 In 1994, Grigg and co-workers for the first time discovered that the in situ generated transient σ-alkyl-Pd(II) intermediate of Heck cyclization could activate the remote C–H bond to construct palladacycle, and its direct reductive elimination forms spiro-heterocycles.21,22 This efficient strategy represents an elegant means to complex carbocyclic and heterocyclic molecular frameworks in one step from well-designed starting materials.23–30 Afterward, other conversion methods of palladacycle, including [1,4]-Pd shift31–35 and coupling reagent capture,36–42 have also been developed to furnish various polycyclic compounds. However, direct reductive elimination and [1,4]-Pd shift conversions of palladacycle rely on the inherent C–H bonds as the terminal functional group, thus leading to poor product diversity. The palladacycle capture strategy has been drawing significant attention because various external reagents could be introduced by trapping palladacycle. Despite great progress, such domino reactions of the capturing strategy are usually initiated by the oxidative addition of palladium to aryl halogens in most cases; in contrast, other triggering modes remain underdeveloped. To the best of our knowledge, the regioselective insertion of unsymmetrical synthons into the palladium center is difficult to control, yielding mainly inseparable isomeric mixtures.43–50
In 1999, Narasaka and co-workers for the first time reported a palladium catalyzed cyclization of γ,δ-unsaturated oxime ester, namely the Narasaka–Heck reaction,51–53 which is an efficient method for synthesizing pyrrole. Subsequently, this kind of reaction was widely used to establish structurally diverse N-heterocyclic compounds,54–57 such as imidazoles,58 pyridines,59–61 indoles62 and isoquinolines.63,64 What is more, Bower,65–69 Zhu,70 Tong71 and other groups72–75 extensively synthesized functionalized pyrrolines by using nucleophiles to trap the σ-alkyl-Pd(II) intermediate of the Narasaka–Heck cyclization. In addition, our group reported the synthesis of polyfluorophenylated pyrrolines by using the C6F5CO2− leaving group as the C6F5− source via its decarboxylation.76 Altogether, previous reports about the Narasaka–Heck reaction mainly focused on σ-alkyl-Pd(II) intermediate nucleophile trapping and β-hydrogen elimination. Recently, our group made a significant breakthrough, synthesizing a series of highly strained spirocyclobutane-pyrrolines through a Narasaka–Heck/C–H activation cascade reaction (Scheme 1a), which is the first case of using the σ-alkyl-Pd(II) intermediate from Narasaka–Heck cyclization for intramolecular C–H activation.77 Despite numerous achievements, the ring size of spirocyclic pyrrolines is limited. Consequently, methods to obtain more diverse spirocyclic pyrrolines are highly desirable, which can be accomplished via employing external reagents to capture the palladacycle from Narasaka–Heck/C–H activation cascade.
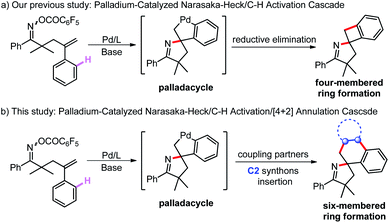 |
| Scheme 1 (a) Our previous study; (b) this study. | |
While the efficient synthesis of various spirocyclic pyrrolines through the domino Narasaka–Heck/C–H activation reaction has been reported, the ring size is limited to a four-membered ring. There is an urgent need for methods to access more diverse spirocyclic products, which are regarded as significant scaffolds in drug discovery and development.78–82 Inspired by these causes and our interest in tandem reactions involving palladacycles, we envisioned that a spiropalladacycle formed by the Narasaka–Heck/C–H activation cascade could be trapped by the C2 synthon (Scheme 1b), and its insertion leads to the formation of a six-membered ring.
Results and discussion
For the insertion of an aromatic ring, cheap and readily available 2-chlorobenzoic acid, in sharp contrast to the unselective insertion of aryne or aryl halide, inserts into palladacycle with high regioselectivity because the palladacycle reacts with 2-chlorobenzoic acids through two sequential C–C cross-couplings, and two C–C bonds are formed with excellent chemoselectivity.83–90 Consequently, we commenced our initial investigation by employing γ,δ-unsaturated oxime ester 1a and 2-chlorobenzoic acid 2a as the model substrates (Table 1). Fortunately, the anticipated domino reaction of 1a and 2a was conducted in the presence of Pd(OAc)2, P(p-Tol)3 and K2CO3 in DMF at 140 °C for 12 h under an argon atmosphere, generating the desired product 3aa in 15% yield (Table 1, entry 1). Given the previous literature reporting that n-Bu4NCl probably activate and stabilize palladium complexes,91–94 we added n-Bu4NCl (2.0 equiv.) to the reaction system and found that the extra addition of n-Bu4NCl do have a positive effect on the conversion (Table 1, entry 2). Further examination of the bases, such as Cs2CO3, Rb2CO3 and K3PO4, showed that Rb2CO3 was the most effective in terms of yield (Table 1, entries 3–5). Using other ligands, including P(o-Tol)3, P(4-OMe-Ph)3, PPh3, and P(2-furan)3, did not improve the yield (Table 1, entries 6–9). Next, after careful screening of palladium sources (Table 1, entries 10–13), Pd(MeCN)2Cl2 turned out to be the optimal choice. Gratifyingly, the yield was dramatically improved by meticulous fine-tuning of the substrate stoichiometry (Table 1, entry 14). Eventually, reducing the reaction temperature displayed better efficiency, improving the isolated yield of 3aa to 65% (Table 1, entry 15).
Table 1 Condition optimization for the reaction of γ,δ-unsaturated oxime ester and 2-chlorobenzoic acida
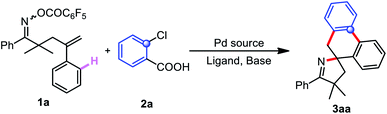
|
Entry |
Pd |
Ligand |
Base |
Yield (%) |
Reaction conditions unless otherwise noted: 1a (0.2 mmol), 2a (0.3 mmol), Pd (10 mol%), ligand (15 mol%), base (4.0 equiv.), n-Bu4NCl (2.0 equiv.), DMF (2 mL), 140 °C, 12 h, Ar; isolated yields.
No n-Bu4NCl.
1a (0.3 mmol), 2a (0.2 mmol).
130 °C.
|
1b |
Pd(OAc)2 |
P(p-Tol)3 |
K2CO3 |
15 |
2 |
Pd(OAc)2 |
P(p-Tol)3 |
K2CO3 |
31 |
3 |
Pd(OAc)2 |
P(p-Tol)3 |
Cs2CO3 |
34 |
4 |
Pd(OAc)2 |
P(p-Tol)3 |
Rb2CO3 |
40 |
5 |
Pd(OAc)2 |
P(p-Tol)3 |
K3PO4 |
7 |
6 |
Pd(OAc)2 |
P(o-Tol)3 |
Rb2CO3 |
Trace |
7 |
Pd(OAc)2 |
P(4-OMe-Ph)3 |
Rb2CO3 |
39 |
8 |
Pd(OAc)2 |
PPh3 |
Rb2CO3 |
21 |
9 |
Pd(OAc)2 |
P(2-furan)3 |
Rb2CO3 |
Trace |
10 |
Pd(PPh3)2Cl2 |
P(p-Tol)3 |
Rb2CO3 |
36 |
11 |
Pd(dba)2 |
P(p-Tol)3 |
Rb2CO3 |
34 |
12 |
Pd(MeCN)2Cl2 |
P(p-Tol)3 |
Rb2CO3 |
52 |
13 |
Pd(TFA)2 |
P(p-Tol)3 |
Rb2CO3 |
31 |
14c |
Pd(MeCN)2Cl2 |
P(p-Tol)3 |
Rb2CO3 |
64 |
15c,d |
Pd(MeCN)2Cl2 |
P(p-Tol)3 |
Rb2CO3 |
65 |
With the optimized reaction conditions in hand (Table 1, entry 15), we examined the generality of this reaction (Table 2). The regioselectivity was initially investigated by testing a variety of 2-chlorobenzoic acid derivatives. As shown in Table 2, the reactions of a series of 2-chlorobenzoic acids 2b–o with 1a yielded the desired products 3ab–3ao with complete regioselectivity. Substrates with a methyl (2b), methoxy (2c) or fluoro (2d) group at the 3-position could efficiently couple with 1a, despite steric hindrance, yielding the corresponding products (3ab–3ad) with perfect regiocontrol. Furthermore, a range of functional groups at the 4-position of 2-chlorobenzoic acid could all survive, furnishing spirocyclic products 3ae–ah. It is worth noting that the second chloro group of substrate 2i remained intact during this conversion, albeit with a decreased yield (3ai). This protocol was also applicable to 5-substituted 2-chlorobenzoic acids to afford products 3aj–al. To our delight, the reaction of the sterically hindered substrates 2m with 1a successfully gave 3am in 51% yield. Pleasingly, di- and thiophen-substituted reactants were converted into 3an and 3aovia this protocol, respectively; the spirocyclic structure of 3an was definitively elucidated by X-ray crystal structure analysis (see the ESI†). Subsequently, the scope of γ,δ-unsaturated oxime esters 1 was evaluated. The γ,δ-unsaturated oxime esters 1b–d substituted with electron-donating methyl or electron-withdrawing trifluoromethyl groups at the para- or meta-position of the aromatic ring on the oxime ester showed good reactivity in this conversion, and provided the corresponding products (3ba–3da) in 44–64% yields. As expected, disubstituted substrates 1e and 1f could also be subjected to the tandem cyclization with 2a. In addition, the reactant 1g bearing a spiro-cyclobutyl moiety also exhibited high reactivity, and 3ga was obtained in 67% yield. Similarly, substituting the aryl ring on the alkene with electron-rich or electron-deficient groups, such as methyl (1h), methoxy (1i), fluoro (1j) and trifluoromethyl (1k), delivered the desired products 3ha–3ka of the cascade reaction with 2a.
Table 2 Substrate scope for the reaction of γ,δ-unsaturated oxime esters and 2-chlorobenzoic acidsa
Reaction conditions unless otherwise noted: 1 (0.3 mmol), 2 (0.2 mmol), Pd(MeCN)2Cl2 (10 mol%), P(p-Tol)3 (15 mol%), Rb2CO3 (4.0 equiv.), n-Bu4NCl (2.0 equiv.), DMF (2 mL), 130 °C, 12 h, Ar; isolated yields.
|
|
Inspired by the above results, we next turned our attention to probing the feasibility of employing cheap and readily accessible ethyl phenylpropiolate as the C2 synthon.95,96 We began our studies by investigating the reaction of γ,δ-unsaturated oxime ester 1a and ethyl phenylpropiolate 4a. It is satisfactory that the target product 5aa was obtained in 53% yield and more than 20
:
1 ratio of regioisomers (rr) in a catalytic system consisting of Pd(PPh3)4 and Cs2CO3 in dioxane at 100 °C for 22 h (Table 3, entry 1). Further exploration of the solvent showed that toluene was the optimal choice (Table 3, entries 2–4). Next, various combinations of palladium sources and ligands were carefully screened (Table 3, entries 5–7), and Pd(PPh3)4 was identified as the most suitable catalyst. Using other bases, such as K2CO3, Na3PO4, K3PO4, NaOAc and PhCOOK, did not improve the yield (Table 3, entries 8–12). Subsequently, the structure of 5aa was definitively elucidated by X-ray crystal structure analysis (see the ESI†).
Table 3 Condition optimization for the reaction of γ,δ-unsaturated oxime ester and ethyl phenylpropiolatea
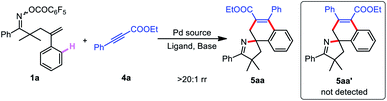
|
Entry |
Pd/ligand |
Base |
Solvent |
Yield (%) |
Reaction conditions unless otherwise noted: 1a (0.2 mmol), 4a (0.4 mmol), Pd (10 mol%), ligand (20 mol%), base (1.5 equiv.), solvent (2 mL), 100 °C, 22 h, Ar; isolated yields; rr values were determined by 1H NMR.
|
1 |
Pd(PPh3)4/— |
Cs2CO3 |
Dioxane |
53 |
2 |
Pd(PPh3)4/— |
Cs2CO3 |
MeCN |
37 |
3 |
Pd(PPh3)4/— |
Cs2CO3 |
DCE |
Trace |
4 |
Pd(PPh3)4/— |
Cs2CO3 |
Toluene |
70 |
5 |
Pd(OAc)2/PPh3 |
Cs2CO3 |
Toluene |
47 |
6 |
Pd(OAc)2/XPhos |
Cs2CO3 |
Toluene |
33 |
7 |
Pd(PPh3)4/PCy3·HBF4 |
Cs2CO3 |
Toluene |
68 |
8 |
Pd(PPh3)4/— |
K2CO3 |
Toluene |
45 |
9 |
Pd(PPh3)4/— |
Na3PO4 |
Toluene |
33 |
10 |
Pd(PPh3)4/— |
K3PO4 |
Toluene |
44 |
11 |
Pd(PPh3)4/— |
NaOAc |
Toluene |
38 |
12 |
Pd(PPh3)4/— |
PhCOOK |
Toluene |
25 |
After identifying the optimal reaction conditions, we set out to investigate the substrate scope of this cascade reaction. As shown in Table 4, both substrates 1 and 4 can be changed, thus constructing a new class of spirocyclic pyrrolines (5aa–ma). To assess the influence of 4a electronic effects on the reaction outcomes, a wide scope of functional groups, including electron-donating methyl (4b) and methoxy (4c) groups and electron-withdrawing trifluoromethyl (4d), cyano (4e), acetyl (4f), fluoro (4g), and ester (4h) groups were introduced into the aryl ring of 4a, and all were well tolerated. In addition, several disubstituted substrates (4i–k) also performed well. The pyridine-substituted product 5al was generated in 50% yield, indicating the compatibility of the heterocycle. Moreover, methyl phenylpropiolate 4m was also a viable coupling partner of 1a to provide 5am in 69% yield. When R7 was not an aromatic ring but an alkyl group, spirocyclic products 5an–ap were still successfully synthesized. Gratifyingly, substitution of the ester with ketone did not impede the generation of 5aq and 5ar. Satisfactorily, both single and double substituents of the aryl ring on oxime ester were subjected to the optimal conditions, delivering the target products 5ba–fa in 52–76% yield. Remarkably, substrate 1g with spirocyclobutane as the R3 and R4 substituents could also cyclize with 4a. Furthermore, reactants (1h–m) bearing various functional groups of the aryl ring on the alkene could participate in the spirocyclization with 4a.
Table 4 Substrate scope for the reaction of γ,δ-unsaturated oxime esters and alkynesa
Reaction conditions unless otherwise noted: 1 (0.2 mmol), 4 (0.4 mmol), Pd(PPh3)4 (10 mol%), Cs2CO3 (1.5 equiv.), toluene (2 mL), 100 °C, 22 h, Ar; isolated yields.
|
|
A series of mechanistic studies were then conducted. First, to probe the regioselective insertion of ethyl phenylpropiolate 4a into spiropalladacycle C and the potential side reaction of intermediate C, DFT calculations were performed (see the ESI† for details).97 As shown in Fig. 1, the energy barrier for the direct reductive elimination of spiropalladacycle C is much higher than that for the migratory insertion of 4a, so the direct reductive elimination of C would not occur in this reaction. In addition, the calculated energy-difference between the corresponding transition states (J-TS and J-TS′) for the two insertion patterns of ethyl phenylpropiolate favors the formation of 5aa other than 5aa′, which is responsible for the high rr value of 5aa. What is more, a series of control experiments were performed to explore whether the C–H activation process is the rate-determining step in this transformation. The intermolecular competition reaction (Scheme 2a) of equimolar 1a and 1a-D5 with 2a gave an intermolecular KIE value of 4.4, suggesting that the cleavage of the C–H bond during the CDM step of the tandem Narasaka–Heck/C–H activation/decarboxylation reaction is the rate-determining step. Similarly, the intermolecular competition experiment (Scheme 2b) of 1a, 1a-D5 and 4a also revealed that the C–H bond cleavage might be the rate-determining step for the reaction of γ,δ-unsaturated oxime esters and alkynes.
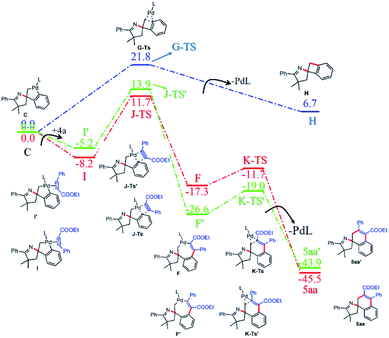 |
| Fig. 1 Free energy profile for the migratory insertion step with ethyl phenylpropiolate 4a and the direct reductive elimination of spiropalladacycle C (L = PPh3). The relative free energies are presented in kcal mol−1. | |
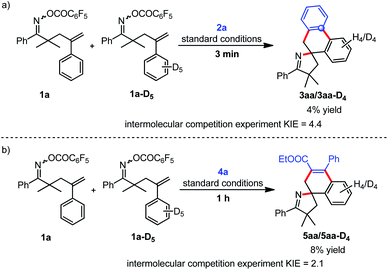 |
| Scheme 2 Control experiments, (a) kinetic isotope effect experiment of 1a and 1a-D5 with 2a; (b) kinetic isotope effect experiment of 1a and 1a-D5 with 4a. | |
On the basis of our experimental results and previous reports, a plausible catalytic cycle of this cascade reaction is illustrated in Scheme 3. Initially, oxidative addition of the Pd(0) to γ,δ-unsaturated oxime ester 1a followed by Narasaka–Heck type cyclization forms the σ-alkyl-Pd(II) intermediate B, which undergoes intramolecular C–H activation to generate spiropalladacycle(II) C. Then, when 2-chlorobenzoic acid 2a is employed as the C2 synthon, spiropalladacycle(II) C transforms into palladacycle(IV) species D by the second oxidative addition to the C–Cl bond of 2a with the assistance of an ortho-chelating carboxyl group, whose sequential reductive elimination and decarboxylation produces intermediate E, and the two sequential C–C cross-couplings are the key to the regioselective introduction of an aromatic ring. Eventually, target product 3aa and Pd(0) species are delivered by the reductive elimination of intermediate E. Alternatively, for the reaction with ethyl phenylpropiolate 4a, the regioselective migratory insertion of 4a into spiropalladacycle C forms intermediate F, and its reductive elimination generates the final product 5aa and Pd(0).
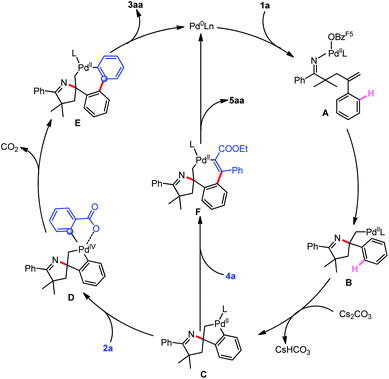 |
| Scheme 3 Proposed mechanism. | |
Conclusions
In conclusion, we developed a palladium-catalyzed Narasaka–Heck/C–H activation/[4 + 2] annulation cascade reaction, in which cheap and readily available 2-chlorobenzoic acid or ethyl phenylpropiolate is employed as the C2 insertion unit to react with γ,δ-unsaturated oxime ester. Remarkably, the highly regioselective insertion of the C2 synthon into the spiropalladacycle intermediate that is formed by δ-C–H activation is the key step in this transformation, thus providing a novel means for efficiently assembling a range of spirocyclic pyrrolines with high regiocontrol. What is more, DFT calculations revealed that the energy-difference between the corresponding transition states for the two insertion patterns of ethyl phenylpropiolate is the reason for the high rr value of 5aa. And further control experiments suggested that the cleavage of the C–H bond is the rate-determining step in this reaction.
Data availability
All data associated with this study are available in the article and ESI.†
Author contributions
W.-X. W. performed the methodology, synthesis, characterization, and analysis, and wrote the manuscript. X. K. and Y. L. carried out the calculation studies. R.-Q. J., X.-S. L., and C.-T. W. revised the manuscript. Y.-M. L. designed the project and supervised the whole experiment. All authors read and approved the final manuscript.
Conflicts of interest
The authors declare no conflicts of interest.
Acknowledgements
This work was supported by a grant from the National Natural Science Foundation of China (NSF 22171114 and 21772075).
References
- O. Baudoin, Chem. Soc. Rev., 2011, 40, 4902–4911 RSC.
- O. Baudoin, Acc. Chem. Res., 2017, 50, 1114–1123 CrossRef CAS PubMed.
- X. Chen, K. M. Engle, D.-H. Wang and J.-Q. Yu, Angew. Chem., Int. Ed., 2009, 48, 5094–5115 CrossRef CAS PubMed.
- S. H. Cho, J. Y. Kim, J. Kwak and S. Chang, Chem. Soc. Rev., 2011, 40, 5068–5083 RSC.
- Á. Gutiérrez-Bonet, F. Juliá-Hernández, B. de Luis and R. Martin, J. Am. Chem. Soc., 2016, 138, 6384–6387 CrossRef PubMed.
- Z. Huang, H. N. Lim, F. Mo, M. C. Young and G. Dong, Chem. Soc. Rev., 2015, 44, 7764–7786 RSC.
- R. Jazzar, J. Hitce, A. Renaudat, J. Sofack-Kreutzer and O. Baudoin, Chem.–Eur. J., 2010, 16, 2654–2672 CrossRef CAS PubMed.
- B.-J. Li and Z.-J. Shi, Chem. Soc. Rev., 2012, 41, 5588–5598 RSC.
- B.-J. Li, S.-D. Yang and Z.-J. Shi, Synlett, 2008, 2008, 949–957 CrossRef.
- T. W. Lyons and M. S. Sanford, Chem. Rev., 2010, 110, 1147–1169 CrossRef CAS PubMed.
- S. R. Neufeldt and M. S. Sanford, Acc. Chem. Res., 2012, 45, 936–946 CrossRef CAS PubMed.
- D. Alberico, M. E. Scott and M. Lautens, Chem. Rev., 2007, 107, 174–238 CrossRef CAS PubMed.
- R. G. Bergman, Nature, 2007, 446, 391–393 CrossRef CAS PubMed.
- O. Daugulis, H.-Q. Do and D. Shabashov, Acc. Chem. Res., 2009, 42, 1074–1086 CrossRef CAS PubMed.
- K. M. Engle, T.-S. Mei, M. Wasa and J.-Q. Yu, Acc. Chem. Res., 2012, 45, 788–802 CrossRef CAS PubMed.
- C. Jia, T. Kitamura and Y. Fujiwara, Acc. Chem. Res., 2001, 34, 633–639 CrossRef CAS PubMed.
- A. E. Shilov and G. B. Shul'pin, Chem. Rev., 1997, 97, 2879–2932 CrossRef CAS PubMed.
- M. Wasa and J.-Q. Yu, J. Am. Chem. Soc., 2008, 130, 14058–14059 CrossRef CAS PubMed.
- V. P. Mehta and J.-A. García-López, ChemCatChem, 2017, 9, 1149–1156 CrossRef CAS.
- Y. Ping, Y. Li, J. Zhu and W. Kong, Angew. Chem., Int. Ed., 2019, 58, 1562–1573 CrossRef CAS PubMed.
- D. Brown, K. Grigg, V. Sridharan and V. Tambyrajah, Tetrahedron Lett., 1995, 36, 8137–8140 CrossRef CAS.
- R. Grigg, P. Fretwell, C. Meerholtz and V. Sridharan, Tetrahedron, 1994, 50, 359–370 CrossRef CAS.
- D. S. Chung, J. S. Lee, H. Ryu, J. Park, H. Kim, J. H. Lee, U. B. Kim, W. K. Lee, M.-H. Baik and S.-g. Lee, Angew. Chem., Int. Ed., 2018, 57, 15460–15464 CrossRef CAS PubMed.
- W. Du, Q. Gu, Z. Li and D. Yang, J. Am. Chem. Soc., 2015, 137, 1130–1135 CrossRef CAS PubMed.
- Q. Huang and R. C. Larock, Tetrahedron Lett., 2009, 50, 7235–7238 CrossRef CAS.
- T. Piou, L. Neuville and J. Zhu, Angew. Chem., Int. Ed., 2012, 51, 11561–11565 CrossRef CAS PubMed.
- R. T. Ruck, M. A. Huffman, M. M. Kim, M. Shevlin, W. V. Kandur and I. W. Davies, Angew. Chem., Int. Ed., 2008, 47, 4711–4714 CrossRef CAS PubMed.
- G. Satyanarayana, C. Maichle-Mössmer and M. E. Maier, Chem. Commun., 2009, 1571–1573, 10.1039/B820636H.
- F. Ye, Y. Ge, A. Spannenberg, H. Neumann and M. Beller, Nat. Commun., 2020, 11, 5383 CrossRef CAS PubMed.
- J. Ye, Z. Shi, T. Sperger, Y. Yasukawa, C. Kingston, F. Schoenebeck and M. Lautens, Nat. Chem., 2017, 9, 361–368 CrossRef CAS PubMed.
- A. Bunescu, T. Piou, Q. Wang and J. Zhu, Org. Lett., 2015, 17, 334–337 CrossRef CAS PubMed.
- T. Čarný, R. Rocaboy, A. Clemenceau and O. Baudoin, Angew. Chem., Int. Ed., 2020, 59, 18980–18984 CrossRef PubMed.
- A. Clemenceau, P. Thesmar, M. Gicquel, A. Le Flohic and O. Baudoin, J. Am. Chem. Soc., 2020, 142, 15355–15361 CrossRef CAS PubMed.
- Q. Huang, A. Fazio, G. Dai, M. A. Campo and R. C. Larock, J. Am. Chem. Soc., 2004, 126, 7460–7461 CrossRef PubMed.
- T. Piou, A. Bunescu, Q. Wang, L. Neuville and J. Zhu, Angew. Chem., Int. Ed., 2013, 52, 12385–12389 CrossRef CAS PubMed.
- A. Lu, X. Ji, B. Zhou, Z. Wu and Y. Zhang, Angew. Chem., Int. Ed., 2018, 57, 3233–3237 CrossRef CAS PubMed.
- M. Pérez-Gómez, S. Hernández-Ponte, D. Bautista and J.-A. García-López, Chem. Commun., 2017, 53, 2842–2845 RSC.
- C. Shao, Z. Wu, X. Ji, B. Zhou and Y. Zhang, Chem. Commun., 2017, 53, 10429–10432 RSC.
- B. Tan, L. Bai, P. Ding, J. Liu, Y. Wang and X. Luan, Angew. Chem., Int. Ed., 2019, 58, 1474–1478 CrossRef CAS PubMed.
- Z. Wu, D. Ma, B. Zhou, X. Ji, X. Ma, X. Wang and Y. Zhang, Angew. Chem., Int. Ed., 2017, 56, 12288–12291 CrossRef CAS PubMed.
- H. Zheng, Y. Zhu and Y. Shi, Angew. Chem., Int. Ed., 2014, 53, 11280–11284 CrossRef CAS PubMed.
- Z. Zuo, J. Wang, J. Liu, Y. Wang and X. Luan, Angew. Chem., Int. Ed., 2020, 59, 653–657 CrossRef CAS PubMed.
- X. Luo, Y. Xu, G. Xiao, W. Liu, C. Qian, G. Deng, J. Song, Y. Liang and C. Yang, Org. Lett., 2018, 20, 2997–3000 CrossRef CAS PubMed.
- M. Pérez-Gómez and J.-A. García-López, Angew. Chem., Int. Ed., 2016, 55, 14389–14393 CrossRef PubMed.
- M. Pérez-Gómez, L. Navarro, I. Saura-Llamas, D. Bautista, M. Lautens and J.-A. García-López, Organometallics, 2017, 36, 4465–4476 CrossRef.
- M. Sickert, H. Weinstabl, B. Peters, X. Hou and M. Lautens, Angew. Chem., Int. Ed., 2014, 53, 5147–5151 CrossRef CAS PubMed.
- D. Wei, M. Y. Li, B. B. Zhu, X. D. Yang, F. Zhang, C. G. Feng and G. Q. Lin, Angew. Chem., Int. Ed., 2019, 58, 16543–16547 CrossRef CAS PubMed.
- T. Yao and D. He, Org. Lett., 2017, 19, 842–845 CrossRef CAS PubMed.
- H. Yoon, A. Lossouarn, F. Landau and M. Lautens, Org. Lett., 2016, 18, 6324–6327 CrossRef CAS PubMed.
- Z. Zuo, H. Wang, Y. Diao, Y. Ge, J. Liu and X. Luan, ACS Catal., 2018, 8, 11029–11034 CrossRef CAS.
- K. Narasaka and M. Kitamura, Eur. J. Org. Chem., 2005, 2005, 4505–4519 CrossRef.
- H. Tsutsui, M. Kitamura and K. Narasaka, Bull. Chem. Soc. Jpn., 2002, 75, 1451–1460 CrossRef CAS.
- H. Tsutsui and K. Narasaka, Chem. Lett., 1999, 28, 45–46 CrossRef.
- S. Chiba, M. Kitamura, O. Saku and K. Narasaka, Bull. Chem. Soc. Jpn., 2004, 77, 785–796 CrossRef CAS.
- M. Kitamura, S. Chiba, O. Saku and K. Narasaka, Chem. Lett., 2002, 31, 606–607 CrossRef.
- M. Kitamura and K. Narasaka, Chem. Rec., 2002, 2, 268–277 CrossRef CAS PubMed.
- K. Okamoto, T. Oda, S. Kohigashi and K. Ohe, Angew. Chem., Int. Ed., 2011, 50, 11470–11473 CrossRef CAS PubMed.
- S. Zaman, K. Mitsuru and A. D. Abell, Org. Lett., 2005, 7, 609–611 CrossRef CAS PubMed.
- S. Liu and L. S. Liebeskind, J. Am. Chem. Soc., 2008, 130, 6918–6919 CrossRef CAS PubMed.
- Z.-H. Ren, Z.-Y. Zhang, B.-Q. Yang, Y.-Y. Wang and Z.-H. Guan, Org. Lett., 2011, 13, 5394–5397 CrossRef CAS PubMed.
- H. Tsutsui and K. Narasaka, Chem. Lett., 2001, 30, 526–527 CrossRef.
- Y. Tan and J. F. Hartwig, J. Am. Chem. Soc., 2010, 132, 3676–3677 CrossRef CAS PubMed.
- T. Gerfaud, L. Neuville and J. Zhu, Angew. Chem., Int. Ed., 2009, 48, 572–577 CrossRef CAS PubMed.
- P. C. Too, Y.-F. Wang and S. Chiba, Org. Lett., 2010, 12, 5688–5691 CrossRef CAS PubMed.
- A. Faulkner and J. F. Bower, Angew. Chem., Int. Ed., 2012, 51, 1675–1679 CrossRef CAS PubMed.
- A. Faulkner, J. S. Scott and J. F. Bower, J. Am. Chem. Soc., 2015, 137, 7224–7230 CrossRef CAS PubMed.
- I. R. Hazelden, R. C. Carmona, T. Langer, P. G. Pringle and J. F. Bower, Angew. Chem., Int. Ed., 2018, 57, 5124–5128 CrossRef CAS PubMed.
- N. J. Race and J. F. Bower, Org. Lett., 2013, 15, 4616–4619 CrossRef CAS PubMed.
- N. J. Race, A. Faulkner, G. Fumagalli, T. Yamauchi, J. S. Scott, M. Rydén-Landergren, H. A. Sparkes and J. F. Bower, Chem. Sci., 2017, 8, 1981–1985 RSC.
- X. Bao, Q. Wang and J. Zhu, Angew. Chem., Int. Ed., 2017, 56, 9577–9581 CrossRef CAS PubMed.
- C. Chen, L. Hou, M. Cheng, J. Su and X. Tong, Angew. Chem., Int. Ed., 2015, 54, 3092–3096 CrossRef CAS PubMed.
- C. Chen, Y. Bao, J. Zhao and B. Zhu, Chem.
Commun., 2019, 55, 14697–14700 RSC.
- L. Feng, L. Guo, C. Yang, J. Zhou and W. Xia, Org. Lett., 2020, 22, 3964–3968 CrossRef CAS PubMed.
- L. Wang and C. Wang, Org. Chem. Front., 2018, 5, 3476–3482 RSC.
- Y. Zhang, H.-J. Ai and X.-F. Wu, Org. Chem. Front., 2020, 7, 2986–2990 RSC.
- W.-X. Wei, S. Chen, Y. Xia, M. Li, X.-S. Li, Y.-P. Han, C.-T. Wang and Y.-M. Liang, ChemCatChem, 2019, 11, 5754–5757 CrossRef CAS.
- W.-X. Wei, Y. Li, Y.-T. Wen, M. Li, X.-S. Li, C.-T. Wang, H.-C. Liu, Y. Xia, B.-S. Zhang, R.-Q. Jiao and Y.-M. Liang, J. Am. Chem. Soc., 2021, 143, 7868–7875 CrossRef CAS PubMed.
- L. Bondada, R. Rondla, U. Pradere, P. Liu, C. Li, D. Bobeck, T. McBrayer, P. Tharnish, J. Courcambeck, P. Halfon, T. Whitaker, F. Amblard, S. J. Coats and R. F. Schinazi, Bioorg. Med. Chem. Lett., 2013, 23, 6325–6330 CrossRef CAS PubMed.
- D. G. Brown, P. R. Bernstein, A. Griffin, S. Wesolowski, D. Labrecque, M. C. Tremblay, M. Sylvester, R. Mauger, P. D. Edwards, S. R. Throner, J. J. Folmer, J. Cacciola, C. Scott, L. A. Lazor, M. Pourashraf, V. Santhakumar, W. M. Potts, S. Sydserff, P. Giguère, C. Lévesque, M. Dasser and T. Groblewski, J. Med. Chem., 2014, 57, 733–758 CrossRef CAS PubMed.
- Y. Deng, Z. Yang, G. W. Shipps, S.-M. Lo, R. West, J. Hwa, S. Zheng, C. Farley, J. Lachowicz, M. van Heek, A. S. Bass, D. P. Sinha, C. R. Mahon and M. E. Cartwright, Bioorg. Med. Chem. Lett., 2013, 23, 791–796 CrossRef CAS PubMed.
- D. A. Griffith, R. L. Dow, K. Huard, D. J. Edmonds, S. W. Bagley, J. Polivkova, D. Zeng, C. N. Garcia-Irizarry, J. A. Southers, W. Esler, P. Amor, K. Loomis, K. McPherson, K. B. Bahnck, C. Préville, T. Banks, D. E. Moore, A. M. Mathiowetz, E. Menhaji-Klotz, A. C. Smith, S. D. Doran, D. A. Beebe and M. F. Dunn, J. Med. Chem., 2013, 56, 7110–7119 CrossRef CAS PubMed.
- J. O. Link, J. G. Taylor, L. Xu, M. Mitchell, H. Guo, H. Liu, D. Kato, T. Kirschberg, J. Sun, N. Squires, J. Parrish, T. Keller, Z.-Y. Yang, C. Yang, M. Matles, Y. Wang, K. Wang, G. Cheng, Y. Tian, E. Mogalian, E. Mondou, M. Cornpropst, J. Perry and M. C. Desai, J. Med. Chem., 2014, 57, 2033–2046 CrossRef CAS PubMed.
- W. C. Fu, Z. Wang, W. T. K. Chan, Z. Lin and F. Y. Kwong, Angew. Chem., Int. Ed., 2017, 56, 7166–7170 CrossRef CAS PubMed.
- Y. Gu, X. Sun, B. Wan, Z. Lu and Y. Zhang, Chem. Commun., 2020, 56, 10942–10945 RSC.
- X. Luo, L. Zhou, H. Lu, G. Deng, Y. Liang, C. Yang and Y. Yang, Org. Lett., 2019, 21, 9960–9964 CrossRef CAS PubMed.
- X. Yang, X. Chen, Y. Xu, M. Zhang, G. Deng, Y. Yang and Y. Liang, Org. Lett., 2021, 23, 2610–2615 CrossRef PubMed.
- Y. Yang, B. Zhou, X. Zhu, G. Deng, Y. Liang and Y. Yang, Org. Lett., 2018, 20, 5402–5405 CrossRef CAS PubMed.
- M. Zhang, W. Deng, M. Sun, L. Zhou, G. Deng, Y. Liang and Y. Yang, Org. Lett., 2021, 23, 5744–5749 CrossRef CAS PubMed.
- Q. Zhao, W. C. Fu and F. Y. Kwong, Angew. Chem., Int. Ed., 2018, 57, 3381–3385 CrossRef CAS PubMed.
- L. Zhou, M. Sun, F. Zhou, G. Deng, Y. Yang and Y. Liang, Org. Lett., 2021, 23, 7150–7155 CrossRef CAS PubMed.
- C. Amatore, M. Azzabi and A. Jutand, J. Am. Chem. Soc., 1991, 113, 8375–8384 CrossRef CAS.
- C. Amatore and A. Jutand, Acc. Chem. Res., 2000, 33, 314–321 CrossRef CAS PubMed.
- M. Piber, A. E. Jensen, M. Rottländer and P. Knochel, Org. Lett., 1999, 1, 1323–1326 CrossRef CAS.
- X. Yang, H. Lu, X. Zhu, L. Zhou, G. Deng, Y. Yang and Y. Liang, Org. Lett., 2019, 21, 7284–7288 CrossRef CAS PubMed.
- J. F. Rodríguez, A. D. Marchese and M. Lautens, Org. Lett., 2018, 20, 4367–4370 CrossRef PubMed.
- H. Yoon, M. Rölz, F. Landau and M. Lautens, Angew. Chem., Int. Ed., 2017, 56, 10920–10923 CrossRef CAS PubMed.
- I. Franzoni, H. Yoon, J.-A. García-López, A. I. Poblador-Bahamonde and M. Lautens, Chem. Sci., 2018, 9, 1496–1509 RSC.
|
This journal is © The Royal Society of Chemistry 2022 |