DOI:
10.1039/D2SC00526C
(Edge Article)
Chem. Sci., 2022,
13, 5693-5700
Designable assembly of atomically precise Al4O4 cubane supported mesoporous heterometallic architectures†
Received
27th January 2022
, Accepted 1st April 2022
First published on 19th April 2022
Abstract
Heterometallic cluster-based framework materials are of interest in terms of both their porous structures and multi-metallic reactivity. However, such materials have not yet been extensively investigated because of difficulties in their synthesis and structural characterization. Herein, we reported the designable synthesis of atomically precise heterometallic cluster-based framework compounds and their application as catalysts in aldol reactions. By using the synergistic coordination protocol, we successfully isolated a broad range of compounds with the general formula, [Al4M4O4(L)12(DABCO)2] (L = carboxylates; DABCO = 1,4-diazabicyclo[2.2.2]-octane; M2+ = Co2+, Mn2+, Zn2+, Fe2+, Cd2+). The basic heterometallic building blocks contain unprecedented main-group γ-alumina moieties and surrounding unsaturated transition metal centers. Interestingly, the porosity and interpenetration of these frameworks can be rationally regulated through the unprecedented strategy of increment of the metal radius in addition to general introduction of sterically bulky groups on the ligand. Furthermore, these porous materials are effective catalysts for aldol reactions. This work provides a catalytic molecular model platform with accurate molecular bonding between the supporters and catalytically active metal ions.
Introduction
Aluminosilicate zeolites with 4-connected metal centers are a class of inorganic porous solids with a range of applications in catalysis, ion exchange and separation.1–6 Compared with these traditional 4-connected nodes,7–18 the use of polynuclear metal clusters as 4-connected nodes is highly desirable because of the availability of large pore sizes and the possibility of combining unique cluster-based properties.19–22 Such an idea has been realized by Feng et al.22,23via the assembly of supertetrahedral metal–chalcogenide clusters. Most significantly, elements without tetrahedral geometry can be incorporated, thus providing access to multifunctional framework materials with zeolite type topology.24–26
Heterometallic oxo clusters may exhibit unique properties resulting from the synergy effect of the two substantially different types of metal ions. Therefore, it is of great interest to incorporate heterometallic clusters as 4-connected centers. However, extensive studies based on 4-connected heterometallic clusters remain scarce because of the lack of a strategy for efficient synthesis. There are two possible synthetic challenges: (1) competitive coordination and integration of two kinds of metal ions, and (2) metal clusters with high nuclearity and positive charge are prone to accepting more organic ligands, thus leading to highly-connected dense topologies. Once the building blocks are established, effective interpenetration regulation is another issue that should be considered.27,28 In 2019, Zheng et al.29 summarized advances in the design and synthesis of cluster organic frameworks based on 4-connected metal–halide clusters,30 metal–oxygen clusters,31 and metal–chalcogen clusters.32 In the domain of metal–oxygen clusters, only transition metal clusters (such as ε-Zn4PMo12
26 or Cd4Cu6
33) have been reported as 4-connected nodes. However, main group element-based examples are challenging and still lacking.
γ-Al2O3 is one of the most common kinds of alumina, which has a large surface area, and strong adsorption capacity and has been widely used in adsorbents, catalysts and catalyst supports.34 With our recent research in Al(III) chemistry, we herein demonstrate a synergistic coordination strategy towards the assembly of 4-connected heterometallic cluster-based framework materials. The strategy is based on spontaneous selective coordination between two kinds of metal centers (Al centers and d-transition metals) and two types of ligands (chelating L with O-donors and bridging DABCO with N donors, respectively) (Scheme 1a).35 Such an approach is universal and we successfully isolate a broad range of compounds [Al4O4M4(L)12(DABCO)2] (denoted as AlOCs; M2+ = Mn2+, Fe2+, Co2+, Zn2+, Cd2+, L = carboxylates; DABCO = 1,4-diazabicyclo[2.2.2]octane) (Table 1). We were surprised to find that the Al4O4 cubane (denoted as the γ-alumina moiety) is located in the center of the 4-connected nodes (Scheme 1b).36,37 In addition, both the inorganic and organic shells of the Al4O4 cubane can be modified to a large extent. Interestingly, controllable interpenetration is realized through the introduction of sterically bulky groups, increment of the ionic radius of transition metal ions, and variation of the M2+/Al3+ concentration. Furthermore, these porous materials are effective catalysts for aldol reactions.
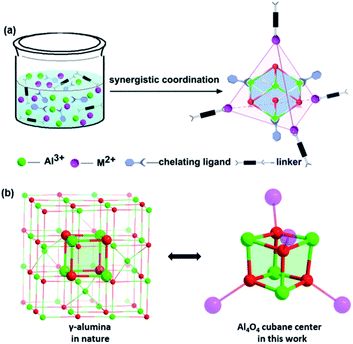 |
| Scheme 1 (a) Scheme demonstrating the synergistic coordination synthetic strategy; (b) comparison between the structures of γ-Al2O3 and the Al4O4 cubane in a tetrahedral cluster node. | |
Table 1 A summary of the 4-connected AlOCsa
Complex |
Composition |
Sp. Gr. |
R
1 Value |
Void ratio |
Abbreviations: BA = benzoate; 2-FBA = 2-fluorobenzoate; 3-FBA = 3-fluorobenzoate; 4-FBA = 4-fluorobenzoate; 2-FA = 2-furan formate; 2-TA = 2-thiophene formate; 3-TA = 3-thiophene formate; 3-MBA = 3-methylbenzoate; 4-MBA = 4-methylbenzoate; 4-CBA = 4-chlorobenzoate; 4-ABA = 4-aminobenzoate.
|
Two-fold diamond (dia) interpenetration
|
AlOC-99
|
Al4Co4O4(BA)12(DABCO)2 |
Fd c |
0.0801 |
1.2% |
AlOC-100
|
Al4Co4O4(2-FBA)12(DABCO)2 |
Fd c |
0.0759 |
No |
AlOC-101
|
Al4Co4O4(3-FBA)12(DABCO)2 |
Fd c |
0.1149 |
7.6% |
AlOC-102
|
Al4Co4O4(4-FBA)12(DABCO)2 |
Fd c |
0.1474 |
2.5% |
AlOC-103
|
Al4Co4O4(2-FA)12(DABCO)2 |
Fd c |
0.0998 |
2.8% |
AlOC-104
|
Al4Co4O4(2-TA)12(DABCO)2 |
Fd c |
0.1447 |
1.6% |
AlOC-105
|
Al4Co4O4(3-TA)12(DABCO)2 |
Fd c |
0.1274 |
2.4% |
AlOC-106
|
Al4Mn4O4(BA)12(DABCO)2 |
Fd c |
0.0858 |
No |
AlOC-107
|
Al4Fe4O4(BA)12(DABCO)2 |
Fd c |
0.0566 |
1.0% |
AlOC-108
|
Al4Zn4O4(BA)12(DABCO)2 |
Fd c |
0.0548 |
1.0% |
![[thin space (1/6-em)]](https://www.rsc.org/images/entities/char_2009.gif) |
Non-interpenetrated diamond (dia) topology
|
AlOC-109
|
Al4Co4O4(3-MBA)12(DABCO)2 |
F4132 |
0.0736 |
42.5% |
AlOC-110
|
Al4Fe4O4(BA)12(DABCO)2 |
F4132 |
0.0634 |
52.8% |
AlOC-111
|
Al4Cd4O4(BA)12(DABCO)2 |
F4132 |
0.0751 |
55.6% |
![[thin space (1/6-em)]](https://www.rsc.org/images/entities/char_2009.gif) |
Non-interpenetrated lonsdaleite (lon) topology
|
AlOC-112
|
Al4Co4O4(BA)12(DABCO)2 |
P 2c |
0.0629 |
53.9% |
AlOC-113
|
Al4Co4O4(3-FBA)12(DABCO)2 |
P 2c |
0.0809 |
47.0% |
AlOC-114
|
Al4Co4O4(4-CBA)12(DABCO)2 |
P 2c |
0.0757 |
44.9% |
AlOC-115
|
Al4Co4O4(4-MBA)12(DABCO)2 |
P 2c |
0.0736 |
44.3% |
AlOC-116
|
Al4Co4O4(4-ABA)12(DABCO)2 |
P 2c |
0.0972 |
48.4% |
AlOC-117
|
Al4Mn4O4(BA)12(DABCO)2 |
P 2c |
0.0576 |
54.3% |
AlOC-118
|
Al4Fe4O4(BA)12(DABCO)2 |
P 2c |
0.1081 |
53.3% |
AlOC-119
|
Al4Zn4O4(BA)12(DABCO)2 |
P 2c |
0.0542 |
53.5% |
AlOC-120
|
Al4Cd4O4(BA)12(DABCO)2 |
P 2c |
0.0784 |
56.4% |
Experimental
Materials and general methods
All the reagents and solvents employed were commercially available and used without further purification. Al(OiPr)3 was acquired from Aladdin Chemical Reagent Shanghai. Fe(Ac)2 was obtained from TCI. Acetonitrile (99%), benzoic acid, Co(Ac)2·4H2O, MnCl2·4H2O, Zn(Ac)2·2H2O and Cd(Ac)2·2H2O were bought from Sinopharm Chemical Reagent Beijing. Ti(OiPr)4 and other carboxylic acids were purchased from Adamas-beta.
The energy dispersive spectroscopy (EDS) analyses of single crystals were performed on a JEOL JSM6700F field-emission scanning electron microscope equipped with an Oxford INCA system. IR spectra (KBr pellets) were recorded on an ABB Bomem MB102 spectrometer over a range of 400–3900 cm−1. Powder X-ray diffraction (PXRD) data were collected on a Rigaku Mini Flex II diffractometer using CuKα radiation (λ = 1.54056 Å) under ambient conditions. The UV-vis diffuse reflection data were recorded at room temperature using a powder sample with BaSO4 as a standard (100% reflectance) on a PerkinElmer Lamda-950 UV spectrophotometer and scanned at 200–1200 nm. The absorption data were calculated from the Kubelka–Munk function, (F(R) = (1 − R)2/2R), where R represents the reflectance.38 Surface chemical analyses were performed by X-ray photoelectron spectroscopy (XPS, Thermo Fisher, ESCALAB 250Xi). Metal proportional analyses were performed on an Ultima-2 inductively coupled plasma (ICP) spectrometer. The TGA curves were recorded in the region of 30–800 °C at a heating rate of 10 °C min−1 in a flowing N2 atmosphere on a Mettler Toledo TGA/SDTA 851e analyzer.
Synthesis of two-fold interpenetrated diamond (dia) frameworks AlOC-99–AlOC-108
Al(OiPr)3 (0.918 g, 4.5 mmol), Co(Ac)2·4H2O (0.75–2.5 g, 3–10 mmol), DABCO (0.224 g, 1 mmol) and benzoic acid (0.978 g, 8 mmol) were dissolved in acetonitrile (10 mL), and then Ti(OiPr)4 (0.5 mL, 1.5 mmol) was added to the bottle and mixed at room temperature. The resultant solution was sealed in a Teflon-lined autoclave (23 mL) and heated at 160 °C for three days. After being cooled to room temperature, red octahedral crystals of AlOC-99 were obtained (maximum yield: ∼64% (1.5 g) for AlOC-99 based on Al(OiPr)3. Synthesis of AlOC-100–AlOC-105 is similar to that of AlOC-99 except that benzoic acid was replaced by 2-FBA, 3-FBA, 4-FBA, 2-FA, 2-TA, and 3-TA. Red octahedral crystals were collected after about three days (maximum yield: ∼10% (260 mg), ∼7% (180 mg), ∼11% (290 mg), ∼1.5% (30 mg), ∼1.6% (40 mg), and ∼1.2% (30 mg) based on Al(OiPr)3 for AlOC-100–AlOC-105, respectively). Synthesis of AlOC-106–AlOC-108 is similar to that of AlOC-99 except that Co(Ac)2·4H2O was replaced by MnCl2·4H2O, Fe(Ac)2 and Cd(Ac)2·2H2O. Yellow or colorless octahedral crystals were collected after about three days (maximum yield: ∼19% (450 mg), ∼1.9% (50 mg), and ∼4.6% (110 mg) for AlOC-106–AlOC-108.
Synthesis of non-interpenetrated diamond (dia) frameworks AlOC-109–AlOC-111
Synthesis of AlOC-109–AlOC-111 is similar to that of AlOC-99 except that benzoic acid was replaced by 3-methylbenzoic acid or Co(Ac)2·4H2O was replaced by Fe(Ac)2 and Cd(Ac)2·2H2O. Red (AlOC-109), yellow (AlOC-110) or colorless (AlOC-111) octahedral crystals were collected after about three days (maximum yield: ∼20.5% (520 mg), ∼3.4% (80 mg) and ∼1.16% (30 mg) for AlOC-109–AlOC-111 based on Al(OiPr)3).
Synthesis of non-interpenetrated lonsdaleite (lon) frameworks AlOC-112–AlOC-120
The synthetic procedure of AlOC-112 is similar to that of AlOC-99, except that the amount of Co(Ac)2·4H2O was reduced to 1–2 mmol. Finally, red hexagonal flaky crystals of AlOC-112 were obtained (maximum yield: ∼34% (800 mg) for AlOC-112 based on Al(OiPr)3). Synthesis of AlOC-113–AlOC-116 is similar to that of AlOC-112 except that benzoic acid was replaced by 3-FBA, 4-CBA, 4-MBA and 4-ABA. Red hexagonal flaky crystals were collected after about three days (maximum yield: ∼23% (600 mg), ∼18% (500 mg), ∼32% (800 mg), and ∼8.2% (210 mg) for AlOC-113–AlOC-116 based on Al(OiPr)3). Synthesis of AlOC-117–AlOC-120 is similar to that of AlOC-112 except that Co(Ac)2·4H2O was replaced by MnCl2·4H2O, Fe(Ac)2, Zn(Ac)2·2H2O and Cd(Ac)2·2H2O. Yellow or colorless hexagonal flaky crystals were collected after about three days (maximum yield: ∼51.5% (1.2 g), ∼1.7% (40 mg), ∼12.6% (300 mg) and ∼2.2% (60 mg) for AlOC-117–AlOC-120 based on Al(OiPr)3).
Caution: The synthesis of AlOC-107 should be performed in a glovebox in a vacuum environment and nitrogen should be pumped into the reactor for removing the soluble oxygen from the solvent. Yellow crystals of freshly prepared Fe-containing compounds (AlOC-107, AlOC-110 and AlOC-118) can gradually turn red due to the rapid oxidation when exposed to air.
General methods for X-ray crystallography
Crystallographic data of AlOC-106 and AlOC-119 were collected on a Mercury single crystal diffractometer equipped with graphite-monochromatic Mo Kα radiation (λ = 0.71073 Å). Compounds AlOC-117 and AlOC-118 were collected on a Supernova single crystal diffractometer equipped with graphite-monochromated Cu Kα radiation (λ = 1.54184 Å). Other compounds (AlOC-99–AlOC-105, AlOC-107–AlOC-116, and AlOC-120) were collected on a Hybrid Pixel Array detector equipped with graphite-monochromated Ga Kα radiation (λ = 1.3405 Å). The structures were solved with direct methods using OLEX2 and refined by full-matrix least-squares on F2 using SHELXTL.39 Contributions to scattering due to disordered solvent molecules were removed using the SQUEEZE routine of PLATON. All hydrogen atoms were theoretically positioned, riding on the concerned atoms and refined with fixed thermal factors. Non-hydrogen atoms were refined anisotropically.
Preparation of active samples for gas adsorption
Crystals were soaked in ethanol and heated at 80 °C for one week. During this time, the ethanol solution should be exchanged several times a day. Subsequently, the sample was degassed under a dynamic vacuum at 100 °C for 6 h to activate the sample. The gas adsorption isotherms of active samples were obtained on a Micromeritics ASAP 2020 volumetric adsorption instrument.
Results and discussion
The tunable shell of Al4O4 supported supertetrahedral nodes
The prominent feature of these compounds is the presence of a central Al4O4 γ-alumina moiety. The accurate crystal structures of Al4N4,40 Al4P4, Al4S4
41 and Al4Se4
42 have been reported, while the Al4O4 cubane has never been reported and has only been studied computationally.43 However, the Al4O4 cubane can act as a subunit that exists in high-nuclearity AlOCs (Al30, Cu2Al30-S, and Zn2Al32).44–46 In this work, the Al4O4 cubane serves as a cornerstone in supertetrahedron nodes, where both inorganic (M2+ = Co, Mn, Zn, Fe, Cd) and organic (a broad range of chelated aromatic carboxylates) compositions are tunable (Fig. 1). And as many as 22 isostructural compounds were successfully isolated (Table 1, AlOC-99–AlOC-120, Fig. S1–S22†). Each Al atom in all compounds adopts a six-coordination geometry, bonding to three μ4-O atoms and three carboxylate oxygen atoms (OCOO) (Fig. S23†). Every external M ion resided in a trigonal bipyramid environment with three OCOO atoms, one μ4-O bridge and one N atom from DABCO. Each triangular face of the supertetrahedral core is consolidated by three bridging bidentate chelating ligands (Fig. S24 and S25†). Compared with the charged tetrahedral cages,47–49 the supertetrahedral cluster [Al4Co4O4(L)12] herein is neutral. Four DABCO linkers on the cluster node allow further connections, which is similar to the 4-connected TO4 (T = Si, Al, P, etc.) node in inorganic zeolite materials.
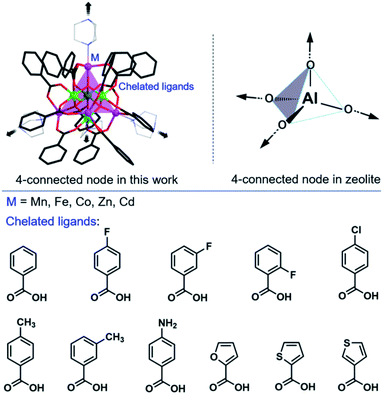 |
| Fig. 1 The tunable inorganic metal ion shell and organic chelated ligand shell on tetrahedral cluster nodes. The atom color code: O, red; C, dark grey and black; N, blue; Al, green. Hydrogen atoms are omitted for clarity. | |
Interpenetration regulation
As shown in Fig. 2, the 4-connected frameworks are categorized into three types. [Al4M4O4(L)12(DABCO)2] undergoes a change in the degree of interpenetration from a dense doubly interpenetrated framework (AlOC-99–AlOC-108, Fig. S1–S10†) to two kinds of highly porous non-interpenetrated framework with diamond (dia) topology: AlOC-109–AlOC-111, Fig. S11–S13;† and lonsdaleite (lon) topology (AlOC-112–AlOC-120, Fig. S14–S22†). It is reported that factors that affect interpenetration include pressure, temperature, solvent, ligand design, etc.27,50,51 Herein, precise suppression of interpenetration by an increment of the metal radius is found in addition to the ligand design and concentration ratio of M2+/Al3+.
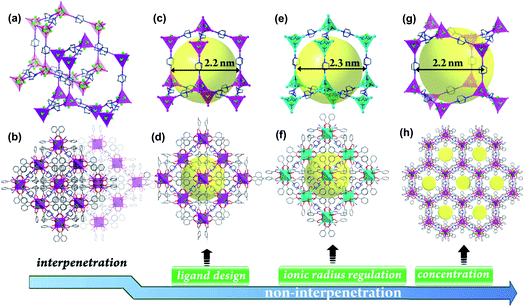 |
| Fig. 2 The interpenetration regulation strategies: introduction of sterically bulky groups, increment of the ionic radius and decrease of the M2+/Al3+ concentration. The mesoporous cavities (a, c, e, and g) and overall frameworks (b, d, f, and h) in two-fold interpenetrated AlOC-99, and non-interpenetrated AlOC-109, AlOC-111 and AlOC-112, respectively. The atom color code: O, red; C, dark grey; N, blue; Co, pink; Cd, turquoise; Al, green. Hydrogen atoms are omitted for clarity. The diamond (dia) frameworks present rhombic windows, while the lonsdaleite (lon) frameworks possess hexagonal channels (diameter: 3.761 nm; interior wall diameter: 0.909 nm) aligned parallel to the c-axis. | |
Interpenetration regulation through ligand design.
The regulation of the topology is greatly related to the types of ligands. For benzoate or fluorobenzoate, both interpenetrated and non-interpenetrated frameworks can be obtained (interpenetrated diamond (dia) topology: AlOC-99–AlOC-102; non-interpenetrated lonsdaleite (lon) topology: AlOC-112 and AlOC-113). However, if large steric groups (–Cl, –CH3 and –NH2) are introduced, only non-interpenetrated frameworks can be generated (AlOC-109 and AlOC-114–AlOC-116) (Fig. 2c, d and S11, S16–S18†). Intriguingly, the presence of the substituents can also enhance the intermolecular and intramolecular hydrogen bond interactions (Fig. S26†). For smaller pentacyclic carboxylates, only doubly interpenetrated frameworks (AlOC-103–AlOC-105) were synthesized (Fig. S5–S7†).
Interpenetration regulation through increasing the ionic radius of TM ions.
Cd-containing frameworks (AlOC-111 and AlOC-120) present non-interpenetrated topologies (Fig. 2e, f and S13, S22†), which are different from those of Mn, Fe, Co, and Zn involving compounds. A possible reason is attributed to its larger radius, and the size of Cd4Al4O4 tetrahedral clusters is obviously larger than that of other ones (Fig. S27†). Although a large number of factors influencing interpenetration have been reported, the effect of the ionic radius was discovered for the first time.
Interpenetration regulation through altering the ratio of M2+/Al3+.
The variation of the mole ratio of raw materials has a great impact on the structural types.8,50 Herein, the M2+/Al3+ concentration plays an important role in interpenetration regulation. For example, when the ratio of Co2+/Al3+ is greater than 1.11, the pure octahedral crystals with a doubly interpenetrated diamond (dia) framework can be obtained (Fig. 2a and b, AlOC-99, Fd
c; Fig. 3a, left), in which the 66 diamond (dia) cage is exclusively made up of six chair-like six-membered rings (Fig. 3b, left). When the ratio of Co2+/Al3+ is lower than 0.44, only pure hexagonal flaky crystals with non-interpenetrated lonsdaleite (lon) topologies can be generated (Fig. 2g, h and S14,†AlOC-112, P
2c; Fig. 3a, right). Different from the 66 diamond (dia) cage, the 65 lonsdaleite (lon) cage in AlOC-112 is composed of chair-like and boat-like six-membered rings (Fig. 3b, right). However, when the ratio of Co2+/Al3+ is controlled between 0.44 and 1.11, the product was a mixture phase containing both octahedral crystals and hexagonal flaky crystals (Fig. 3a, middle).
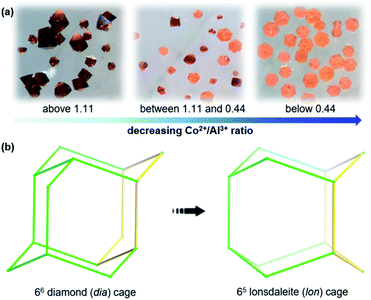 |
| Fig. 3 (a) Photos of reaction products obtained from controlling the ratio of Co2+/Al3+. (b) The simplified diamond (dia) and lonsdaleite (lon) cages. Chair-like (left) and boat-like (right) six-membered rings have been highlighted with yellow bonds. | |
Therefore, it is reasonable to infer that the low M2+/Al3+ ratio favored the non-interpenetrated lonsdaleite (lon) form,52 which is supported by the experimental results that come from the isolation of a series of isostructural Mn, Fe, Zn and Cd constructed AlOCs (AlOC-117–AlOC-120; Table 1, Fig. S19–S22†). For Fe constructed frameworks, the control of the Fe2+/Al3+ ratio is not easy due to the rapid oxidation of Fe2+. Thus, the synthesis of the doubly interpenetrated diamond (dia) framework of AlOC-107 with a high Fe2+/Al3+ ratio should be performed in a glovebox under pumped nitrogen. Instead, non-interpenetrated dia framework AlOC-110 will be generated when synthesized under the same conditions without nitrogen.
Physical performance
The non-interpenetrated framework density (for example, AlOC-112 (0.823 g cm−3)) is approximately half that of doubly interpenetrated AlOC-99 (1.651 g cm−3). The formation of a non-interpenetrated framework is noteworthy given the mesoporous cavity channels (ca. 2.2–2.3 nm) (Fig. 2c, e, g and S30†). Correspondingly, it also exhibits obvious higher solvent-accessible volumes (e.g., 53.9% for AlOC-112 calculated using PLATON).53 Considering their potential application in gas adsorption and separation, N2 sorption tests were conducted to evaluate porosity. Based on N2 sorption isotherms, the BET surface areas were calculated to be 11.48, 779.6 and 797.2 m2 g−1 for AlOC-99, AlOC-109 and AlOC-112 (Fig. 4a and S31†). The pronounced H4 hysteresis loops of AlOC-109 and AlOC-112 are located at high P/P0 (P/P0 = 0.4–1.0), further confirming the presence of the mesopores in the materials.54,55 The experimental CO2 uptake of AlOC-99, AlOC-109 and AlOC-112 was measured to be 10.2, 13.9 and 21.7 cm3 g−1 at 273 K.
The stability of frameworks plays an important role in research and industrial production, because it provides an applicable scope of the material. Their stability is related to interpenetration and topology. For example, the doubly interpenetrated framework is thermodynamically stable when heated to 300 °C, while the non-interpenetrated ones can only tolerate 250 °C (Fig. S32 and S33†), indicating that interpenetration in AlOC-99 obviously enhances the stability of the framework. In addition, AlOC-99 and AlOC-109 were stable after being immersed in an aqueous solution with different pH values (Fig. S34 and S35†). The lonsdaleite (lon) framework of AlOC-112 will collapse gradually in water, indicating that the diamond (dia) type framework is more stable than that of lonsdaleite (lon). Nevertheless, both of them were stable in common organic solvents, including MeOH, EtOH, MeCN, DMF and DMSO (Fig. S36†). Their solution stabilities are related to hydrophobic wettability. And the measured contact angles for AlOC-99, AlOC-109 and AlOC-112 are 160.58°, 140.13° and 153.23°, respectively (Fig. S37 and S38†).56 Furthermore, all three materials can remain in the air for more than one year (Fig. S39†), which is related to the presence of the Al4O4 core because compounds like Co2Bdc2Dabco without aluminium (Fig. S40†) cannot be exposed to air for more than two weeks.57,58
These compounds were all characterized by powder X-ray diffraction (PXRD), IR spectroscopy, and energy dispersive spectroscopy (EDS) analyses (Fig. S41–S68†). The atomic ratio of M to Al was determined to be approximately 1
:
1 by inductively coupled plasma (ICP) analysis, which is in agreement with the crystallographic results. The valence states of the metal ions were confirmed by bond-valence-sum (BVS) calculations and X-ray photoelectron spectroscopy (XPS) (Table S5, Fig. S69–S90†). Intriguingly, these compounds present a variety of colors (Fig. 4b), that are affected by several factors. For example, Fe-containing compounds are easily oxidized, and their colors can be changed from yellow to red when exposed to air (Fig. S91†). The colors of Co constructed frameworks are related to the framework density and chelated ligands. Doubly interpenetrated AlOC-99 presents darker color when compared with non-interpenetrated AlOC-112. Furthermore, the colors of 4-FBA coordinated frameworks (e.g., AlOC-102) are also different from that of BA modified structures due to the electron-withdrawing effects of F centers (e.g., AlOC-99). Diffuse reflectance spectroscopy measurements were performed on all complexes to better understand their optical absorption properties (Fig. S92–S95†).
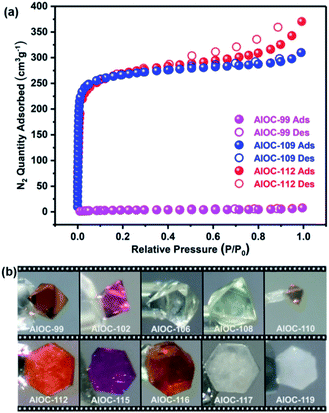 |
| Fig. 4 (a) The N2 sorption isotherms of AlOC-99, AlOC-109 and AlOC-112. (b) Crystal photos of representative samples. | |
Catalytic performance
The aldol reaction is a basic transformation in creating new carbon–carbon bonds and β-hydroxyl carbonyl compounds.59 Considering the higher stability and catalytically active sites (unsaturated transition metals) on the Al4O4 support, three representative AlOCs (AlOC-99, AlOC-109 and AlOC-112) were used as catalysts for the direct aldol reaction of acetone and nitro-substituted aromatic aldehyde in DMSO. The catalytic results are summarized in Table S6.†
In the presence of 10 mmol% catalysts, all three AlOCs show high catalytic activities giving desired aldol products in 79–88% yield. Upon completion of the reaction, all of them could be readily recovered by centrifugation and reused for the next cycle without significant loss of activity. The yield after three consecutive runs of AlOC-109 can still remain 88% for isolated products. PXRD exhibited that the recycled sample retained high crystallinity after catalysis (Fig. S96†). In order to explore the impact of penetration on performance, the amount of catalyst is reduced to 5 mmol%, and mesoporous frameworks afford higher yield (67% for AlOC-109, and 68% for AlOC-112) compared with doubly interpenetrated AlOC-99 (48%). This suggests that the catalytic reactions with a low content catalyst are related to the specific surface area. Besides, the control experiment without catalyst loading was also performed, and no condensation product can be observed, indicating that these crystals possess catalytic capability.
The probable catalytic mechanism is shown in Fig. 5.60 First, the Co centers with unsaturated coordination sites polarize the carbonyl group of acetone, leading to the dissociation of the α-proton and generating a metal enolate. The oxygen atoms on BAs bond to the α-proton and leave the Co center transitorily. Then, 4-nitrobenzaldehyde combined with the metal enolate through C–C coupling, and condensation products can be obtained.
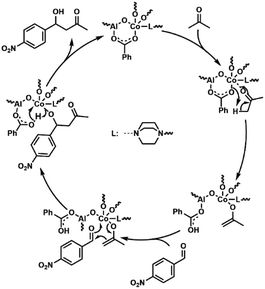 |
| Fig. 5 Probable catalytic mechanism for the aldol condensation reaction by using AlOC-99, AlOC-109 and AlOC-112 as catalysts. | |
Conclusions
This is not only the first time that the γ-alumina moiety is found in crystalline porous compounds but it also serves as a support for loading various metal ions. The incorporation of heterometallic ions broadens the scope and potential applications of framework materials. This work provides a typical catalytic molecular model and demonstrates the unambiguous connections between the support (γ-alumina moiety) and catalytically active metal ions at the atomic level. Such γ-alumina moiety-supported materials can provide a reference for the catalytic system to achieve the hetero-disperse requirement meanwhile exposing as many catalytic sites as possible.
Data availability
All data supporting this study are available from article and ESI.† The raw data files are available from author upon reasonable request.
Author contributions
All authors contributed extensively to the work presented in this paper. J. Zhang and W.-H. Fang conceived the research project. Y.-J. Liu performed the synthesis and characterization experiments. Y. Yu performed catalytic experiments. Y.-F. Sun assisted in the data collection. W.-H. Fang and Y.-J. Liu wrote the manuscript and the ESI with input from the other authors.
Conflicts of interest
There are no conflicts to declare.
Acknowledgements
This work was supported by the National Key Research & Development Program of China (2021YFA1501500), National Natural Science Foundation of China (92061104, 21935010 and 21973096), Natural Science Foundation of Fujian Province (2021J06035) and Youth Innovation Promotion Association CAS (2021081).
Notes and references
- P. Feng, X. Bu and G. D. Stucky, Nature, 1997, 388, 735 CrossRef CAS.
- R. Banerjee, H. Furukawa, D. Britt, C. Knobler, M. O'Keeffe and O. M. Yaghi, J. Am. Chem. Soc., 2009, 131, 3875 CrossRef CAS PubMed.
- R. Simancas, D. Dari, N. Velamazán, M. T. Navarro, A. Cantín, J. L. Jordá, G. Sastre, A. Corma and F. Rey, Science, 2010, 330, 1219 CrossRef CAS PubMed.
- Z. Wang, J. Yu and R. Xu, Chem. Soc. Rev., 2012, 41, 1729 RSC.
-
J. Cejka, A. Corma and S. Zones, Zeolites and Catalysis. Synthesis, Reactions and Applications, Wiley, Weinheim, 2010 Search PubMed.
- C. Sun, Z. Liu, S. Wang, H. Pang, R. Bai, Q. Wang, W. Chen, A. Zheng, W. Yan and J. Yu, CCS Chem., 2021, 3, 189 CrossRef CAS.
- S.-T. Zheng, T. Wu, F. Zuo, C. Chou, P. Feng and X. Bu, J. Am. Chem. Soc., 2012, 134, 1934 CrossRef CAS PubMed.
- E. E. Knyazeva and I. I. Ivanova, Pet. Chem., 2019, 59, 262 CrossRef CAS.
- X. Luo, Y. Cao, T. Wang, G. Li, J. Li, Y. Yang, Z. Xu, J. Zhang, Q. Huo, Y. Liu and M. Eddaoudi, J. Am. Chem. Soc., 2016, 138, 786 CrossRef CAS PubMed.
- H. Wang, L. Han, D. Zheng, M. Yang, Y. H. Andaloussi, P. Cheng, Z. Zhang, S. Ma, M. J. Zaworotko, Y. Feng and Y. Chen, Angew. Chem., Int. Ed., 2020, 59, 6263 CrossRef CAS PubMed.
- K. Williams, L. Meng, S. Lee, L. Lux, W. Gao and S. Ma, Inorg. Chem. Front., 2016, 3, 393 RSC.
- S.-Y. Zhang, Z.-Y. Wang, J. Gao, K. Wang, E. Gianolio, S. Aime, W. Shi, Z. Zhou, P. Cheng and M. J. Zaworotko, Chem, 2019, 5, 1609 CAS.
- X.-C. Huang, Y.-Y. Lin, J.-P. Zhang and X.-M. Chen, Angew. Chem., Int. Ed., 2006, 45, 1557 CrossRef CAS PubMed.
- F. Wang, Z.-S. Liu, H. Yang, Y.-X. Tan and J. Zhang, Angew. Chem., Int. Ed., 2011, 50, 450 CrossRef CAS PubMed.
- K. S. Park, Z. Ni, A. P. Côté, J. Y. Choi, R. Huang, F. J. Uribe-Romo, H. K. Chae, M. O'Keeffe and O. M. Yaghi, Proc. Natl. Acad. Sci. U.S.A., 2006, 103, 10186 CrossRef CAS PubMed.
- N. T. T. Nguyen, H. Furukawa, F. Gándara, H. T. Nguyen, K. E. Cordova and O. M. Yaghi, Angew. Chem., Int. Ed., 2014, 53, 10645 CrossRef CAS PubMed.
- J. Zhang, T. Wu, C. Zhou, S. M. Chen, P. Y. Feng and X. H. Bu, Angew. Chem., Int. Ed., 2009, 48, 2542 CrossRef CAS PubMed.
- H.-X. Zhang, F. Wang, H. Yang, Y.-X. Tan, J. Zhang and X. Bu, J. Am. Chem. Soc., 2011, 133, 11884 CrossRef CAS PubMed.
- Y. Kang, F. Wang, J. Zhang and X. Bu, J. Am. Chem. Soc., 2012, 134, 17881 CrossRef CAS PubMed.
- H. Li, A. Laine, M. O'Keeffe and O. M. Yaghi, Science, 1999, 283, 1145 CrossRef CAS PubMed.
- P. Feng, X. Bu and N. Zheng, Acc. Chem. Res., 2005, 38, 293 CrossRef CAS PubMed.
- J. Zhang, X. Bu, P. Feng and T. Wu, Acc. Chem. Res., 2020, 53, 2261 CrossRef CAS PubMed.
- N. F. Zheng, X. H. Bu and P. Y. Feng, Nature, 2003, 426, 428 CrossRef CAS PubMed.
- K. S. Lokare, N. Frank, B. Braun-Cula, I. Goikoetxea, J. Sauer and C. Limberg, Angew. Chem., Int. Ed., 2016, 55, 12325 CrossRef CAS PubMed.
- B. Nohra, H. El Moll, L. M. Rodriguez Albelo, P. Mialane, J. Marrot, C. Mellot-Draznieks, M. O'Keeffe, R. N. Biboum, J. Lemaire, B. Keita, L. Nadjo and A. Dolbecq, J. Am. Chem. Soc., 2011, 133, 13363 CrossRef CAS PubMed.
- L. M. Rodriguez-Albelo, A. R. Ruiz-Salvador, A. Sampieri, D. W. Lewis, A. Gómez, B. Nohra, P. Mialane, J. Marrot, F. Sécheresse, C. Mellot-Draznieks, R. Ngo Biboum, B. Keita, L. Nadjo and A. Dolbecq, J. Am. Chem. Soc., 2009, 131, 16078 CrossRef PubMed.
- G. Verma, S. Butikofer, S. Kumar and S. Ma, Top. Curr. Chem., 2020, 378, 4 CrossRef CAS PubMed.
- B. Hou, C. Qin, C. Sun, X. Wang and Z. Su, CCS Chem., 2021, 3, 287 CrossRef CAS.
- L.-D. Lin, D. Zhao, X.-X. Li and S.-T. Zheng, Chem.–Eur. J., 2019, 25, 442 CrossRef CAS PubMed.
- D. Braga, L. Maini, P. P. Mazzeo and B. Ventura, Chem.–Eur. J., 2010, 16, 1553 CrossRef CAS PubMed.
- L. Chong, H. G. Mao, Y. Q. Sun and J. L. Song, Inorg. Chem., 2004, 43, 1964 CrossRef PubMed.
- J. P. Lang, Q. F. Xu, R. X. Yuan and B. F. Abrahams, Angew. Chem., Int. Ed., 2004, 43, 4741 CrossRef CAS PubMed.
- L.-D. Lin, C.-C. Deng, D. Zhao, X.-X. Li and S.-T. Zheng, Chem.–Eur. J., 2018, 24, 251 CrossRef CAS PubMed.
- Y. Xie, D. Kocaefe, Y. Kocaefe, J. Cheng and W. Liu, Nanoscale Res. Lett., 2016, 11, 259 CrossRef PubMed.
- R. G. Pearson, J. Am. Chem. Soc., 1963, 85, 3533 CrossRef CAS.
- G. Paglia, C. E. Buckley, A. L. Rohl, B. A. Hunter, R. D. Hart, J. V. Hanna and L. T. Byrne, Phys. Rev. B, 2003, 68, 144110 CrossRef.
- B. C. Lippens and J. H. De Boer, Acta Crystallogr., 1964, 17, 1312 CrossRef CAS.
-
W. W. Wendlandt and H. G. Hecht, Reflectance Spectroscopy, Interscience, New York, 1966 Search PubMed.
-
G. M. Sheldrick, SHELXL-2014 Program for Crystal Structure Solution and Refinement, Univerity of Göttingen, Göttingen, Germany, 2014 Search PubMed.
- G. D. Piero, M. Cesari, G. Dozzi and A. Mazzei, J. Organomet. Chem., 1977, 129, 281 CrossRef.
- C. Schnitter, A. Klemp, H. W. Roesky, H.-G. Schmidt, C. Röpken, R. Herbst-Irmer and M. Noltemeyer, Eur. J. Inorg. Chem., 1998, 2033 CrossRef CAS.
- C. Jeff Harlan, E. G. Gillan, S. G. Bott and A. R. Barron, Organometallics, 1996, 15, 5479 CrossRef.
- A. C. Stelzer, P. Hrobárik, T. Braun, M. Kaupp and B. Braun-Cula, Inorg. Chem., 2016, 55, 4915 CrossRef CAS PubMed.
- S. Abeysinghe, K. W. Corum, D. L. Neff, S. E. Mason and T. Z. Forbes, Langmuir, 2013, 29, 14124 CrossRef CAS PubMed.
- S. Abeysinghe, D. K. Unruh and T. Z. Forbes, Inorg. Chem., 2013, 52, 5991 CrossRef CAS PubMed.
- K. W. Corum, M. Fairley, D. K. Unruh, M. K. Payne, T. Z. Forbes and S. E. Mason, Inorg. Chem., 2015, 54, 8367 CrossRef CAS PubMed.
- C. M. Hong, R. G. Bergman, K. N. Raymond and F. D. Toste, Acc. Chem. Res., 2018, 51, 2447 CrossRef CAS PubMed.
- D. Zhang, T. K. Ronson, S. Güryel, J. D. Thoburn, D. J. Wales and J. R. Nitschke, J. Am. Chem. Soc., 2019, 141, 14534 CrossRef CAS PubMed.
- Y.-P. He, L.-B. Yuan, G.-H. Chen, Q.-P. Lin, F. Wang, L. Zhang and J. Zhang, J. Am. Chem. Soc., 2017, 139, 16845 CrossRef CAS PubMed.
- T. Yamada and H. Kitagawa, J. Am. Chem. Soc., 2009, 131, 6312 CrossRef CAS PubMed.
- J. Zhang, L. Wojtas, R. W. Larsen, M. Eddaoudi and M. J. Zaworotko, J. Am. Chem. Soc., 2009, 131, 17040 CrossRef CAS PubMed.
- Q. Lin, X. Bu, C. Mao, X. Zhao, K. Sasan and P. Feng, J. Am. Chem. Soc., 2015, 137, 6184 CrossRef CAS PubMed.
- A. L. Spek, J. Appl. Crystallogr., 2003, 36, 7 CrossRef CAS.
- M. Kruk and M. Jaroniec, Chem. Mater., 2001, 13, 3169 CrossRef CAS.
- S. Yang, X. Song, P. Zhang and L. Gao, ACS Appl. Mater. Interfaces, 2015, 7, 75 CrossRef CAS PubMed.
- X. Fan, F. Yuan, D. Li, S. Chen, Z. Cheng, Z. Zhang, S. Xiang, S.-Q. Zang, J. Zhang and L. Zhang, Angew. Chem., Int. Ed., 2021, 60, 12949 CrossRef CAS PubMed.
- H. Wang, J. Getzschmann, I. Senkovska and S. Kaskel, Microporous Mesoporous Mater., 2008, 116, 653 CrossRef CAS.
- M. Andrzejewski and A. Katrusiak, J. Phys. Chem. Lett., 2017, 8, 279 CrossRef CAS PubMed.
- X. Yang, P. K. Majhi, H. Chai, B. Liu, J. Sun, T. Liu, Y. Liu, L. Zhou, J. Xu, J. Liu, D. Wang, Y. Zhao, Z. Jin and Y. R. Chi, Angew. Chem., Int. Ed., 2021, 60, 159 CrossRef CAS PubMed.
- S. Rojas-Buzo, P. García-García and A. Corma, Green Chem., 2018, 20, 3081 RSC.
Footnote |
† Electronic supplementary information (ESI) available: Experimental section, detailed structure charts, stability analysis, sorption isotherms, characterization, catalytic mechanism, and tables. The structures reported in this article have been deposited at the Cambridge Crystallographic Data Centre (CCDC). CCDC 2056099–2056118, 2056127, 2156657 (AlOC-99–AlOC-120). For ESI and crystallographic data in CIF or other electronic format see https://doi.org/10.1039/d2sc00526c |
|
This journal is © The Royal Society of Chemistry 2022 |